Influenza and Influenza Vaccine: A Review
Affiliations.
- 1 Nurse-Midwifery and Women's Health Nurse Practitioner Programs, University of Cincinnati, Cincinnati, Ohio.
- 2 Department of Obstetrics and Gynecology, University of Cincinnati, Cincinnati, Ohio.
- 3 Family Nurse Practitioner Program, University of Cincinnati, Cincinnati, Ohio.
- PMID: 33522695
- PMCID: PMC8014756
- DOI: 10.1111/jmwh.13203
Influenza is a highly contagious, deadly virus, killing nearly half a million people yearly worldwide. The classic symptoms of influenza are fever, fatigue, cough, and body aches. In the outpatient setting, diagnosis can be made by clinical presentation with optional confirmatory diagnostic testing. Antiviral medications should be initiated as soon as possible, preferably within 24 hours of initiation of symptoms. The primary preventive measure against influenza is vaccination, which is recommended for all people 6 months of age or older, including pregnant and postpartum women, unless the individual has a contraindication. Vaccination should occur at the beginning of flu season, which typically begins in October. It takes approximately 14 days after vaccination for a healthy adult to reach peak antibody protection. There are challenges associated with vaccine composition and vaccine uptake. It takes approximately 6 to 8 months to identify and predict which influenza strains to include in the upcoming season's vaccine. During this time, the influenza virus may undergo antigenic drift, that is, mutating to avoid a host immune response. Antigenic drift makes the vaccine less effective in some seasons. The influenza virus occasionally undergoes antigenic shift, in which it changes to a novel virus, creating potential for a pandemic. There are also barriers to vaccine uptake, including lack of or limited access to care and misconceptions about receiving the vaccine. Interventions that improve access to and uptake of the influenza vaccine must be initiated, targeting multiple levels, including health care policy, patients, health care systems, and the health care team. This article reviews information about influenza identification, management, and prevention.
Keywords: diagnostic tests; influenza; pregnancy; screening; vaccination.
© 2021 by the American College of Nurse-Midwives.
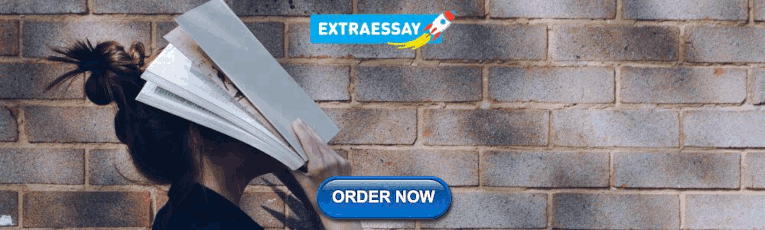
Publication types
- Antiviral Agents / therapeutic use*
- Epidemics / prevention & control
- Genetic Drift
- Influenza A virus / genetics
- Influenza A virus / immunology
- Influenza Vaccines / therapeutic use*
- Influenza, Human / diagnosis
- Influenza, Human / epidemiology
- Influenza, Human / prevention & control*
- Influenza, Human / therapy*
- Reverse Transcriptase Polymerase Chain Reaction
- Vaccination
- Antiviral Agents
- Influenza Vaccines
- U.S. Department of Health & Human Services

- Virtual Tour
- Staff Directory
- En Español
You are here
News releases.
Media Advisory
Monday, April 8, 2019
Scientists review influenza vaccine research progress and opportunities

In a new series of articles, experts in immunology, virology, epidemiology, and vaccine development detail efforts to improve seasonal influenza vaccines and ultimately develop a universal influenza vaccine. The 15 articles are part of a supplement in the April 15 issue of the Journal of Infectious Diseases . Researchers from the National Institute of Allergy and Infectious Diseases (NIAID), part of the National Institutes of Health, and scientists supported by NIAID, are among the contributing authors. Barney S. Graham, M.D., Ph.D., deputy director of NIAID’s Vaccine Research Center (VRC), and Michelle C. Crank, M.D., head of the Translational Sciences Core in the VRC’s Viral Pathogenesis Laboratory, edited the supplement.
In an introductory article, NIAID Director Anthony S. Fauci, M.D. and Catharine I. Paules, M.D., an infectious disease physician at Penn State Health Milton S. Hershey Medical Center, underscore the public health need for improved influenza vaccines, noting the approximately 291,000 to nearly 646,000 deaths worldwide each year due to seasonal influenza. They also discuss the possibility of another influenza pandemic, which occurs when a novel influenza virus to which most people do not have immunity arises unpredictably. The 1918 influenza pandemic caused an estimated 50 million to 100 million deaths.
The current seasonal influenza vaccine reduces influenza-related hospitalizations and deaths. However, people must get vaccinated annually due to constantly changing influenza viruses, and in some years, the vaccine confers less-than-optimal protection against infection. Drs. Fauci and Paules note that recent scientific advances, combined with scientists’ efforts to coordinate and accelerate their research activities, have provided unprecedented momentum toward developing a so-called “ universal” influenza vaccine . Such a vaccine would offer long-term protection against multiple seasonal and pandemic influenza viruses.
The supplement articles detail ongoing research and what remains to be learned about influenza—such as how the human immune system responds to influenza infection and vaccination. Experts also discuss how such research might influence vaccine design approaches and help the public health community better prepare for the next influenza pandemic.
In closing remarks, Drs. Crank and Graham, along with John R. Mascola, M.D., VRC director, note, “Vaccinology is experiencing a revolution thanks to scientific and technological breakthroughs of the past decade, and hopefully we can find the resolve, political will, and new business plans to take full advantage of these new opportunities and prepare ourselves before the next pandemic arrives.”
Articles in the supplement from NIAID experts include:
CI Paules and AS Fauci. Influenza vaccines: good, but we can do better. Journal of Infectious Diseases DOI: 10.1093/infdis/jiy633 (2019)
CM Saad-Roy et al . Dynamic perspectives on the search for a universal influenza vaccine. Journal of Infectious Diseases DOI: 10.1093/infdis/jiz044 (2019)
DM Morens and JK Taubenberger. Making universal influenza vaccines: lessons from the 1918 pandemic. Journal of Infectious Diseases DOI: 10.1093/infdis/jiy728 (2019)
MC Crank et al . Preparing for the next influenza pandemic: the development of a universal influenza vaccine. Journal of Infectious Diseases DOI: 10.1093/infdis/jiz043 (2019)
M Kanekiyo et al . New vaccine design and delivery technologies. Journal of Infectious Diseases DOI: 10.1093/infdis/jiy745 (2019)
NIAID Director Anthony S. Fauci, M.D., is available for comment.
To schedule interviews, please contact Jennifer Routh, (301) 402-1663, [email protected] .
NIAID conducts and supports research — at NIH, throughout the United States, and worldwide — to study the causes of infectious and immune-mediated diseases, and to develop better means of preventing, diagnosing and treating these illnesses. News releases, fact sheets and other NIAID-related materials are available on the NIAID website .
About the National Institutes of Health (NIH): NIH, the nation's medical research agency, includes 27 Institutes and Centers and is a component of the U.S. Department of Health and Human Services. NIH is the primary federal agency conducting and supporting basic, clinical, and translational medical research, and is investigating the causes, treatments, and cures for both common and rare diseases. For more information about NIH and its programs, visit www.nih.gov .
NIH…Turning Discovery Into Health ®
Connect with Us
- More Social Media from NIH
Log in using your username and password
- Search More Search for this keyword Advanced search
- Latest content
- Current issue
- BMJ Journals More You are viewing from: Google Indexer
You are here
- Online First
- Trivalent and quadrivalent seasonal influenza vaccine in adults aged 60 and older: a systematic review and network meta-analysis
- Article Text
- Article info
- Citation Tools
- Rapid Responses
- Article metrics

- http://orcid.org/0000-0001-6388-4825 Areti Angeliki Veroniki 1 , 2 ,
- Sai Surabi Thirugnanasampanthar 2 ,
- Menelaos Konstantinidis 1 , 2 ,
- Jasmeen Dourka 2 ,
- Marco Ghassemi 2 ,
- Dipika Neupane 2 ,
- Paul Khan 2 ,
- Vera Nincic 2 ,
- Margarita Corry 3 ,
- Reid Robson 2 ,
- Amanda Parker 2 ,
- Charlene Soobiah 1 , 2 ,
- Angela Sinilaite 4 ,
- http://orcid.org/0000-0002-8738-0055 Pamela Doyon-Plourde 4 ,
- Anabel Gil 4 ,
- Winnie Siu 4 ,
- Nasheed Moqueet 4 ,
- http://orcid.org/0000-0002-6257-4806 Adrienne Stevens 4 ,
- Kelly English 5 ,
- http://orcid.org/0000-0002-0751-8932 Ivan D Florez 6 , 7 , 8 ,
- Juan J Yepes-Nuñez 9 , 10 ,
- http://orcid.org/0000-0001-5662-8647 Brian Hutton 11 ,
- Matthew Muller 1 , 12 , 13 ,
- Lorenzo Moja 14 ,
- Sharon Straus 1 , 2 , 15 ,
- Andrea C Tricco 2 , 16
- 1 Institute of Health Policy Management and Evaluation , University of Toronto , Toronto , Ontario , Canada
- 2 Knowledge Translation Program , Li Ka Shing Knowledge Institute, St. Michael’s Hospital, Unity Health Toronto , Toronto , Ontario , Canada
- 3 Trinity College Dublin School of Nursing and Midwifery , Dublin , Ireland
- 4 Public Health Agency , Ottawa , Ontario , Canada
- 5 Patient Partner, Strategy for Patient Oriented-Research Evidence Alliance (SPOR EA) , St Michael's Hospital , Toronto , Ontario , Canada
- 6 Department of Pediatrics , University of Antioquia Faculty of Medicine , Medellin , Colombia
- 7 School of Rehabilitation Science , McMaster University , Hamilton , Ontario , Canada
- 8 Pediatric Intensive Care Unit , Clinica Las Américas-AUNA , Medellin , Colombia
- 9 University of los Andes Faculty of Medicine , Bogota , Cundinamarca , Colombia
- 10 Pulmonology Service, Internal Medicine Section , University Hospital of the Fundacion Santa Fe de Bogota , Bogota , Colombia
- 11 Ottawa Hospital Research Institute , University of Ottawa , Ottawa , Ontario , Canada
- 12 Department of Medicine , St Michael's Hospital, Unity Health Toronto , Toronto , Ontario , Canada
- 13 Institute of Medical Sciences , University of Toronto , Toronto , Ontario , Canada
- 14 Department of Biomedical Sciences and Technologies , University of Milan , Milano , Lombardia , Italy
- 15 Department of Geriatric Medicine , University of Toronto , Toronto , Ontario , Canada
- 16 Epidemiology Division & Institute of Health Policy, Management, and Evaluation , University of Toronto Dalla Lana School of Public Health , Toronto , Ontario , Canada
- Correspondence to Dr Areti Angeliki Veroniki, Knowledge Translation Program, Li Ka Shing Knowledge Institute, St Michael's Hospital, Unity Health Toronto, Toronto, ON, Canada; argie.veroniki{at}gmail.com
Objectives To compare the efficacy of influenza vaccines of any valency for adults 60 years and older.
Design and setting Systematic review with network meta-analysis (NMA) of randomised controlled trials (RCTs). MEDLINE, EMBASE, JBI Evidence-Based Practice (EBP) Database, PsycINFO, and Cochrane Evidence -Based Medicine database were searched from inception to 20 June 20, 2022. Two reviewers screened, abstracted, and appraised articles (Cochrane Risk of Bias (ROB) 2.0 tool) independently. We assessed certainty of findings using Confidence in Network Meta-Analysis and Grading of Recommendations, Assessment, Development and Evaluations approaches. We performed random-effects meta-analysis and network meta-analysis (NMA), and estimated odds ratios (ORs) for dichotomous outcomes and incidence rate ratios (IRRs) for count outcomes along with their corresponding 95% confidence intervals (CIs) and prediction intervals.
Participants Older adults (≥60 years old) receiving an influenza vaccine licensed in Canada or the USA (vs placebo, no vaccine, or any other licensed vaccine), at any dose.
Main outcome measures Laboratory-confirmed influenza (LCI) and influenza-like illness (ILI). Secondary outcomes were the number of vascular adverse events, hospitalisation for acute respiratory infection (ARI) and ILI, inpatient hospitalisation, emergency room (ER) visit for ILI, outpatient visit, and mortality, among others.
Results We included 41 RCTs and 15 companion reports comprising 8 vaccine types and 206 032 participants. Vaccines may prevent LCI compared with placebo, with high-dose trivalent inactivated influenza vaccine (IIV3-HD) (NMA: 9 RCTs, 52 202 participants, OR 0.23, 95% confidence interval (CI) (0.11 to 0.51), low certainty of evidence) and recombinant influenza vaccine (RIV) (OR 0.25, 95%CI (0.08 to 0.73), low certainty of evidence) among the most efficacious vaccines. Standard dose trivalent IIV3 (IIV3-SD) may prevent ILI compared with placebo, but the result was imprecise (meta-analysis: 2 RCTs, 854 participants, OR 0.39, 95%CI (0.15 to 1.02), low certainty of evidence). Any HD was associated with prevention of ILI compared with placebo (NMA: 9 RCTs, 65 658 participants, OR 0.38, 95%CI (0.15 to 0.93)). Adjuvanted quadrivalent IIV (IIV4-Adj) may be associated with the least vascular adverse events, but the results were very uncertain (NMA: eight 8 RCTs, 57 677 participants, IRR 0.18, 95%CI (0.07 to 0.43), very low certainty of evidence). RIV on all-cause mortality may be comparable to placebo (NMA: 20 RCTs, 140 577 participants, OR 1.01, 95%CI (0.23 to 4.49), low certainty of evidence).
Conclusions This systematic review demonstrated efficacy associated with IIV3-HD and RIV vaccines in protecting older persons against LCI. RIV vaccine may reduce all-cause mortality when compared with other vaccines, but the evidence is uncertain. Differences in efficacy between influenza vaccines remain uncertain with very low to moderate certainty of evidence.
PROSPERO registration number CRD42020177357.
- immunization
Data availability statement
Data are available on reasonable request. The full dataset and statistical code are available at https://osf.io/eqb9h/ .
This is an open access article distributed in accordance with the Creative Commons Attribution Non Commercial (CC BY-NC 4.0) license, which permits others to distribute, remix, adapt, build upon this work non-commercially, and license their derivative works on different terms, provided the original work is properly cited, appropriate credit is given, any changes made indicated, and the use is non-commercial. See: http://creativecommons.org/licenses/by-nc/4.0/ .
https://doi.org/10.1136/bmjebm-2023-112767
Statistics from Altmetric.com
Request permissions.
If you wish to reuse any or all of this article please use the link below which will take you to the Copyright Clearance Center’s RightsLink service. You will be able to get a quick price and instant permission to reuse the content in many different ways.
WHAT IS ALREADY KNOWN ON THIS TOPIC
Seasonal influenza vaccination of older adults (≥60 years old) is an important societal, cost-effective means of reducing morbidity and mortality.
A multitude of licensed seasonal influenza vaccines for older adults are available in a variety of formulations (such as IIV3, IIV4; prepared in standard and high doses; with and without an adjuvant) relying on production methods including those based on embryonated chicken eggs, or mammalian cell cultures and comprising seasonally selected viral strains or recombinant constructs.
Lack of high-quality analysis of randomised controlled trial (RCT) data pertaining to influenza vaccine production and composition poses challenges for public health clinicians and policy-makers who are tasked with making evidence-based decisions regarding recommendations about choosing optimally efficacious and safe influenza vaccines for older adults.
WHAT THIS STUDY ADDS
This systematic review and network meta-analysis of RCT data found that recombinant influenza vaccines were among the most effective (lowest odds of laboratory-confirmed influenza) and safest (lowest odds of all-cause mortality) of any licensed influenza vaccine type administered to older adults.
HOW THIS STUDY MIGHT AFFECT RESEARCH, PRACTICE OR POLICY
Our review points to a potential safety concern regarding increased odds of all-cause mortality associated with older adults receiving adjuvanted influenza vaccines (IIV3-adj and IIV4-adj).
Introduction
Seasonal influenza is a leading cause of infectious respiratory disease globally causing 3–5 million cases of severe illness and 650 000 deaths annually. 1 In Canada, influenza ranks among the top 10 causes of mortality, with approximately 3500 deaths and 12 200 influenza hospitalisations per year. 2 Annually, influenza results in an economic burden on average of US$14 000–US$20 000 per laboratory-confirmed influenza (LCI) hospitalisation. 3
Older adults, particularly those who are frail bare a disproportionate burden of influenza and its complications. Globally, individuals 75 years of age and older experience the highest rates of influenza-associated respiratory deaths (51.3–99.4 deaths per 100 000 vs 13.3–27.8 in those 60–74 years of age and 1.0–5.1 in those less than 60 years of age). 4 Annual influenza vaccination is the best way to prevent influenza infection and its complications. 5 6
Although there are various influenza vaccines available for older adults, all current vaccines contain two influenza A virus strains and either one (trivalent) or two (quadrivalent) influenza B virus strains. 7 Existing randomised controlled trials (RCTs) focus on the safety and efficacy of individual vaccines. The lack of direct comparative vaccine efficacy (VE) evidence poses challenges to public health clinicians and policy-makers to make evidence-based decisions regarding the preferential use of one influenza vaccine over another in older adults regarding vaccine choice. We aimed to systematically assess the comparative efficacy of influenza vaccines (eg, trivalent and quadrivalent at standard-dose (SD), high-dose (HD) or adjuvanted (Adj)) in adults 60 years of age and older. A systematic review with network meta-analysis (NMA) was conducted to fill this critical knowledge gap in comparative VE.
The protocol was registered in PROSPERO (CRD42020177357), and any deviations are reported in online supplemental appendix 1 (additional file 1) . In Canada, national immunisation recommendations of vaccine use are made by the National Advisory Committee on Immunisation (NACI). Our protocol was revised in collaboration with the NACI Influenza Working Group, to improve the feasibility and relevancy of this review for key knowledge users. Below, we briefly describe the methods used in our review; more details are presented in online supplemental appendix 2 (additional file 1) . This systematic review follows PRISMA (Preferred Reporting Items for Systematic Reviews and Meta-analyses) extension to NMA 8 and Guidance for Reporting Involvement of Patients and the Public (GRIPP-2) reporting guidelines 9 ( online supplemental additional files 2 and 3 ), as well as the methods outline in the Cochrane Handbook for Systematic Reviews of Interventions. 10
Supplemental material
Knowledge user engagement.
We enhanced systematic review process by employing an integrated knowledge translation approach 11 from project onset via established partnerships with the NACI Influenza Working Group, which will use these research results to inform the development of guidance for influenza vaccination. Our systematic review included two clinicians (MM and SS), one patient partner (KE), the NACI Secretariat (ASinilaite, PD-P, AG, WS, NM and AStevens) and seven researchers (AAV, MK, IDF, JJY-N, BH, LM and ACT). Throughout the research process, all knowledge users actively participated by contributing their insights in various stages, such as developing and refining the research question, reviewing the search parameters, framing the risk groups, prioritising the outcome measures and interpreting the study findings.
Data sources and searches
We searched Ovid MEDLINE, JBI (formerly the Joanna Briggs Institute) Evidence-Based Practice Database, Embase, PsycINFO and Cochrane Evidence-Based Medicine database from inception to 31 March 2022, with an updated search on 20 June 2022. An expert librarian developed the search strategies with input from the research team. Another expert librarian peer-reviewed the search strategy using the Peer Review of Electronic Search Strategies checklist (see online supplemental appendix 3 (additional file 1) for literature search). 12 Grey literature (ie, unpublished or difficult-to-locate studies) was searched using the Canadian Agency for Drugs and Technologies in Health guide (see online supplemental appendix 4 (additional file 1) ). 13 We consulted content experts from the NACI Influenza Working Group, and scanned the NACI Practices review, 14 the National Immunisation Technical Advisory Group websites (eg, SAGE, JCVI, ATAGI, STIKO ( https://www.nitag-resource.org ), reference lists of included studies and related reviews searching for additional relevant studies ( online supplemental appendix 4 (additional file 1) ). A library technician searched for article corrections and retractions to inform our retrieval of full-text reports.
Eligibility criteria
Below, we formulate the Population, Intervention, Comparator, Outcome, Study design criteria for our review.
We included RCTs evaluating adults 60 years of age and older. Studies involving the general population that reported stratified data and results for older adults were also included. If stratified data and results were not reported, authors were contacted to retrieve any additional information with up to three reminders. RCTs with wider population characteristics reporting on relevant outcomes of interest in subsets of participants, and including a subcategory of age 60 years and older were also eligible.
Intervention
RCTs comparing any influenza vaccine at any dose that was licensed in Canada or the USA, at time of the review and irrespective of commercial name. Eligible influenza vaccines included but were not limited to egg based, quadrivalent inactivated influenza vaccine (IIV4) at SD or HD or Adj, trivalent IIV (IIV3) at SD or HD or Adj, mammalian cell culture-based trivalent or quadrivalent IIV, and quadrivalent or trivalent recombinant influenza vaccine (RIV4 or RIV3). We determined vaccine licence at time of the study based on the details provided by the studies and/or trial protocols. Intramuscular and subcutaneous routes of administration of relevant licensed vaccines were considered for inclusion.
We excluded intradermal, intranasal and AS03-adjuvanted vaccines, which are not licensed for use in Canada or the USA. Pandemic vaccines were excluded along with experimental vaccines that have not been approved for use.
Another influenza vaccine, placebo or any other licensed vaccine was eligible.
Eligible outcomes were determined in collaboration with the NACI Influenza Working Group and clinician experts based on the GRADE (Grading of Recommendations, Assessment, Development and Evaluations) 15 methodology for rating the importance of outcomes for decision-making. 16 The primary outcomes were LCI and influenza-like illness (ILI). Secondary outcomes were the number of vascular adverse events, hospitalisation for acute respiratory infection (ARI) and ILI, inpatient hospitalisation, emergency room (ER) visit for ILI, outpatient visit and mortality. All outcomes included binary data apart from vascular adverse events that included count data. Other outcomes of interest included ARI cases, LCI hospitalisation, LC-ARI cases, ER visit for ARI or LCI or pneumonia or any cause, hospitalisation for pneumonia due to ILI, ARI, LCI, inpatient or outpatient hospital visit, hospitalisation due to cardiovascular events, LCI-related mortality, LCI-related healthcare interactions, number of hospitalisations due to cardiovascular events, number of participants with vascular adverse events and influenza-related vascular adverse events.
Study design
We included RCTs regardless of publication status, publication date, duration of follow-up, language of publication, geographical region or setting (eg, community, residential facilities, nursing homes).
Selection process and data extraction
The study selection, data extraction and certainty of evidence processes are described in online supplemental appendix 2 .
We included both RCTs and cluster RCTs in the review. Following the Cochrane recommendations, 17 for cluster RCTs, we initially planned to abstract the effective sample size and/or intraclass correlation. However, since this was not available in the included studies, 18–20 we used the adjusted effect estimates considering the cluster design as reported in the publications. Arm-level data were abstracted from multiarm RCTs as reported in the publications, and analysed in an NMA accounting for correlation between intervention arms that share a common comparator.
Within and across study bias assessment
Once a pilot-test was carried out for risk of bias (ROB) assessment on a random sample of five studies, two reviewers (JD, PK, VN and RR) independently and in duplicate appraised the included RCTs using the Cochrane ROB 2.0 tool. 21 Conflicts were resolved by a third reviewer (MK). The ROB was rated as low, high or some concerns in each of the following domains:
Randomisation process.
Deviations from the intended interventions.
Missing outcome data.
Measurement of the outcome selection of the reported results.
Timing of identification or recruitment of participants (cluster RCTs only, as per ROB 2.0 extension to cluster RCTs).
We used relevant signalling questions within each domain, as suggested by ROB 2.0 tool, and responded as yes, probably yes, probably no, no or no information, which helped reach overall ROB judgements ( https://www.riskofbias.info/ ). 22 ROB was assessed for each outcome included in a meta-analysis separately (completed for primary and secondary outcomes on 7 April 2023 and 15 May 2023, respectively), and based on the reported study information (following the principles of intention to treat, where available).
We visually inspected small-study effects and reporting bias using the comparison-adjusted funnel plot when at least ten studies were available per outcome. 23 For this analysis, the interventions were ordered chronologically 24 according to when they were licensed in Canada (or USA where applicable).
Study-level information on participant and study characteristics was synthesised descriptively.
Pairwise meta-analyses
We synthesised data in a meta-analysis using the OR for dichotomous outcomes and the incidence rate ratio (IRR) for count outcomes, along with corresponding 95% CIs when at least two studies were available. We performed a random-effects inverse-variance meta-analysis since we expected the studies to be methodologically and clinically different (eg, vaccine dose, participant age). We estimated the overall effect size and its 95% CI using the Hartung-Knapp-Sidik-Jonkman (HKSJ) method for meta-analyses with few studies 25–27 : specifically, when the estimated heterogeneity was positive (>0) among at least three studies in the meta-analysis, we used the HKSJ approach, 28 29 otherwise we applied the Wald-type approach. 25–27 30–32 We supplemented estimated overall effects and 95% CIs with prediction intervals (PIs) to capture the interval within which we expected the true intervention effect of a new study to fall. We used the restricted maximum likelihood method 33 to estimate the between-study variance (τ 2 ) and the Q-profile approach to calculate its 95% CI. 34 35 We also calculated the percentage of variation that can be attributed to heterogeneity rather than chance using the I 2 statistic. 36
Network meta-analyses
For each outcome, we explored connectivity of evidence and network geometry graphically through network diagrams and summarising interventions, RCTs and intervention comparisons. Node formulation, including lumping or splitting of individual interventions, was decided based on knowledge user expertise, including the clinical, patient and policy-makers with the NACI Secretariat perspectives, and in accordance to our research question. We considered that different decisions on lumping or splitting interventions may impact the validity of our NMA results, and assessed relevant assumptions in all different network geometries. We conducted a frequentist random-effects NMA for each connected network diagram, when the number of studies exceeded the number of intervention nodes. Alternatively, pairwise meta-analysis was conducted for each intervention comparison separately. A common within-network τ 2 was assumed for each network, which was a clinically reasonable assumption since networks were composed of influenza-type vaccines; τ 2 was estimated using the restricted maximum likelihood method. 33 We estimated summary ORs or IRRs and presented them along with 95% CIs and PIs. Interventions were ranked according to their efficacy using the P-score statistic. 37
We conducted an NMA under the transitivity assumption, where trial participants are equally likely to be randomised to any of the network interventions and the included trials are sufficiently similar across intervention comparisons with respect to the distribution of effect modifiers. We visually assessed transitivity across intervention comparisons on the a priori selected effect modifiers: overall ROB, participant age, percentage of female participants and year of publication, using mean for continuous and mode for discrete variables. The statistical manifestation of intransitivity can cause inconsistency in the network of interventions. We assessed for consistency using both local (node-splitting) 38 and global (design-by-treatment interaction) 39 approaches. We planned to assess transitivity for populations with comorbid conditions, frailty and previous influenza vaccination, but these were rarely reported in the included studies.
Exploring the impact of effect modifiers
For the primary outcomes and outcomes displaying evidence of inconsistency, intransitivity or heterogeneity in any NMA, we performed subgroup and sensitivity analyses considering the predefined potential treatment effect modifiers and monitored changes in heterogeneity and effect estimates, whenever possible. In our primary outcomes, we also compared match and mismatch between the circulating and vaccine strains. To determine matched and mismatched studies, we followed previously published methods. 40 We categorised a study as matched when the influenza strains were antigenically similar to the vaccine stains. Similarly, we categorised a study as mismatched when the influenza strains were antigenically distinct from vaccine strains. When the information on matching was not reported in the study, we determined the matching using surveillance data ( online supplemental appendices 5–7 (additional file 1) ). We also combined intervention nodes for placebo and for trivalent and quadrivalent formulation by vaccine type (ie, adjuvant, SD, HD and RIV). At the request of the NACI Influenza Working Group, we excluded tetanus–diphtheria–pertussis vaccine (Tdap) in a sensitivity analysis, where Tdap was included as an intervention. We were unable to explore dose effects in a dose-response NMA due to network sparsity. 41 Similarly, we were unable to explore vaccine formulation, frequency, route of administration, platform, type and dose of adjuvant, and licensing product names due to network sparsity or due to incomplete reporting in individual studies which also increased network sparsity further.
Presentation of results
Summary ORs/IRRs for all pairwise comparisons, along with their 95% CIs and 95% PIs, were presented in tables. We also transformed ORs to risk ratios (RRs) and calculated the VE for all pairwise comparisons in the LCI and ILI outcomes using the combined mean baseline risk per outcome. P-scores across interventions and outcomes were presented in a rank-heat plot ( https://rankheatplot.com/rankheatplot/ ). 33 Statistical analyses were conducted using the meta 42 and netmeta 43 packages in R.
Assessment of the confidence in the evidence
We assessed confidence in NMA estimates for each primary NMA outcome, using Confidence in Network Meta-Analysis (ie, LCI, number of vascular adverse events, outpatient visits and all-cause mortality outcomes). 44 Also, we assessed confidence in pairwise meta-analysis estimates for which an NMA could not be performed using the GRADE approach (ie, in IIV3-HD vs IIV3-SD for ILI, hospitalisation due to ARI, ER visit due to ILI, hospitalisation due to ILI, inpatient hospitalisation, and in IIV3-SD vs placebo for ILI). 45 More details are presented in online supplemental appendix 2 .
Overall, 41 studies (206 032 participants) and 15 companion reports were included, 18–20 46–96 after screening 6858 citations and 3425 full-text articles ( figure 1 ). All of the included studies were written in English. Two 71 97 of the seven contacted authors 71 97–102 responded to our emails asking for additional information regarding study arms (eg, details about the vaccine used in the RCT) and outcomes (eg, outcome data stratified by age or study arm), and one 71 provided outcome data stratified by study arm for analysis. Of the 41 RCTs, 26 were included in the quantitative analysis; the remaining 15 RCTs did not report relevant data for the analysis (eg, study size but no event data were available) 20 52–54 61 64 66 68 70 73–75 77–79 or compared the same intervention node (eg, Agriflu vs Fluvirin 103 both of which are trivalent, inactivated, egg-based influenza vaccines) or were disconnected from the NMA and did not contribute to any pairwise meta-analyses ( online supplemental appendix 8 ). Lists of the studies included in the review and studies excluded after data abstraction can be found in online supplemental appendices 9 and 10 (additional file 1) , respectively.
- Download figure
- Open in new tab
- Download powerpoint
PRISMA flow diagram for identification of eligible studies. JBI, Joanna Briggs Institute; PRISMA, Preferred Reporting Items for Systematic Reviews and Meta-analyses,
Study, patient and intervention characteristics
The included RCTs were published between 1994 and 2021 with most (36%) published between 2016 and 2020 ( table 1 ). Most of the studies were conducted in the USA (N=19, 46%) and funded by industry (N=23, 56%). Three were cluster-RCTs. 18–20 Most RCTs (N=9, 22%) compared IIV3-SD vs IIV3-HD, followed by RCTs comparing IIV3-SD vs placebo or vs IIV3-Adj (N=4, 10%) ( table 1 , online supplemental appendix 11 (additional file 1) . The duration of follow-up and timing of assessment was reported in 24 studies and ranged between 3 months and 1 year.
The reported participant age within most RCTs (n=34, 83%) was ≥65 years; participants reflected racial diversity (n=19, 46%; table 2 , online supplemental appendix 12 (additional file 1) .
- View inline
Study characteristics of included studies (N=41)
Participant characteristics of included studies (N=41)
Overall, eight influenza vaccine types were identified across all RCT interventions: IIV3-Adj, IIV4-Adj, IIV3-HD, IIV4-HD, IIV3-SD, IIV4-SD, RIV3 and RIV4. Most influenza vaccines were egg-based (n=47, 46%), and at SD (n=64, 63%). The delay between doses, which, if reported and/or applicable, ranged from 3 to 4 weeks ( table 3 , online supplemental appendix 13 (additional file 1) ). In our analysis, and as recommended by our knowledge users, RIV3 was combined with RIV4 in a single node. Also, the individual intervention groups (IIV3-Adj, IIV4-Adj, IIV3-HD, IIV4-HD, IIV3-SD, IIV4-SD, RIV) assessed in our analysis did not consider multiple doses, modalities or types of adjuvants.
Vaccine characteristics of included studies (N=41)
Of the a priori selected outcomes, 17 were omitted from both pairwise or NMA. This is because in 10 of these outcomes, there was a lack of relevant data within the identified studies. In two outcomes only one study reported relevant data, while for five outcomes, there were multiple studies available, but one study was included per intervention comparison. Of the outcomes analysed in pairwise or NMA, an additional seven outcomes had studies which were not included in the analyses, because intervention comparisons included a single study or were disconnected from the underlying network. See online supplemental appendix 8 (additional file 1) for more details.
Within-study ROB and across-study reporting bias
Within-study bias appraisal suggested that overall low ROB was present for: 2 (22%) RCTs reporting LCI, 9 (45%) RCTs reporting all-cause mortality, 4 (50%) RCTs reporting vascular adverse events, 3 (33%) RCTs reporting ILI and 1 RCT (50%) reporting ER-visits ( figure 2 , online supplemental appendix 14 (additional file 1) ). Overall, ROB for outpatient visits, hospitalisation for ARI and ILI, and inpatient hospitalisations outcomes were judged to be at some or high concerns ( online supplemental appendix 15 (additional file 1) ).
Cochrane Risk of Bias 2 summary results of primary outcomes (N=26 RCTs). LCI (n=9). ILI (n=9). RCTs, randomised controlled trials.
Reporting bias across studies could be assessed only for the all-cause mortality outcome, due to the small number of studies per outcome. The comparison-adjusted funnel indicated no evidence of publication bias or small-study effects ( online supplemental appendix 16 (additional file 1) ).
Results of testing assumptions
Assessment of the consistency assumption was possible for LCI and all-cause mortality outcomes (with available closed loops of evidence), where there was no evidence of statistical inconsistency (design-by-treatment interaction model LCI: χ 2 =2.35, 2 df, p=0.31; all-cause mortality: χ 2 =0.23, 3 df, p=0.98; online supplemental appendix 17A (additional file 1) ). Overall, there were no important concerns regarding average age or ROB, but the mean percentage of female participants varied for LCI (range across comparisons: 32%–59%), outpatient visits (range across comparisons: 26%–55%) and all-cause mortality (range across comparisons: 26%–63%) outcomes, which may challenge the reliability of the transitivity assumption ( online supplemental appendix 17B (additional file 1) ).
Results of syntheses
Below, we present the analysis results for NMA and pairwise meta-analysis for the included vaccines against placebo or against IIV3-SD if a placebo node was not available for the outcome. Additional results and analyses are presented in online supplemental appendix 18 (additional file 1) . The network geometry for each outcome analysed in an NMA is presented in figure 3 .
Network-plots of (A) LCI, (B) number of vascular AEs, (C) outpatient visits, (D) all-cause mortality. Adj, adjuvanted; AEs, adverse events; HD, high dosage; IIV3, inactivated influenza vaccine trivalent; IIV4, IIV quadrivalent; LCI, laboratory-confirmed influenza; RIV, recombinant influenza vaccine; SD, standard dosage; Tdap, tetanus–diphtheria–pertussis.
Laboratory-confirmed influenza
The NMA for LCI included 9 RCTs, 48 55–57 62 63 69 76 96 6 interventions (IIV3-SD, IIV3-HD, IIV3-Adj, IIV4-SD, RIV, placebo) and 52 202 participants (see online supplemental appendix 19 (additional file 1) for study definitions). The network geometry was informed by eight intervention comparisons with a range of 1–3 RCTs. Results suggested that vaccines may reduce incidence of LCI compared with placebo, but with varying degrees of precision of effect estimates, although with no appreciable between-study network heterogeneity (I 2 =0%, τ=0.00). IIV3-HD (OR 0.23, 95% CI (0.11 to 0.51), P-score=0.78; low certainty of evidence, VE 72.85 95% CI (43.5 to 86.64)) and RIV (OR 0.25, 95% CI (0.08 to 0.73), P-score=0.73; low certainty of evidence, VE 70.63 95% CI (22.86 to 90.21)) were the most effective vaccines. These were followed by IIV3-Adj vaccine (OR 0.24, 95% CI (0.04 to 1.47), P-score=0.65; low certainty of evidence, VE 71.73 95% CI (−34.45 to 95.06)) ( table 4 , online supplemental appendices 17C and 20 (additional file 1) ).
Summary of results in outcomes with pairwise and network meta-analysis
Results were imprecise in sensitivity analyses restricting to studies with percentage of female above 50% and matched vs mismatched circulating strains ( online supplemental appendix 18C (additional file 1) ). When formulations were combined (ie, Adj, SD, HD, RIV), RIV performed best (OR 0.22, 95% CI (0.10 to 0.49), P-score=0.78, 95% PI (0.08, 0.63), I 2 =0%, τ=0.00). Precise results were identified for SD (OR 0.31, 95% CI (0.15 to 0.67), P-score=0.33, 95% PI (0.12 to 0.85)) and HD (OR 0.23, 95% CI (0.11 to 0.50), P-score=0.71, 95% PI (0.08 to 0.64)).
Influenza-like illness
NMA with original coding of interventions was not possible for ILI. Pairwise meta-analysis suggested that IIV3-SD may reduce ILI incidence compared with placebo, but the result was imprecise (2 RCTs, 46 96 854 participants; OR 0.39, 95% CI (0.15 to 1.02), I 2 =8%, τ=0.21 low certainty of evidence, VE 57.79 95% CI (−1.75 to 83.22)) ( table 5 , online supplemental appendices 21–23 (additional file 1) ) for study outcome definitions).
Summary of results in outcomes with pairwise meta-analysis
In a sensitivity analysis, we combined available vaccine formulations from IIV3-Adj, IIV4-Adj, IIV3-HD, RIV, IIV3-SD, IIV4-SD, Tdap and placebo to Adj, HD, RIV, SD, Tdap and placebo. In this case NMA was possible, including 9 RCTs, 46 49 55–57 60 62 76 96 5 interventions (Adj, HD, RIV, SD and Tdap) compared with placebo, and 65 658 participants. HD performed best, yet the 95% PI suggested that new evidence may change results (OR 0.38, 95% CI (0.15 to 0.93), P-score=0.77, 95% PI (0.09 to 1.63), I 2 =0%, τ=0.00). Similar precision of the effect estimate was identified for RIV (OR 0.38, 95% CI (0.15 to 0.94), P-score=0.68, 95% PI (0.09 to 1.66)) and SD (OR 0.38, 95% CI (0.16 to 0.95), P-score=0.57, 95% PI (0.09 to 1.67)), yet PIs were wide ( online supplemental appendix 17C (additional file 1) ).
Secondary outcomes analysed in both network and pairwise meta-analysis
Number of vascular adverse events.
The NMA for the number of vascular adverse events included 8 RCTs, 55 56 58 59 71 78 94 5 interventions (IIV3-SD, IIV3-HD, IIV3-Adj, IIV4-HD, IIV4-Adj), and 57 677 participants (see Appendices 17, 18, and 24 ( online supplemental appendices 17, 18, and 24 (additional file 1) ) for study outcome definitions). The network geometry was informed by four intervention comparisons with a range of 1–4 RCTs. Results suggested that HD and Adj vaccines prevented more vascular adverse events compared with IIV3-SD, but with large dispersion among studies (I 2 =97%, τ=0.34). IIV4-Adj was ranked the most efficacious intervention and may be associated with lower vascular adverse events (IRR 0.18, 95% CI (0.07 to 0.43), P-score=0.98, 95% PI (0.03 to 1.07); very low certainty of evidence) followed by IIV4-HD (IRR 0.46, 95% CI (0.21 to 1.00), P-score=0.71, 95% PI (0.09 to 2.41); low certainty of evidence) compared with IIV3-SD ( table 4 , online supplemental appendix 17C (additional file 1) ). However, PIs were wide and evidence of low certainty. Results were similar when restricting to RCTs with low overall ROB, and there was low between-study heterogeneity (I 2 =0%, τ=0.00), but more imprecision around the summary effect estimates.
Outpatient visits
The NMA for the outpatient visits included 4 RCTs, 46 55 56 96 3 interventions (IIV3-SD, IIV3-HD, placebo) and 41 995 participants. The network geometry was informed by two intervention comparisons each of which included two RCTs. IIV3-SD (OR 0.64, 95% CI (0.36 to 1.14)) and IIV3-HD (OR 0.65, 95% CI (0.35 to 1.19)) may be superior to placebo in being less associated with visits, but with imprecise effect estimates, moderate between-study network heterogeneity (I 2 =61%, τ=0.11), and very low certainty of evidence ( online supplemental appendix 17C (additional file 1) ). Pairwise meta-analysis suggested an imprecise effect for IIV3-SD compared with placebo (OR 0.40, 95% CI (0.07 to 2.14), 2 RCTs, 814 participants, I 2 =77%, τ=1.08) ( table 4 ).
All-cause mortality
The NMA for all-cause mortality included 20 RCTs, 18 19 46 47 49 50 55–60 62 65 67 72 93 95 96 9 interventions (IIV3-SD, IIV3-HD, IIV3-Adj, IIV4-SD, IIV4-HD, IIV4-Adj, RIV, Tdap, placebo) and 140 577 participants ( table 4 ). The network geometry was informed by 11 intervention comparisons with a range of 1–6 RCTs. The effect of RIV on all-cause mortality may be comparable to placebo (OR 1.01, 95% CI (0.23 to 4.49), low certainty of evidence). Both RIV and placebo ranked among the best interventions (P-score: RIV 0.75, placebo 0.71). Despite the low between-study heterogeneity in the network (I 2 =0%, τ=0.00), effect estimates were highly imprecise with very low to moderate certainty of evidence ( online supplemental appendix 17C (additional file 1) . Pairwise meta-analysis estimate of IIV3-SD against placebo was highly uncertain (OR 1.47, 95% CI (0.43 to 5.06), 2 RCTs, 46 96 854 participants, I 2 =0%, τ=0.00).
Inconclusive results were provided in sensitivity analyses restricting to studies with percentage of female above 50% and overall low ROB ( online supplemental appendix 17C (additional file 1) ). One RCT 60 (6961 participants) showed that IIV3-Adj was associated with lower odds of influenza-related deaths compared with IIV3-SD (OR 0.75, 95% CI (0.17 to 3.36)), yet the estimate was imprecise. Another RCT 93 (1741 participants) suggested that IIV3-SD was associated with higher odds of influenza-related deaths compared with IIV4-SD, yet the result was imprecise (OR 3.01, 95% CI (0.12 to 73.92)) ( table 5 ).
This systematic review and NMA provides a comprehensive assessment of influenza VE in older adults. We included a total of 41 RCTs, involving over 200 000 participants. Our results indicated that influenza vaccines were effective in preventing LCI compared with placebo. IIV3-HD showed the most promising results in preventing LCI, followed by RIV, IIV3-Adj and IIV3-SD. However, precision of effect estimates varied among interventions with low overall certainty of evidence, except for the comparison IIV3-HD versus IIV3-SD where certainty was high. The findings highlight the potential benefits of IIV3-HD in preventing hospitalisations for ILI and hospitalisation for ARI, and of IIV4-Adj in reducing vascular adverse events among older adults. Overall, few trials were included in the intervention comparisons in LCI, ILI, vascular adverse events, hospitalisations for ILI and ARI, and influenza-related mortality outcomes. VE may differ across certain participant characteristics, such as age, sex or circulating strains between and within seasons. However, there was insufficient evidence to make definite conclusions or explore heterogeneity in populations with different characteristics (eg, comorbidities, frailty) or study characteristics (eg, circulating strains or between influenza season variability) that could modify the intervention effect. Within-study ROB was predominantly at some or high concerns across outcomes, while small-study effects and reporting bias were only possible to assess in all-cause mortality outcome with no overall concerns. Overall, our results should be interpreted with caution since uncertainty around the point estimates was high and certainty of evidence was primarily low. High certainty of evidence was in LCI (for 1 of the 15 comparisons), ILI-hospitalisation (for the single comparison), and ILI cases (for the single comparison), but moderate in vascular adverse events (for 1 of the 10 comparisons), all-cause mortality (for 15 of the 36 comparisons), ARI-hospitalisation (for the single comparison) and ER-visits (for the single comparison).
Our findings align with the systematic review and NMA conducted by Minozzi et al , 104 who assessed the efficacy and safety of influenza vaccines in 220 RCTs of 100 677 children, 329 127 adults, including older adults. Overall, NMA results from their review among the older adult population were consistent with our results in the sense that influenza vaccines are better than placebo for LCI, although with varying levels of precision in effect estimates. Differences in pairwise results between the two reviews were unremarkable. Another review from the Advisory Committee on Immunisation Practices 105 included 51 studies of which 27 were RCTs and 24 were non-randomised studies to assess the relative efficacy and safety of influenza vaccines in older adults. They did not conduct any RCT-based meta-analyses that could be compared with those in our review. Relative to both reviews, searches in our review were more comprehensive and up to date including 18 additional RCTs with 71 049 participants not previously included in these reviews. We also evaluated credibility of evidence using both GRADE and CINeMA approaches, which to our knowledge has not been done elsewhere.
The strengths of our study lie in its systematic approach, extensive knowledge user engagement, and rigorous analysis using NMA to compare various influenza vaccines. This integrated knowledge translation approach allowed for valuable insights throughout the research process, from refining the research question to interpreting the findings. We followed the Cochrane Handbook methods for systematic reviews, 30 and reviewers worked in pairs and independently for screening, data abstraction and ROB appraisal. We reported the results using the PRISMA-NMA 8 and GRIPP-2 reporting statements. 9
However, some limitations should be considered. First, the limited number of studies constrained the analysis for specific outcomes, such as ILI, ER-visit for ILI, hospitalisation for ILI and ARI outcomes. The sparsity of networks, in some cases with only one or two studies per intervention comparison, increased the imprecision of effect estimates and reduced statistical power to detect differences in effects. Second, the lack of studies in certain outcomes comparing enhanced influenza vaccines limited the adequate assessment of the NMA assumptions, including exploring sources of heterogeneity (eg, through meta-regression analysis), small-study effects and inconsistency. Also, demographic variables that may explain heterogeneity, such as frailty, comorbidities and season intensities, were absent from the primary studies. RCTs usually were limited to one or two influenza seasons and because of the characteristics of influenza viruses (high mutation) and the history of exposure to influenza viruses in the population (herd immunity, effect of repeated vaccination and protection from previous exposure to influenza viruses) it is very hard to predict the efficacy of influenza vaccines in future seasons. Also, studies usually include relatively healthy populations, and there is a need for high quality RCT among older frail adults. Heterogeneity among studies varied in NMA from low to severe, but in all pairwise meta-analyses it was generally low, except for the inpatient hospitalisation outcome. While no major concerns were identified for small-study effects and publication bias for the all-cause mortality outcome, we could not assess this for any other outcome. For LCI and all-cause mortality outcomes where it was possible to assess, there was no evidence of statistical inconsistency. The transitivity assumption was likely satisfied regarding the overall ROB and participants’ age. We conducted a subgroup analysis of LCI with respect to whether vaccine strains matched the circulating strains. A more targeted analysis would have looked at specific strains; however, there was an insufficient number of studies to undertake such an analysis. Lastly, approximately half of the included RCTs reported races other than white/Caucasian, but these studies included predominantly white participants. Future studies are needed to capture influenza VE in racial diversity, so that they are representative of populations where trials are conducted.
Our study contributes valuable insights to inform evidence-based public health decisions and immunisation guidelines for older adults (eg, through the NACI Influenza Working Group). In particular, the NACI Supplemental Guidance on Influenza Vaccination in Adults 65 Years of Age and Older is anticipated to be published on the Government of Canada website in Spring 2024. Policy-makers and healthcare providers should consider these findings when formulating immunisation strategies to protect older and vulnerable populations from seasonal influenza and its complications. Annual vaccination with any vaccine is the best way to prevent infection and its complications.
Conclusions
Our analysis of RCT data highlights efficacy associated with IIV3-HD and RIV vaccines in protecting elderly persons against LCI. RIV vaccine may reduce all-cause mortality when compared with other vaccines we examined, but the evidence is uncertain. Moreover, our analysis points to a possible association of higher all-cause mortality in elderly persons receiving adjuvant vaccines (IIV3-Adj and IIV4-Adj). The comparative efficacy of individual vaccines regarding LCI, ILI, and secondary outcomes (eg, influenza-related mortality) remains uncertain. Differences among the influenza vaccines are possible and might emerge with accumulation of new evidence.
Our results should be interpreted with caution due to imprecise and heterogeneous summary effects with low certainty of evidence of the RCT studies we included. VE may vary depending on population (eg, frailty, comorbidities) virus strain matching and study characteristics. More high-quality RCTs are necessary that encompass multiple influenza seasons to evaluate the relationship between these factors and VE, such as seasonal vaccines specifically based on RIV and IIV4 formulations.
Ethics statements
Patient consent for publication.
Not applicable.
Acknowledgments
The authors wish to thank Huda Ashoor, Areej A. Hezam, Myanca Rodrigues, Ji Yoon Kim, Patricia Rios, Amruta Radhakrishnan and Kelsey Young for their contributions in screening and data abstracting the studies. We thank Brahmleen Kaur, Kiran Ninan and Navjot Mann for their support in formatting the paper and creating the EndNote library. We thank Dr Jessie McGowan for developing the literature searches, Tamara Rader for peer-reviewing the literature search, and Raymond Daniel for conducting the literature search. We thank the National Advisory Committee on Immunisation Influenza Working Group for their content expertise.
- Lafond KE ,
- Porter RM ,
- Whaley MJ , et al
- Noorduyn SG , et al
- Iuliano AD ,
- Roguski KM ,
- Chang HH , et al
- Centers for Disease Control and Prevention
- Alizadeh K ,
- Alizadeh K , et al
- Harding AT ,
- Salanti G ,
- Caldwell DM , et al
- Staniszewska S ,
- Simera I , et al
- Higgins J ,
- Chandler J , et al
- Straus SE ,
- McGowan J ,
- Sampson M ,
- Salzwedel DM , et al
- Brozek JL ,
- Alonso-Coello P , et al
- Guyatt GH ,
- Kunz R , et al
- Eldridge S ,
- Gravenstein S ,
- Davidson HE ,
- Han LF , et al
- Taljaard M , et al
- McConeghy KW ,
- Canaday DH , et al
- Sterne JAC ,
- Savović J ,
- Page MJ , et al
- Flemyng E ,
- Boutron I , et al
- Chaimani A ,
- Higgins JPT ,
- Mavridis D , et al
- Barberis I ,
- Ault SK , et al
- Hartung J ,
- Veroniki AA ,
- Jackson D ,
- Bender R , et al
- Higgins JP ,
- Veroniki AA
- Koch A , et al
- Viechtbauer W , et al
- DerSimonian R ,
- Viechtbauer W
- Thompson SG ,
- Deeks JJ , et al
- Schwarzer G
- Welton NJ ,
- Barrett JK , et al
- Tricco AC ,
- Soobiah C , et al
- Del Giovane C ,
- Jackson D , et al
- Balduzzi S ,
- Nikolakopoulou A , et al
- Nikolakopoulou A ,
- Papakonstantinou T , et al
- Vist GE , et al
- Regan M , et al
- Herrington D , et al
- Belongia EA ,
- Levine MZ ,
- Olaiya O , et al
- Reynales H ,
- Poder A , et al
- Chang L-J ,
- Janosczyk H , et al
- Cowling BJ ,
- Perera RAPM ,
- Valkenburg SA , et al
- de Bruijn IA ,
- Gerez L , et al
- Della Cioppa G ,
- Nicolay U ,
- Lindert K , et al
- DiazGranados CA ,
- Dunning AJ ,
- Jordanov E , et al
- Kimmel M , et al
- Dunkle LM ,
- Izikson R ,
- Patriarca P , et al
- Rosen J , et al
- Falsey AR ,
- Treanor JJ ,
- Tornieporth N , et al
- Reyes MRA-DL ,
- Reynales H , et al
- Leffell DJ ,
- Bock SA , et al
- Keitel WA ,
- El Sahly HM , et al
- Andrew MK ,
- Loeb M , et al
- McLean HQ ,
- King JP , et al
- Ross TM , et al
- Matassa V ,
- Ciarlet M , et al
- Donazzolo Y ,
- Jambrecina A , et al
- Register ECT
- Rudenko LG ,
- Grigorieva E , et al
- Sanchez L ,
- Matsuoka O ,
- Inoue S , et al
- Scheifele DW ,
- McNeil SA ,
- Ward BJ , et al
- Schmader KE ,
- Harrington T , et al
- Szymczakiewicz-Multanowska A ,
- Bugarini R , et al
- Lattanzi M ,
- Izu A , et al
- Leung VKY ,
- Mordant FL , et al
- Treanor J ,
- Dumyati G ,
- O’Brien D , et al
- Treanor JT ,
- Albano FR ,
- Sawlwin DC , et al
- Strout CB , et al
- Vardeny O ,
- Udell JA , et al
- Wongsurakiat P ,
- Maranetra KN ,
- Wasi C , et al
- Pregliasco F ,
- Serpilli W , et al
- Campbell JD ,
- Chambers CV ,
- Brady RC , et al
- Winokur P ,
- Brady R , et al
- Ferguson M ,
- Davis M , et al
- Phrommintikul A ,
- Kuanprasert S ,
- Wongcharoen W , et al
- Tinoco JC ,
- Pavia-Ruz N ,
- Cruz-Valdez A , et al
- Minozzi S ,
- Gianola S , et al
Supplementary materials
Supplementary data.
This web only file has been produced by the BMJ Publishing Group from an electronic file supplied by the author(s) and has not been edited for content.
- Data supplement 1
- Data supplement 2
- Data supplement 3
- Data supplement 4
X @AVeroniki, @AStevens_PhD, @ivand_florez, @76Juano
Contributors AAV, SS and ACT designed the study. ACT and SS obtained funding. SST, MG and JD coordinated the study. SST, JD, MG, PK, VN, MC, RR, AP and CS screened articles, abstracted data and carried out risk of bias assessments. AAV and MK conducted data analyses. IDF and JJY-N conducted GRADE certainty of evidence assessments. AAV drafted the first version of the manuscript. All authors contributed to the manuscript’s revision and interpretation of findings. AAV is the guarantor. The corresponding author attests that all listed authors meet authorship criteria and that no others meeting the criteria have been omitted.
Funding This work was funded by a Canadian Institutes of Health Research Drug Safety and Effectiveness Network (No. DMC–166263). SS is funded by a Tier 1 Canada Research Chair in Knowledge Translation and Quality of Care, the Mary Trimmer Chair in Geriatric Medicine, and a Foundation Grant (Canadian Institutes of Health Research). ACT holds a Tier 2 Canada Research Chair in Knowledge Synthesis. MC was part supported by the Health Research Board (Ireland) and the HSC Public Health Agency (Grant number CBES-2018-001) through Evidence Synthesis Ireland/Cochrane Ireland.
Competing interests None declared.
Patient and public involvement Patients and/or the public were involved in the design, or conduct, or reporting, or dissemination plans of this research. Refer to the Methods section for further details.
Provenance and peer review Not commissioned; externally peer reviewed.
Supplemental material This content has been supplied by the author(s). It has not been vetted by BMJ Publishing Group Limited (BMJ) and may not have been peer-reviewed. Any opinions or recommendations discussed are solely those of the author(s) and are not endorsed by BMJ. BMJ disclaims all liability and responsibility arising from any reliance placed on the content. Where the content includes any translated material, BMJ does not warrant the accuracy and reliability of the translations (including but not limited to local regulations, clinical guidelines, terminology, drug names and drug dosages), and is not responsible for any error and/or omissions arising from translation and adaptation or otherwise.
Read the full text or download the PDF:
- Open access
- Published: 14 February 2020
Better influenza vaccines: an industry perspective
- Juine-Ruey Chen 1 na1 ,
- Yo-Min Liu 2 , 3 na1 ,
- Yung-Chieh Tseng 2 &
- Che Ma ORCID: orcid.org/0000-0002-4741-2307 2
Journal of Biomedical Science volume 27 , Article number: 33 ( 2020 ) Cite this article
25k Accesses
64 Citations
30 Altmetric
Metrics details
Vaccination is the most effective measure at preventing influenza virus infections. However, current seasonal influenza vaccines are only protective against closely matched circulating strains. Even with extensive monitoring and annual reformulation our efforts remain one step behind the rapidly evolving virus, often resulting in mismatches and low vaccine effectiveness. Fortunately, many next-generation influenza vaccines are currently in development, utilizing an array of innovative techniques to shorten production time and increase the breadth of protection. This review summarizes the production methods of current vaccines, recent advances that have been made in influenza vaccine research, and highlights potential challenges that are yet to be overcome. Special emphasis is put on the potential role of glycoengineering in influenza vaccine development, and the advantages of removing the glycan shield on influenza surface antigens to increase vaccine immunogenicity. The potential for future development of these novel influenza vaccine candidates is discussed from an industry perspective.
Seasonal influenza outbreaks cause 3 to 5 million cases of severe illness and 290,000 to 650,000 respiratory deaths each year [ 1 , 2 ]. The Orthomyxoviridae are a family of enveloped viruses with a genome consisting of 6~8 segments of negative-sense single-stranded RNA, including four genera of influenza virus: A, B, C and D [ 3 ]. Influenza A and B are the main cause of annual flu outbreaks in humans, with influenza A further classified into subtypes based on their surface glycoproteins hemagglutinin (HA) and neuraminidase (NA). 18 HA subtypes (H1~H18) and 11 NA subtypes (N1~N11) are currently known, most notable today are the H1N1 and H3N2 subtypes that co-circulate in the human population. Since the 1970s influenza B has diverged into two lineages based on antigenicity, the Yamagata and Victoria lineages, with little or no serum cross-reactivity [ 4 ]. In contrast to the severity and epidemic potential of influenza A and B, influenza C infections induce only mild flu symptoms in children, while influenza D is not known to infect humans [ 5 ].
Recurrent influenza epidemics with pre-existing immunity occurs because the influenza virus employs two mechanisms to escape recognition: antigenic drift and antigenic shift. Antigenic drift is the gradual accumulation of point mutations on the influenza virus’ surface glycoproteins HA and NA, driven by high error rates (estimated at 1.5 × 10 − 5 per nucleotide per replication [ 6 ]) of the virus’ RNA-dependent RNA polymerase (RdRP). Mutations that allow the virus to evade the host immune system are positively selected for and become fixed, resulting in the rise of new strains that are antigenically different from what the host was vaccinated against. The second escape mechanism, antigenic shift, is the reassortment of gene segments across different strains infecting the same host, resulting in a wholesale change in antigenicity [ 7 , 8 ]. Antigenic shift have historically been associated with influenza pandemics, the most recent example being the 2009 swine-origin H1N1 that included segments from classical swine H1N1, Eurasian swine H1N1, and a triple reassortant from 1998 [ 9 ]. The rise of new strains through antigenic drift and shift is followed by cross-immunity mediated competition between antigenically similar strains, which results in a progressive replacement of existing strains with new variants [ 10 , 11 ].
Unfortunately, current seasonal influenza vaccines are strain-specific and have a very narrow range of coverage, meaning extensive surveillance, accurate predictions and annual vaccination are needed as circulating strains evolve continuously over time. This task is coordinated by the World Health Organization (WHO) Global Influenza Surveillance and Response System (GISRS), which gathers year-round data from hundreds of national influenza centers around the world and issue vaccine formulation recommendations for each upcoming flu season [ 12 ]. When vaccine strains are well-matched with circulating strains, vaccination provides healthy adults younger than 65 years with 70–90% protection [ 13 ], and reduced hospitalizations in the elderly and those with chronic illnesses by 30–70% [ 14 , 15 , 16 ]. However, in years when there is a mismatch between the vaccine and circulating strains, the vaccine effectiveness (VE) tends to be much lower [ 17 ].
Here we discuss various challenges the current seasonal flu vaccine is facing, and how a universal influenza vaccine approach through carbohydrate design to elicit broadly neutralizing antibodies (bnAbs) targeting the influenza HA glycoprotein can potentially play a role in the future of influenza prevention. Despite the first influenza vaccine being commercially available as early as 1945, influenza outbreaks continue to be a major public health concern today. It is imperative for health authorities, researchers and the pharmaceutical industry to work together on improving the efficacy of influenza vaccines.
Limitations and drawbacks of current influenza vaccines
Traditional trivalent influenza vaccines include two inactivated influenza A strains (H1N1 and H3N2) and one influenza B strain, but this has recently been overtaken by quadrivalent influenza vaccine comprised of H1N1, H3N2 and both influenza B lineages that offers a more complete coverage [ 18 ]. Commercially available vaccine options include egg- or cell-based inactivated influenza vaccine (IIV), a live attenuated influenza vaccine (LAIV), and a recombinant HA vaccine produced in insect cells [ 16 ].
Egg-based inactivated influenza vaccines
The production of egg-based influenza vaccines has remained virtually unchanged since the advent of split (subvirion) vaccines in the 1970s, and still commands 88% of the global market share in 2018 [ 19 ]. The main advantages of the egg-based platform include an excellent production capacity that is capable of producing an estimated 1.5 billion doses annually, and a low production cost that allows global access to the vaccine [ 20 ].
The strain-specific nature of current vaccines necessitates the annual selection of candidate vaccine viruses (CVVs), including screening the antigenicity of isolates, preparing reassortant viruses, and adaptation of the virus to eggs (Fig. 1 ). For egg-based manufacture, the entire process from strain selection to vaccine availability typically takes 6~8 months with tight time constraints, and any unexpected circumstance such as a delayed WHO strain recommendation [ 21 ] or unexpected low virus yield [ 22 ], can snowball into significant production delays and directly affect vaccine supply. This lengthy interval also gives circulating influenza viruses time to mutate, as it did during the 2014–2015 flu season when late-emerging H3N2 variants rendered the recommended vaccine strain ineffective [ 8 ].
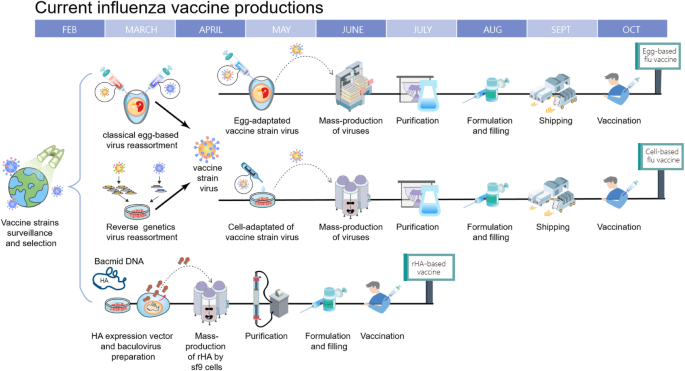
Timeline of current influenza vaccine production methods. Schematic overview of egg-based, cell-based and protein-based influenza vaccine production. Vaccine strains that match circulating influenza viruses for the upcoming flu season are selected by the World Health Organization (WHO) Global Influenza Surveillance and Response System (GISRS). High yielding vaccine strains for egg- or cell-based production are generated by either classic or reverse genetic reassortment. These adapted viruses go into mass production, either in embryonated chicken eggs or MDCK cells with a production timeline of approximately six to eight months. In recombinant HA (rHA) vaccines, the HA sequence is cloned into baculovirus and expressed by insect cells, significantly shortening production time
A second drawback of using an egg-based platform stems from the adaptation process of culturing a human virus in avian tissue, where adaptive mutations may accumulate and potentially change the strain’s antigenicity [ 23 , 24 , 25 ]. HA, apart from being the primary target for neutralizing antibodies, is the main facilitator of influenza virus entry by binding to sialic acids on the surface of the host cells. Human influenza HA preferentially bind to α-2,6 linked sialic acids commonly found on epithelial cells in the human upper respiratory tract [ 26 , 27 ]. However, in egg-based production vaccine strains are inoculated into the allantoic cavity of embryonated chicken eggs which only contain α-2,3 linkages [ 28 ]. With successive passages, this becomes a selective pressure that can cause the acquisition or a total shift in receptor specificity, with its accompanying mutations and antigenic changes on HA’s receptor binding site. A recent example of this occurred during the 2016–2017 flu season, when egg-adapted vaccine strains were found to lack a glycosylation site (T160, H3 numbering) on H3N2 HA antigenic site B, one of the five major antigenic sites that induce neutralizing antibodies [ 24 ].
A third concern is the egg-based platform relies on a steady supply of embryonated eggs. This egg supply can be overwhelmed by sudden increases in demand, such as during a pandemic.
Live attenuated influenza vaccines
LAIV is generated by combining the HA and NA of currently circulating strains with the internal proteins of an attenuated cold-adapted strain. This results in a reassortant vaccine virus that can be administered intranasally and has some limited replicative ability in the human upper respiratory tract. As the entire influenza replication cycle is utilized at the site of infection, LAIV has also been reported to elicit cell-mediated immunity [ 29 ] and local mucosal immunity [ 30 ] besides the induction of a robust antibody response. Clinically, LAIV has shown variable but overall comparable efficacy to IIV in adults and better efficacy in children.
Recently however, the necessity of effective replication in human respiratory tissue has emerged as an area of concern. The US Advisory Committee on Immunization Practices (ACIP) recommended against LAIV between 2016 to 2018 due to low efficacy of the H1N1 component [ 31 ], although this phenomenon was not noted in Europe and Canada [ 32 ]. The reason for this lack of efficacy is still unclear, but possibilities include viral interference of tetravalent vaccine strains resulting in reduced virus shedding for the weakest strain, strong cross-reactive antibodies from previous seasons preventing virus replication, and inherent lower replication in host tissue by the H1N1 pandemic strain [ 33 ], among others. ACIP has since resumed recommendation for LAIV in 2018 following a change in the H1N1 vaccine component [ 34 ].
Secondly, as currently-available LAIV is also produced in embryonated chicken eggs, it is plagued by many of the same concerns as egg-based IIV. In 2019 AstraZeneca’s LAIV product FluMist experienced manufacturing issues due to low yields in two strains, resulting in a reduction in shipments worldwide [ 35 ].
Cell-based and recombinant HA vaccines
In order to overcome limitations of the egg-based manufacturing process, production systems using mammalian or insect cell cultures have emerged [ 36 , 37 ].
The manufacturing process for cell-based IIV is similar to egg-based IIVs, but has several advantages over the latter (Fig. 1 ). Viral production in a cell culture bioreactor is more flexible, more scalable and unaffected by egg shortages. Additionally, recent comparisons have shown that cell-based vaccines provided a moderately higher VE for elderly individuals ( ≧ 65 years old) than egg-based vaccines, possibly due to less egg-adapted mutations [ 38 ].
For recombinant HA production in insect cells, the baculovirus expression system is utilized to manufacture recombinant HA, which is then purified and formulated into HA trimer “rosettes” [ 39 ]. This not only has the same benefits of speed, flexibility and scalability as cell-based IIV, but also eliminates the reliance on influenza virus replication for vaccine production and the time-consuming process of strain selection. FluBlok, a recombinant HA vaccine developed by Sanofi Pasteur, was found to be 30% more efficacious than traditional IIV for people ≧ 50 years old [ 40 ].
However, the comparatively high cost of these alternatives to egg-based influenza vaccines have prevented them from taking a bigger share of the influenza vaccine market. According to the US Centers for Disease Control (CDC) adult influenza vaccine contract pricing for 2019–2020, the cost of the cell-based vaccine Flucelvax is approximately 40% higher than an inactivated egg-based vaccine produced by the same manufacturer. The recombinant HA vaccine Flublok can be more than twice as expensive as egg-based vaccines [ 41 ]. Additionally, while cell-based and recombinant vaccines have the benefit of speed and flexibility that is critical for pandemic preparedness, it does not translate to a competitive advantage on the seasonal vaccine market [ 42 ]. So far slow progress has been made to transition away from egg-based production, and more support from governments around the world is needed.
Next-generation influenza vaccines
Various next-generation influenza vaccines under development aims to broaden or lengthen the human immune response with novel antigens and adjuvants, gradually expanding the strain-specific nature of current vaccines to include all strains within a subtype (eg all H1 strains), multiple subtypes (eg H1/H5/H9), or incorporating all subtypes within a group (influenza A group 1 or group 2), with the ultimate goal of creating a truly “universal” pan-influenza vaccine that can elicit lifelong immunity against all influenza A and B viruses [ 43 ].
From a public health perspective influenza continues to be the only human disease that requires annual vaccination. It is estimated that replacing just 10% of seasonal vaccines with a universal vaccine would avert 6300 influenza-related deaths and save 1.1 billion US dollars in direct healthcare costs per year in the United States alone [ 44 ]. In 2017, the National Institute of Allergy and Infectious Diseases (NIAID) in the US laid out a detailed strategic plan for the development of a universal influenza vaccine, highlighting knowledge gaps and research areas in pursuit of this common goal [ 43 ]. In their outline, they established four criteria for a universal influenza vaccine as: 75% effectiveness against symptomatic influenza infection, protection against both group I and group II influenza viruses, durable protection that last at least 1 year, and be suitable for all age groups. It is with these criteria in mind that we discuss various vaccine candidates being developed (Table 1 ).
Altering glycan composition on recombinant HA and split virus vaccines
Historically, a crucial strategy of influenza virus’ escape from pre-existing immunity is the addition of N-glycosylation sites on the immunodominant HA head domain [ 75 ]. These bulky but poorly-immunogenic N-glycans allow the virus to hide antigenically-conserved domains from host immune system recognition [ 76 ], a mechanism known as “glycan shielding”.
When H1N1 first emerged in 1918, it carried only one conserved glycosylation site at position 104 (H1 numbering) on the HA head. But as the virus continued circulating in the human population up to the 1950s, it sequentially acquired glycans at positions 144, 172 and 177, all at or adjacent to the major antigenic site Sa on the HA head. This was followed by a 20-year hiatus as H1N1 was supplanted by H2N2, before re-emerging in 1977 carrying the same three acquired and one conserved glycosylation sites as before. The following decades saw N144 replaced by N142, the disappearance of N172, and the acquisition of N71 before the glycan shield was ultimately reset due to the emergence of 2009 pandemic H1N1, carrying only the original conserved glycosylation site on 104 [ 77 ]. Conversely, H3N2 circulated in 1968 carrying two glycans on its HA head, N81 and N165 (H3 numbering). Although the glycosylation site at position 81 was subsequently lost, positions 63, 122, 126, 133, 144, and 246 were accrued and retained [ 78 ]. Overall, the continued circulation of an influenza subtype in the human population corresponds to a steady increase in N-glycans on its HA head domain. Evidence that these acquired N-glycans provide a shielding effect comes from not only the observation that they tend to appear on or near major antigenic sites, but also studies have shown the acquisition of sites 177 and 142 on H1N1 slow genetic drift in the shielded areas [ 79 ], and mutational deletion of 177, 142 and 71 on a pre-pandemic H1N1 strain elicited a protective immune response against the 2009 pandemic H1N1 strain [ 77 ]. Similarly, in H3N2 positive selection disappeared when an antigenic site becomes shielded by N-glycans [ 78 ], and the introduction of five recent glycosylation sites at positions 63, 122, 126, 133 and 246 allowed a 1968 H3N2 strain to evade polyclonal human serum raised against it [ 80 ].
These observations indicate that exposing the comparatively conserved, glycan-shielded regions of viral hemagglutinin could be a potential strategy to increase the breadth of influenza vaccine protection [ 52 , 81 , 82 ]. However, previous attempts have shown complete de-glycosylation of all carbohydrate moieties on influenza HA by either prokaryotic production [ 52 ], tunicamycin treatment [ 83 ] or PNGase F digestion [ 53 ] does not appear to be a viable strategy. Conserved N-glycosylation sites on the HA stem are essential for intracellular transport, correct glycoprotein folding and HA trimerization [ 84 ], and a completely unglycosylated HA would have a high chance of altered antigenicity.
Therefore, our group focuses on harnessing glycoengineering techniques to alter N-glycan composition on the HA, creating recombinant HAs that retain only a single N-acetylglucosamine (GlcNAc) attached to asparagine per N-glycosylation site (monoglycosylated HA, or HA mg ). To accomplish this, N-acetylglucosaminyltransferase I deficient (GnTI − ) human embryonic kidney cells that are unable to synthesize complex type N-glycans were used to produce secreted, transmembrane domain truncated HAs that have only high mannose residues on their N-glycosylation sites. These high mannose HAs were then further trimmed with the high mannose-cleaving enzyme endoglycosidase H leaving a single GlcNAc residue, dramatically decreasing the size and shielding effect of these N-glycans while still maintaining the native HA structure in its trimeric state.
Antibodies raised against HA mg inoculation demonstrated better binding affinity, neutralization and cross-reactivity than the unprocessed HA (fully glycosylated HA, or HA fg ) [ 52 , 53 ]. HA mg also induced the maturation of dendritic cells, more splenic granzyme B-secreting CD8 + T cells, and elicited a more diverse HA-specific B-cell repertoire than that of HA fg when used as a vaccine (Fig. 2 ). In terms of cross-protection, inoculation with an H1N1 pre-pandemic Bris/07 HA mg not only provided better protection in mice against laboratory strains WSN and PR8, but also offered 70% protection against a pandemic strain [ 52 , 53 ].
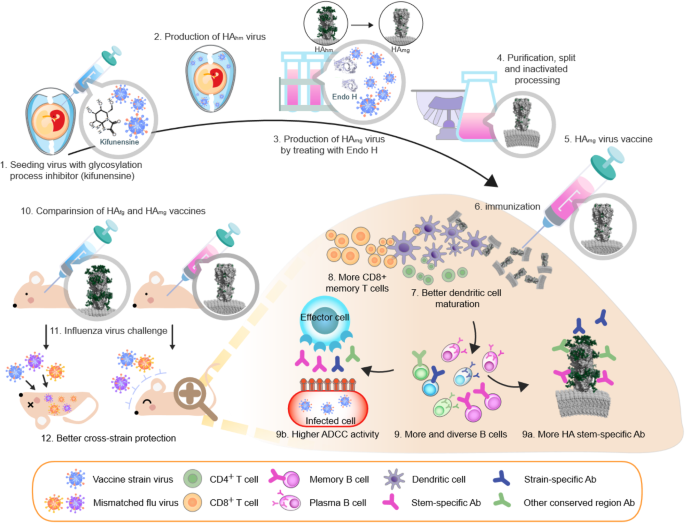
The production and immune response of monoglycosylated influenza vaccine. The production of monoglycosylated split virus vaccine adds two key steps to the traditional egg-based platform. Kifunensine, a mannosidase I inhibitor, is added during egg inoculation to arrest viral glycoprotein processing, resulting in a uniformly high mannose composition. Endoglycosidase H is added after harvest to trim high mannose residues down to a single GlcNAc. The resultant monoglycosylated split vaccine provides a more diverse immune response and more effective cross-strain protection than conventional egg-based vaccines. HA fg , non-modified egg-based vaccine with complex type N-glycans attached to HA; HA hm , HA with only high mannose type N-glycans; HA mg , HA with a single GlcNAc at its N-glycosylation sites. Models of HA fg , HA hm and HA mg are created with Protein Data Bank ID code 3LZG and 6FYT by adding glycan with GlyProt ( http://www.glycosciences.de/modeling/glyprot/php/main.php ), coot ( https://www2.mrc-lmb.cam.ac.uk/personal/pemsley/coot/ ) and PDB of lipid bilayer from Lipid Bilayer Membranes for RasMol ( https://www.umass.edu/microbio/rasmol/bilayers.htm ). The images were displayed with program PyMOL ( www.pymol.org )
While a recombinant HA mg vaccine would have all the advantages of a cell culture production system including speed, flexibility and safety, egg-based production remains the mainstay of influenza vaccine manufacture today. Devising a simple method to apply the monoglycosylation concept to egg-based vaccines with minimal modification will allow this procedure to be integrated into established production methods. Extensive testing found that kifunensine, an α-mannosidase I inhibitor, can be injected into embryonated eggs to convert influenza virus membrane glycoproteins to a uniformly high mannose composition. After harvesting these virions their high mannose N-glycans were then trimmed with endoglycosidase H to create intact monoglycosylated virus particles, and all participating reagents are removed in subsequent purification steps [ 54 ].
Like the recombinant HA mg before, monoglycosylated split inactivated influenza vaccines produced by kifunensine and endoglycosidase H treatment were shown to have higher neutralization and cross-neutralization activity, higher hemagglutination inhibition (HAI), more HA stem selectivity, and higher antibody dependent cellular cytotoxicity (ADCC) (Fig. 2 ). A monoglycosylated pandemic H1N1 split virus vaccine offered cross-protection against strains as diverse as the pre-pandemic NC/99 and the laboratory strain WSN [ 54 ]. Aside from having simplified glycans, this procedure produces antigens that are virtually identical to the current influenza vaccine, and would presumably offer a similar safety profile.
Recombinant HA vaccines
An adjuvanted recombinant HA trivalent nanoparticle influenza vaccine (tNIV) has been developed by Novavax using the baculovirus expression system to produce recombinant HAs, which were then purified and mixed with polysorbate 80 to form protein-detergent nanoparticles of 2~7 HA trimers [ 45 ]. The administration of this tNIV with a saponin adjuvant (Matrix-M) in ferrets induced higher levels of neutralizing antibodies against a panel of A (H3N2) strains than a commercial inactivated vaccine (trivalent Fluzone). A Phase I/II clinical trial showed similar results in patients, where tNIV induced significantly greater HAI responses compared to trivalent Fluzone against not only previous strains, but a forward-drifted A/Singapore variant [ 46 ].
Another candidate is a chimeric HA (cHA) vaccine born from a collaboration between Icahn School of Medicine at Mount Sinai and GSK/NIH. This strategy originates from the observation that our immune system tends to focus on the immunodominant but highly variable HA head domain, while the subdominant conserved stem region has a better ability to elicit bnAbs. By sequential immunization with a cHA protein consisting of a stem from circulating strains coupled to an irrelevant HA head from exotic influenzas, the strategy is devised to re-direct our immune system to better stimulate stem-specific responses [ 48 ]. In a preclinical study, ferrets sequentially immunized with heterologous influenza strains including live attenuated influenza vaccine (LAIV) bearing an H8 head domain and an H1 stem domain (cH8/1) and a split-inactivated vaccine bearing an H5 head domain and an H1 stem domain (cH5/1), conferred superior protection against challenge with pandemic H1N1 virus following different prime-boost combinations and immunization regimens [ 49 ]. This approach is currently in collaboration with GSK in a phase I study, and clinical data will be obtained by the end of 2019.
Epitope-peptide based vaccines
Multimeric-001 (M-001) is a vaccine currently being developed by BiondVax Pharmaceuticals consisting of nine conserved B and T cell epitopes from HA, nucleoprotein (NP) and matrix 1 (M1) protein arranged in triplicate and put onto a single recombinant protein [ 57 ] . Phase I/II clinical studies have shown the M-001 vaccine induced both cellular and humoral immunity to influenza A and B strains as a standalone vaccine [ 58 ], and also enhanced seroconversion when used as a primer for elderly patients before inoculation with inactivated trivalent vaccines [ 85 ].
FLU-v is another epitope-based vaccine developed by SEEK (PepTcell) based on in silico multiple alignment of influenza sequences and prediction of possible T-cell epitopes. Six consensus sequences from influenza NP, M1 and matrix 2 (M2) proteins were identified and synthesized into a candidate vaccine. Flu-v has been shown to induce a specific CD8 + response against these conserved epitopes and confer protection against heterotypic infection in mice [ 59 ], and a Phase Ib challenge trial also showed that the blood cells from immunized subjects exhibited cross reactive immunity against different influenza viruses [ 62 , 63 ].
CodaVax is an LAIV being developed by Codagenix that takes advantage of inherent human codon pair bias to reconstruct the influenza viral genome with synonymous but sub-optimal codons. This results in viral proteins that have the same amino acid sequence and antigenicity as wild type strains but attenuated due to excessive use of rare codons [ 64 , 65 ]. In animal models, the vaccine is shown to be effective at lower doses than conventional LAIV [ 66 ]. CodaVax has scheduled a phase I/II trial in the first quarter of 2017.
M2SR is an M2 deficient single-replication LAIV being developed by FluGen. In this strategy the M2 sequence in the viral genome (critical for viral uncoating and assembly) is largely deleted, but viruses are produced in M2-expressing cells to generate infective virions. Therefore, after inoculation into a host the attenuated virus is unable to propagate infective progeny, limiting the infection to a single round of replication [ 67 ]. In a ferret model M2SR was found to be less susceptible to the negative effects of pre-existing immunity on drifted strains [ 68 ]. Initial results from a placebo-controlled phase II trial indicate that the vaccine was effective against a mismatched H3N2 challenge.
DNA-based vaccine
Inovio has made efforts to apply their Syncon® synthetic DNA vaccine platform to influenza. By sequence alignment and cluster grouping of HA they have generated four “micro-consensus” sequences within an influenza subtype, which were then cloned onto expression vectors and delivered to the vaccine recipient via in vivo electroporation [ 72 ]. In mouse and ferret models these micro-consensus sequences against H1N1, H3N2 and H7N9 were found to elicit protective immunity against lethal challenges.
M2 conserved domain vaccine
ACAM-FLU-A is an influenza M2 ectodomain vaccine developed by Acambis (now Sanofi Pasteur). Due to overlapping nucleotides with M1, the M2 ectodomain is highly conserved in influenza A viruses, but poorly immunogenic [ 74 ]. ACAM-FLU-A utilizes the Hepatitis B core (HBc) as a carrier to fuse three tandem repeats of the M2 ectodomain onto each HBc subunit, creating an immunogenic virus-like particle (VLP). Initial results showed intramuscular injection of the vaccine was able to generate anti-M2 ectodomain seroconversion in 90% of healthy volunteers [ 73 ]. However, after immunization M2-specific antibody titers steadily declined over a 1-year period [ 86 ], so combination with the other antigens or adjuvants might be necessary.
Challenges for universal influenza vaccine development
The need for accurate surrogate markers of ve for clinical study and licensing approval.
Precisely characterizing influenza immunity and correlates of immune protection is one of the three major areas for improvement outlined in NIAID’s strategic plan for a universal influenza vaccine [ 87 ]. Serological assays such as hemagglutination inhibition (HAI) and single radial hemolysis (SRH) have long been held by regulatory agencies as a correlate of protection for inactivated influenza vaccine licensure. European Medicines Agency’s (EMA) Committee for Human Medicinal Products (CHMP) criteria indicates that for seasonal influenza vaccine approval one of three conditions must be met: seroprotection (HI titer of ≧ 1:40 or SRH of 25 mm 2 ) rate of over 70%, seroconversion (4-fold increase in titer) rate more than 40%, or a geometric mean increase (pre- vs post-vaccination) of 2.5 times in healthy adults, and 60, 30%, 2.0x respectively for elders [ 88 ]. The US FDA Center for Biologics Evaluation and Research (CBER) follows a similar criterion for accelerated approval [ 89 ].
However, HAI and SRH assays may not always be applicable when it comes to LAIV or novel next-generation vaccines currently under development. HAI measures the antibody-mediated inhibition of erythrocyte agglutination caused by HA binding to sialic acids on the erythrocyte surface. As such, the assay only detects antibodies directed at the HA head domain where its receptor binding site is located. Universal vaccine strategies based on eliciting immune response against conserved epitopes on the HA stem domain, M2, M1 or NP would not be detected by the HAI assay. SRH detects the concentration of influenza-targeting antibodies by measuring a ring of hemolysis caused by the antibody-virus-erythrocyte complex activating the complement system [ 90 ]. While this method measures all serum antibodies against influenza surface antigens, it still does not recognize local mucosal immunity or cell-mediated immunity, such as immunization strategies that target M1 or NP [ 91 ].
This has led to the recognition that non-HAI or SRH assays need to be taken into account for regulatory approval of next-generation influenza vaccines [ 87 , 88 ], though challenges in standardization of assays and reproducibility between laboratories still need to be overcome. Finally, human challenge trials are gaining acceptance by regulatory agencies for universal vaccine development which may lack traditional serological correlates for protection [ 87 , 92 , 93 , 94 ]. There is increasing recognition that utilizing all aspects of our immune system are needed to control influenza outbreaks.
Eligibility for vulnerable groups
Elderly people often have more serious complications from influenza infections and a less robust immune response to vaccination [ 95 ]. Currently, high dose or adjuvanted IIVs are recommended for people 65 years and older, while LAIV is only approved for healthy adults up to the age of 49. On the other end of the spectrum, maternally-derived antibodies generated from inoculation during pregnancy are expected to provide protection for infants < 6 months, so vaccination that elicit a predominantly cell-mediated immune response are unlikely to be of use. Novel strategies for a universal flu vaccine will have to take into account differences in immune response from specific populations that are at higher risk for influenza complications.
Long-term protection
With traditional seasonal flu vaccine human immunity wanes in 6–8 months of time, enough to last through the influenza season [ 96 , 97 ]. But if a universal vaccine were to break the cycle of yearly vaccinations, long-term protection will be needed. Having durable protection for at least 1 year and preferably through multiple seasons is one of the four criteria set by the NIAID for a universal influenza vaccine [ 87 ], but how to achieve that goal is still unknown. Immunization schedules, formulations, dosages, and adjuvants will all likely have to be considered.
Conclusions
The evolution of influenza vaccine development has shown a trend of cell-based vaccines gradually taking the place of traditional egg-based manufacture. With the plethora of next-generation vaccines currently under development, WHO expects a universal influenza A vaccine to be in advanced clinical trials as early as 2027 [ 98 ]. Although many candidates have shown promising results in preclinical studies, demonstrating clinical safety and efficacy in a human population remains the most significant hurdle towards regulatory approval.
Our group has pioneered the strategy of exposing previously-shielded conserved epitopes on the HA through enzymatic trimming of N-glycans. This technique has been shown to elicit cross-neutralizing antibodies against antigenically diverse strains of influenza viruses within a subtype [ 52 , 53 ], and thus hypothetically a trivalent or tetravalent monoglycosylated vaccine containing the three influenza subtypes (H1, H3, and influenza B) circulating in the human population would be, for all intents and purposes, a universal flu vaccine.
We believe this monoglycosylated split virus vaccine strategy has three unique qualities that give it a significant advantage in the new drug developmental process:
The monoglycosylated split vaccine provides multiple conserved epitopes for immune recognition
Due to the rapid mutation rate of the influenza virus, using only a single conserved epitope as the antigenic target for universal vaccine runs the risk of generating escape mutants [ 99 , 100 ]. In our previous studies we have only demonstrated the concept that monoglycosylated split virus vaccine induces more stem-specific antibodies directed against conserved epitopes on the HA stem [ 54 ]. However, in theory by trimming off oligosaccharides on every N-glycosylation site on the HA, multiple conserved epitopes would be exposed, inducing a multi-faceted immune response that imposes a higher evolutionary barrier for escape mutant generation. Another influenza glycoprotein that could potentially benefit from the monoglycosylation process is NA. The preparation of monoglycosylated split virus vaccine would remove glycans from not only HA but also NA, hypothetically inducing more anti-NA antibodies that interfere with virus budding, disease progression and severity of symptoms [ 101 ].
The monoglycosylated split vaccine induces a similar immune response to current IIVs, meeting established surrogates of VE
Although a more diversified criteria encompassing CMI, neutralization assays and NA antibodies is encouraged, traditional serological assays remain the gold standard for regulatory approval. By incorporating our monoglycosylation technology onto the existing inactivated split vaccine platform, we could invoke a similar humoral response as conventional IIVs. Serological surrogates of vaccine efficacy such as HAI or SRH can be measured and non-inferiority comparisons with conventional vaccines can be made, opening up a well-trodden path towards licensure.
The monoglycosylated split vaccine is suitable for all age groups
Whether novel vaccine strategies that are effective on healthy adults are equally suitable for all age groups remains a concern. Due to having the same constituents as an IIV, the monoglycosylated split vaccine can be expected to offer a similar safety profile as the conventional flu vaccine. As such, it is possible that formulations suitable for different age groups, such as reduced dosage for children and high dose/adjuvanted vaccines for the elderly, can also be applied to our monoglycosylated split vaccine. Furthermore, the robust humoral immunity induced by IIV assures sufficient protection for infants < 6 months by maternal vaccination.
Even though recent advances in influenza vaccine manufacture such as cell-based and recombinant HA have allowed for a much quicker production timeline, using conventional strain-specific vaccines against a rapidly evolving influenza virus assures we are always playing catch-up. As our understanding of influenza pathogenesis and immune response continues to grow, developing a universal vaccine that provides long-lasting protection against divergent strains or subtypes is becoming an increasingly attainable goal. We believe our monoglycosylated split vaccine strategy that applies a simple modification step to pre-existing egg-based production platforms to provide broader immunity in the end product, is a significant step towards this goal.
Availability of data and materials
Not applicable.
Abbreviations
Antibody-dependent cellular cytotoxicity
broadly neutralizing Antibodies
Center for Biologics Evaluation and Research
Centers for Disease Control and Prevention
Committee for Human Medicinal Products
Cell Mediated Immunity
Candidate Vaccine Virus
European Medicines Agency
Food and Drug Administration
Global Influenza Surveillance and Response System
N-Acetylglucosamine
N-acetylglucosaminyltransferase I −
Hemagglutinin
fully glycosylated HA
Hemagglutination Inhibition
Monoglycosyalted HA
Hepatitis B core
Inactivated Influenza Vaccine
high-dose inactivated trivalent influenza vaccine
Live attenuated influenza vaccine
Matrix 1 protein
Matrix 2 protein
M2 knockout vaccine
Madin-Darby Canine Kidney
Microneutralization
Neuraminidase
National Institute of Allergy and Infectious Diseases
Nucleoprotein
RNA-dependent RNA polymerase
Single Radial Hemolysis
trivalent nanoparticle influenza vaccine
Vaccine Effectiveness
World Health Organization
WHO. World Health Organization (WHO) Influenza (Seasonal) 2018 Available from: who.int/en/news-room/fact-sheets/detail/influenza-(seasonal) .
Google Scholar
Iuliano AD, Roguski KM, Chang HH, Muscatello DJ, Palekar R, Tempia S, et al. Estimates of global seasonal influenza-associated respiratory mortality: a modelling study. Lancet. 2018;391(10127):1285–300.
PubMed Google Scholar
Palese P. Influenza: old and new threats. Nat Med. 2004;10:S82.
CAS PubMed Google Scholar
WT RPA, Harmon MW, Rota JS, Kendal AP, Nerome K. Cocirculation of two distinct evolutionary lineages of influenza type B virus since 1983. Virology. 1990;175(1):59–68.
Hause BM, Collin EA, Liu R, Huang B, Sheng Z, Lu W, et al. Characterization of a novel influenza virus in cattle and swine: proposal for a new genus in the Orthomyxoviridae family. MBio. 2014;5(2):e00031–14.
PubMed PubMed Central Google Scholar
Parvin JD, Moscona A, Pan WT, Leider JM, Palese P. Measurement of the mutation rates of animal viruses: influenza a virus and poliovirus type 1. J Virol. 1986;59(2):377–83.
CAS PubMed PubMed Central Google Scholar
Webster RGLW, Air GM, Schild GC. Molecular mechanisms of variation in influenza viruses. Nature. 1982;296(5853):115–21.
Chambers BS, Parkhouse K, Ross TM, Alby K, Hensley SE. Identification of Hemagglutinin residues responsible for H3N2 antigenic drift during the 2014-2015 influenza season. Cell Rep. 2015;12(1):1–6.
Garten RJ, Davis CT, Russell CA, Shu B, Lindstrom S, Balish A, et al. Antigenic and genetic characteristics of swine-origin 2009 a(H1N1) influenza viruses circulating in humans. Science. 2009;325(5937):197–201.
Ferguson NM, Galvani AP, Bush RM. Ecological and immunological determinants of influenza evolution. Nature. 2003;422:428.
Bush RM, Bender CA, Subbarao K, Cox NJ, Fitch WM. Predicting the evolution of human influenza a. Science. 1999;286(5446):1921.
WHO. Global Influenza Surveillance and Response System (GISRS) Available from: https://www.who.int/influenza/gisrs_laboratory/en/ .
Davenport FM. Control of influenza. Med J Aust. 1973;1:33–8.
CDC. Key Facts About Influenza (Flu) 2019 Available from: https://www.cdc.gov/flu/about/keyfacts.htm .
Goodwin K, Viboud C, Simonsen L. Antibody response to influenza vaccination in the elderly: a quantitative review. Vaccine. 2006;24(8):1159–69.
Grohskopf LA, Sokolow LZ, Broder KR, Walter EB, Fry AM, Jernigan DB. Prevention and control of seasonal influenza with vaccines: recommendations of the advisory committee on immunization practices-United States, 2018-19 influenza season. MMWR Recomm Rep. 2018;67(3):1–20.
Ohmit SE, Victor JC, Rotthoff JR, Teich ER, Truscon RK, Baum LL, et al. Prevention of antigenically drifted influenza by inactivated and live attenuated vaccines. N Engl J Med. 2006;355(24):2513–22.
Tisa V, Barberis I, Faccio V, Paganino C, Trucchi C, Martini M, et al. Quadrivalent influenza vaccine: a new opportunity to reduce the influenza burden. J Preventive Med hygiene. 2016;57(1):E28–33.
CAS Google Scholar
Pandey S, Manjrekar S, Sumant O. Influenza Vaccine Market by Vaccine Type (Quadrivalent and Trivalent), Type (Seasonal and Pandemic), Technology (Egg-based and Cell-based), Age Group (Pediatric and Adult), and Route of Administration (Injection and Nasal Spray): Global Opportunity Analysis and Industry Forecast, 2019–2026. Portland: Allied Market Research; 2019. Available from: https://www.alliedmarketresearch.com/influenza-vaccines-market .
McLean KA, Goldin S, Nannei C, Sparrow E, Torelli G. The 2015 global production capacity of seasonal and pandemic influenza vaccine. Vaccine. 2016;34(45):5410–3.
WHO. Addendum to the recommended composition of influenza virus vaccines for use in the 2019–2020 northern hemisphere influenza season 2019. Available from: https://www.who.int/influenza/vaccines/virus/recommendations/201902_recommendation_addendum.pdf?ua=1 . [cited 2019 11/14].
Abelin A, Colegate T, Gardner S, Hehme N, Palache A. Lessons from pandemic influenza a(H1N1): the research-based vaccine industry's perspective. Vaccine. 2011;29(6):1135–8.
Skowronski DM, Janjua NZ, De Serres G, Sabaiduc S, Eshaghi A, Dickinson JA, et al. Low 2012–13 influenza vaccine effectiveness associated with mutation in the egg-adapted H3N2 vaccine strain not antigenic drift in circulating viruses. PLoS One. 2014;9(3):e92153.
Zost SJ, Parkhouse K, Gumina ME, Kim K, Diaz Perez S, Wilson PC, et al. Contemporary H3N2 influenza viruses have a glycosylation site that alters binding of antibodies elicited by egg-adapted vaccine strains. Proc Natl Acad Sci. 2017;114(47):12578.
Widjaja L, Ilyushina N, Webster RG, Webby RJ. Molecular changes associated with adaptation of human influenza a virus in embryonated chicken eggs. Virol. 2006;350(1):137–45.
Nicholls JM, Chan RWY, Russell RJ, Air GM, Peiris JSM. Evolving complexities of influenza virus and its receptors. Trends Microbiol. 2008;16(4):149–57.
Imai M, Kawaoka Y. The role of receptor binding specificity in interspecies transmission of influenza viruses. Curr Opinion Virol. 2012;2(2):160–7.
Ito T, Suzuki Y, Takada A, Kawamoto A, Otsuki K, Masuda H, et al. Differences in sialic acid-galactose linkages in the chicken egg amnion and allantois influence human influenza virus receptor specificity and variant selection. J Virol. 1997;71(4):3357–62.
Hoft DF, Babusis E, Worku S, Spencer CT, Lottenbach K, Truscott SM, et al. Live and inactivated influenza vaccines induce similar humoral responses, but only live vaccines induce diverse T-cell responses in young children. J Infect Dis. 2011;204(6):845–53.
Mohn KG-I, Brokstad KA, Pathirana RD, Bredholt G, Jul-Larsen Å, Trieu MC, et al. Live attenuated influenza vaccine in children induces B-cell responses in tonsils. J Infect Dis. 2016;214(5):722–31.
Gaglani M, Pruszynski J, Murthy K, Clipper L, Robertson A, Reis M, et al. Influenza vaccine effectiveness against 2009 pandemic influenza a(H1N1) virus differed by vaccine type during 2013-2014 in the United States. J Infect Dis. 2016;213(10):1546–56.
Tam TWS. Intranasal influenza vaccine: why does Canada have different recommendations from the USA on its use? Paediatr Child Health. 2018;23(1):31–4.
Singanayagam A, Zambon M, Lalvani A, Barclay W. Urgent challenges in implementing live attenuated influenza vaccine. Lancet Infect Dis. 2018;18(1):e25–32.
Grohskopf LA, Sokolow LZ, Fry AM, Walter EB, Jernigan DB. Update: ACIP recommendations for the use of quadrivalent live attenuated influenza vaccine (LAIV4)—United States, 2018–19 influenza season. Morb Mortal Wkly Rep. 2018;67(22):643.
Immunization NACo. An Advisory Committee Statement (ACS): Canadian Immunization Guide Chapter on Influenza and Statement on Seasonal Influenza Vaccine for 2019-2020. Ottawa: Public Health Agency Of Canada; 2018.
CDC. Flublok Seasonal Influenza (Flu) Vaccine [Available from: https://www.cdc.gov/flu/protect/vaccine/qa_flublok-vaccine.htm .
Cox MMJ, Izikson R, Post P, Dunkle L. Safety, efficacy, and immunogenicity of Flublok in the prevention of seasonal influenza in adults. Therapeutic Advances Vaccin. 2015;3(4):97–108.
Izurieta HS, Chillarige Y, Kelman J, Wei Y, Lu Y, Xu W, et al. Relative effectiveness of cell-cultured and egg-based influenza vaccines among elderly persons in the United States, 2017-2018. J Infect Dis. 2019;220(8):1255–64.
Cox MMJ, Hashimoto Y. A fast track influenza virus vaccine produced in insect cells. J Invertebr Pathol. 2011;107:S31–41.
Dunkle LM, Izikson R, Patriarca P, Goldenthal KL, Muse D, Callahan J, et al. Efficacy of recombinant influenza vaccine in adults 50 years of age or older. N Engl J Med. 2017;376(25):2427–36.
CDC. Archived CDC Vaccine Price List as of 1 September 2019. Available from: https://www.cdc.gov/vaccines/programs/vfc/awardees/vaccine-management/price-list/index.html .
The White House, Office of the Press Secretary. Statement from the Press Secretary on the Executive Order Modernizing Influenza Vaccines in the U.S. to Promote National Security and Public Health [press release] (2019 Sep 19). Available from: https://www.whitehouse.gov/briefings-statements/statement-press-secretary-executive-order-modernizing-influenza-vaccines-u-s-promote-national-security-public-health/ . Cited 2020 Feb 3.
Paules CI, Marston HD, Eisinger RW, Baltimore D, Fauci AS. The pathway to a universal influenza vaccine. Immunity. 2017;47(4):599–603.
Sah P, Alfaro-Murillo JA, Fitzpatrick MC, Neuzil KM, Meyers LA, Singer BH, et al. Future epidemiological and economic impacts of universal influenza vaccines. Proc Natl Acad Sci. 2019;116(41):20786–92.
Smith G, Liu Y, Flyer D, Massare MJ, Zhou B, Patel N, et al. Novel hemagglutinin nanoparticle influenza vaccine with matrix-M adjuvant induces hemagglutination inhibition, neutralizing, and protective responses in ferrets against homologous and drifted a(H3N2) subtypes. Vaccine. 2017;35(40):5366–72.
Shinde V, Fries L, Wu Y, Agrawal S, Cho I, Thomas DN, et al. Improved titers against influenza drift variants with a nanoparticle vaccine. N Engl J Med. 2018;378(24):2346–8.
Lovgren Bengtsson K, Morein B, Osterhaus AD. ISCOM technology-based matrix M adjuvant: success in future vaccines relies on formulation. Expert Rev Vaccines. 2011;10(4):401–3.
Krammer F, Pica N, Hai R, Margine I, Palese P. Chimeric hemagglutinin influenza virus vaccine constructs elicit broadly protective stalk-specific antibodies. J Virol. 2013;87(12):6542–50.
Nachbagauer R, Liu WC, Choi A, Wohlbold TJ, Atlas T, Rajendran M, et al. A universal influenza virus vaccine candidate confers protection against pandemic H1N1 infection in preclinical ferret studies. NPJ Vaccines. 2017;2:26.
Krammer F, Margine I, Hai R, Flood A, Hirsh A, Tsvetnitsky V, et al. H3 stalk-based chimeric hemagglutinin influenza virus constructs protect mice from H7N9 challenge. J Virol. 2014;88(4):2340–3.
Krammer F, Palese P, Steel J. Advances in universal influenza virus vaccine design and antibody mediated therapies based on conserved regions of the hemagglutinin. Curr Top Microbiol Immunol. 2015;386:301–21.
Wang CC, Chen JR, Tseng YC, Hsu CH, Hung YF, Chen SW, et al. Glycans on influenza hemagglutinin affect receptor binding and immune response. Proc Natl Acad Sci U S A. 2009;106(43):18137–42.
Chen JR, Yu YH, Tseng YC, Chiang WL, Chiang MF, Ko YA, et al. Vaccination of monoglycosylated hemagglutinin induces cross-strain protection against influenza virus infections. Proc Natl Acad Sci U S A. 2014;111(7):2476–81.
Tseng YC, Wu CY, Liu ML, Chen TH, Chiang WL, Yu YH, et al. Egg-based influenza split virus vaccine with monoglycosylation induces cross-strain protection against influenza virus infections. Proc Natl Acad Sci U S A. 2019;116(10):4200–5.
Atsmon J, Caraco Y, Ziv-Sefer S, Shaikevich D, Abramov E, Volokhov I, et al. Priming by a novel universal influenza vaccine (Multimeric-001)-a gateway for improving immune response in the elderly population. Vaccine. 2014;32(44):5816–23.
Gottlieb T, Ben-Yedidia T. Epitope-based approaches to a universal influenza vaccine. J Autoimmun. 2014;54:15–20.
Atsmon J, Kate-Ilovitz E, Shaikevich D, Singer Y, Volokhov I, Haim KY, et al. Safety and immunogenicity of multimeric-001--a novel universal influenza vaccine. J Clin Immunol. 2012;32(3):595–603.
van Doorn E, Liu H, Ben-Yedidia T, Hassin S, Visontai I, Norley S, et al. Evaluating the immunogenicity and safety of a BiondVax-developed universal influenza vaccine (Multimeric-001) either as a standalone vaccine or as a primer to H5N1 influenza vaccine: phase IIb study protocol. Medicine (Baltimore). 2017;96(11):e6339.
Stoloff GA, Caparros-Wanderley W. Synthetic multi-epitope peptides identified in silico induce protective immunity against multiple influenza serotypes. Eur J Immunol. 2007;37(9):2441–9.
Pleguezuelos O, Robinson S, Stoloff GA, Caparros-Wanderley W. Synthetic influenza vaccine (FLU-v) stimulates cell mediated immunity in a double-blind, randomised, placebo-controlled phase I trial. Vaccine. 2012;30(31):4655–60.
van Doorn E, Pleguezuelos O, Liu H, Fernandez A, Bannister R, Stoloff G, et al. Evaluation of the immunogenicity and safety of different doses and formulations of a broad spectrum influenza vaccine (FLU-v) developed by SEEK: study protocol for a single-center, randomized, double-blind and placebo-controlled clinical phase IIb trial. BMC Infect Dis. 2017;17(1):241.
Pleguezuelos O, Robinson S, Fernandez A, Stoloff GA, Mann A, Gilbert A, et al. A synthetic influenza virus vaccine induces a cellular immune response that correlates with reduction in symptomatology and virus shedding in a randomized phase Ib live-virus challenge in humans. Clin Vaccine Immunol. 2015;22(7):828–35.
Mitchell CA, Ramessar K, O'Keefe BR. Antiviral lectins: selective inhibitors of viral entry. Antivir Res. 2017;142:37–54.
Mueller S, Coleman JR, Papamichail D, Ward CB, Nimnual A, Futcher B, et al. Live attenuated influenza virus vaccines by computer-aided rational design. Nat Biotechnol. 2010;28(7):723–6.
Yang C, Skiena S, Futcher B, Mueller S, Wimmer E. Deliberate reduction of hemagglutinin and neuraminidase expression of influenza virus leads to an ultraprotective live vaccine in mice. Proc Natl Acad Sci U S A. 2013;110(23):9481–6.
Stauft CB, Yang C, Coleman JR, Boltz D, Chin C, Kushnir A, et al. Live-attenuated H1N1 influenza vaccine candidate displays potent efficacy in mice and ferrets. PLoS One. 2019;14(10):e0223784.
Sarawar S, Hatta Y, Watanabe S, Dias P, Neumann G, Kawaoka Y, et al. M2SR, a novel live single replication influenza virus vaccine, provides effective heterosubtypic protection in mice. Vaccine. 2016;34(42):5090–8.
Hatta Y, Boltz D, Sarawar S, Kawaoka Y, Neumann G, Bilsel P. Novel influenza vaccine M2SR protects against drifted H1N1 and H3N2 influenza virus challenge in ferrets with pre-existing immunity. Vaccine. 2018;36(33):5097–103.
Elliott STC, Keaton AA, Chu JD, Reed CC, Garman B, Patel A, et al. A synthetic micro-consensus DNA vaccine generates comprehensive influenza a H3N2 immunity and protects mice against lethal challenge by multiple H3N2 viruses. Hum Gene Ther. 2018;29(9):1044–55.
Yan J, Morrow MP, Chu JS, Racine T, Reed CC, Khan AS, et al. Broad cross-protective anti-hemagglutination responses elicited by influenza microconsensus DNA vaccine. Vaccine. 2018;36(22):3079–89.
Yan J, Villarreal DO, Racine T, Chu JS, Walters JN, Morrow MP, et al. Protective immunity to H7N9 influenza viruses elicited by synthetic DNA vaccine. Vaccine. 2014;32(24):2833–42.
Choo AY, Broderick KE, Kim JJ, Sardesai NY. DNA-based influenza vaccines: evaluating their potential to provide universal protection. IDrugs. 2010;13(10):707–12.
De Filette M, Martens W, Smet A, Schotsaert M, Birkett A, Londono-Arcila P, et al. Universal influenza a M2e-HBc vaccine protects against disease even in the presence of pre-existing anti-HBc antibodies. Vaccine. 2008;26(51):6503–7.
Deng L, Cho KJ, Fiers W, Saelens X. M2e-based universal influenza a vaccines. Vaccines (Basel). 2015;3(1):105–36.
O'Donnell CD, Wright A, Vogel LN, Wei CJ, Nabel GJ, Subbarao K. Effect of priming with H1N1 influenza viruses of variable antigenic distances on challenge with 2009 pandemic H1N1 virus. J Virol. 2012;86(16):8625–33.
Tate MD, Job ER, Deng YM, Gunalan V, Maurer-Stroh S, Reading PC. Playing hide and seek: how glycosylation of the influenza virus hemagglutinin can modulate the immune response to infection. Viruses. 2014;6(3):1294–316.
Medina RA, Stertz S, Manicassamy B, Zimmermann P, Sun X, Albrecht RA, et al. Glycosylations in the globular head of the hemagglutinin protein modulate the virulence and antigenic properties of the H1N1 influenza viruses. Sci Transl Med. 2013;5(187):187ra70.
Kobayashi Y, Suzuki Y. Evidence for N-glycan shielding of antigenic sites during evolution of human influenza a virus hemagglutinin. J Virol. 2012;86(7):3446–51.
Wei CJ, Boyington JC, Dai K, Houser KV, Pearce MB, Kong WP, et al. Cross-neutralization of 1918 and 2009 influenza viruses: role of glycans in viral evolution and vaccine design. Sci Transl Med. 2010;2(24):24ra1.
Abe Y, Takashita E, Sugawara K, Matsuzaki Y, Muraki Y, Hongo S. Effect of the addition of oligosaccharides on the biological activities and antigenicity of influenza a/H3N2 virus Hemagglutinin. J Virol. 2004;78(18):9605–11.
Chen JR, Ma C, Wong CH. Vaccine design of hemagglutinin glycoprotein against influenza. Trends Biotechnol. 2011;29(9):426–34.
Zhou T, Doria-Rose NA, Cheng C, Stewart-Jones GBE, Chuang GY, Chambers M, et al. Quantification of the impact of the HIV-1-glycan shield on antibody elicitation. Cell Rep. 2017;19(4):719–32.
Hurtley SM, Bole DG, Hoover-Litty H, Helenius A, Copeland CS. Interactions of misfolded influenza virus hemagglutinin with binding protein (BiP). J Cell Biol. 1989;108(6):2117–26.
Roberts PC, Garten W, Klenk HD. Role of conserved glycosylation sites in maturation and transport of influenza a virus hemagglutinin. J Virol. 1993;67(6):3048–60.
Lowell GH, Ziv S, Bruzil S, Babecoff R, Ben-Yedidia T. Back to the future: immunization with M-001 prior to trivalent influenza vaccine in 2011/12 enhanced protective immune responses against 2014/15 epidemic strain. Vaccine. 2017;35(5):713–5.
Saelens X. The Role of Matrix Protein 2 Ectodomain in the Development of Universal Influenza Vaccines. J Infect Dis. 2019;219(Supplement 1):S68–74.
Erbelding EJ, Post DJ, Stemmy EJ, Roberts PC, Augustine AD, Ferguson S, et al. A universal influenza vaccine: the strategic plan for the National Institute of Allergy and Infectious Diseases. J Infect Dis. 2018;218(3):347–54.
Wijnans L, Voordouw B. A review of the changes to the licensing of influenza vaccines in Europe. Influenza Other Respir Viruses. 2016;10(1):2–8.
Weir JP, Gruber MF. An overview of the regulation of influenza vaccines in the United States. Influenza Other Respir Viruses. 2016;10(5):354–60.
Schild GC, Pereira MS, Chakraverty P. Single-radial-hemolysis: a new method for the assay of antibody to influenza haemagglutinin. Applications for diagnosis and seroepidemiologic surveillance of influenza. Bull World Health Organ. 1975;52(1):43–50.
Gianchecchi E, Torelli A, Montomoli E. The use of cell-mediated immunity for the evaluation of influenza vaccines: an upcoming necessity. Human Vaccin Immunotherapeutics. 2019;15(5):1021–30.
Oxford JS, Oxford JR. Clinical, scientific and ethnographic studies of influenza in quarantine. Expert Rev Vaccin. 2012;11(8):929–37.
Oxford JS, Gelder C, Lambkin R. Would you volunteer to be quarantined and infected with influenza virus? Expert Rev Anti-Infect Ther. 2005;3(1):1–2.
Wilkinson TM, Li CK, Chui CS, Huang AK, Perkins M, Liebner JC, et al. Preexisting influenza-specific CD4+ T cells correlate with disease protection against influenza challenge in humans. Nat Med. 2012;18(2):274–80.
Govaert TM, Thijs CT, Masurel N, Sprenger MJ, Dinant GJ, Knottnerus JA. The efficacy of influenza vaccination in elderly individuals. A randomized double-blind placebo-controlled trial. JAMA. 1994;272(21):1661–5.
Petrie JG, Ohmit SE, Truscon R, Johnson E, Braun TM, Levine MZ, et al. Modest waning of influenza vaccine efficacy and antibody titers during the 2007-2008 influenza season. J Infect Dis. 2016;214(8):1142–9.
Ferdinands JM, Fry AM, Reynolds S, Petrie JG, Flannery B, Jackson ML, et al. Intraseason waning of influenza vaccine protection: evidence from the US influenza vaccine effectiveness network, 2011–2012 through 2014–2015. Clin Infect Dis. 2017;64(5):544–50.
Organization WH. WHO preferred product characteristics for next generation influenza vaccines. 2017.
Chai N, Swem LR, Reichelt M, Chen-Harris H, Luis E, Park S, et al. Two escape mechanisms of influenza a virus to a broadly neutralizing stalk-binding antibody. PLoS Pathog. 2016;12(6):e1005702.
Doud MB, Lee JM, Bloom JD. How single mutations affect viral escape from broad and narrow antibodies to H1 influenza hemagglutinin. Nat Commun. 2018;9(1):1386.
Marcelin G, Sandbulte MR, Webby RJ. Contribution of antibody production against neuraminidase to the protection afforded by influenza vaccines. Rev Med Virol. 2012;22(4):267–79.
Download references
Acknowledgements
Funding was provided by Academia Sinica Genomics Research Center Summit Project AS-SUMMIT-108.
Author information
Juine-Ruey Chen and Yo-Min Liu contributed equally to this work.
Authors and Affiliations
RuenHuei Biopharmaceuticals, Inc., Taipei, 100, Taiwan
Juine-Ruey Chen
Genomics Research Center, Academia Sinica, Taipei, 115, Taiwan
Yo-Min Liu, Yung-Chieh Tseng & Che Ma
Institute of Microbiology and Immunology, National Yang Ming University, Taipei, 112, Taiwan
You can also search for this author in PubMed Google Scholar
Contributions
JRC, YML, Y-CT, and CM wrote the manuscript. JRC and YCT designed and illustrated table and figures. All authors read and approved the final manuscript.
Corresponding author
Correspondence to Che Ma .
Ethics declarations
Ethics approval and consent to participate, consent for publication, competing interests.
Patent applications of monoglycosylated influenza vaccines have been submitted by Academia Sinica with C.M., J.R.C. and Y.C.T. as inventors. RuenHuei Biopharmaceuticals, Inc. is in co-development with OPKO Health, Inc. on the monoglycosylated influenza vaccine technology licensed by Academia Sinica.
Additional information
Publisher’s note.
Springer Nature remains neutral with regard to jurisdictional claims in published maps and institutional affiliations.
Rights and permissions
Open Access This article is distributed under the terms of the Creative Commons Attribution 4.0 International License ( http://creativecommons.org/licenses/by/4.0/ ), which permits unrestricted use, distribution, and reproduction in any medium, provided you give appropriate credit to the original author(s) and the source, provide a link to the Creative Commons license, and indicate if changes were made. The Creative Commons Public Domain Dedication waiver ( http://creativecommons.org/publicdomain/zero/1.0/ ) applies to the data made available in this article, unless otherwise stated.
Reprints and permissions
About this article
Cite this article.
Chen, JR., Liu, YM., Tseng, YC. et al. Better influenza vaccines: an industry perspective. J Biomed Sci 27 , 33 (2020). https://doi.org/10.1186/s12929-020-0626-6
Download citation
Received : 05 December 2019
Accepted : 23 January 2020
Published : 14 February 2020
DOI : https://doi.org/10.1186/s12929-020-0626-6
Share this article
Anyone you share the following link with will be able to read this content:
Sorry, a shareable link is not currently available for this article.
Provided by the Springer Nature SharedIt content-sharing initiative
- Influenza virus
- Universal vaccine
- Monoglycosylated HA
- Monoglycosylated split vaccine
Journal of Biomedical Science
ISSN: 1423-0127
- Submission enquiries: Access here and click Contact Us
- General enquiries: [email protected]
April 15, 2024
COVID, Flu and RSV Vaccines Are Lifesavers. Why Aren’t More Older Adults Getting Them?
We need to do more to ensure older adults—including those living in long-term care facilities—are up to date on recommended vaccines
By Sarah Meyer & Georgina Peacock
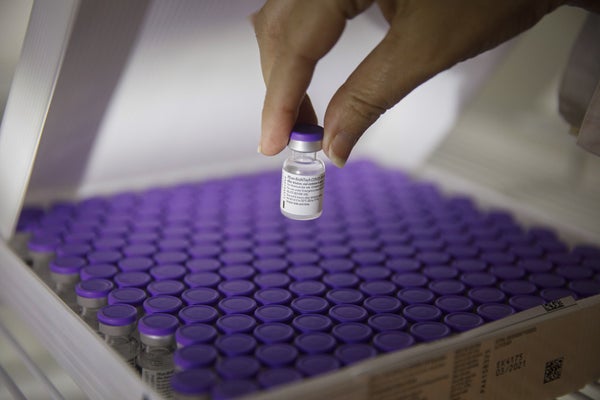
Kobi Wolf/Bloomberg via Getty Images
On supporting science journalism
If you're enjoying this article, consider supporting our award-winning journalism by subscribing . By purchasing a subscription you are helping to ensure the future of impactful stories about the discoveries and ideas shaping our world today.
For the first time, we have vaccines that can protect older adults against three leading—and sometimes fatal—respiratory viral diseases: influenza, COVID and respiratory syncytial virus (RSV). This is a breakthrough; studies show that these vaccines are effective at protecting older adults from severe disease outcomes, including hospitalization and death. Yet some seniors—including many who live in nursing homes—aren’t getting these vaccines. And the prevaccine days of the COVID pandemic showed us how deadly respiratory illness could be among older people in group settings. As scientists at the Centers for Disease Control and Prevention, we have been tracking vaccination rates among older people. Given how easily these diseases are spread, and the possibility of severe disease with long and complex hospitalizations, we must do more to help inoculate them. As this winter respiratory virus season winds down, it’s crucial we start planning for the next one. We can use the lessons we’ve learned from the vaccine rollouts for flu, COVID and RSV to give seniors the best shot at protection. Older adults have a higher risk of severe disease and death from these respiratory virus infections compared to other age groups. Both their first-line innate immune responses and their slower, infection-specific adaptive immune responses decline. This decline, combined with higher rates of chronic diseases such as heart disease and diabetes and—for people who live in long-term care facilities—an increased chance of disease spread, leaves older folks at risk for severe disease and death. Improving the use of these vaccines through the fall and winter respiratory illness seasons could mean healthier seniors and fewer visits to urgent care and the emergency department and fewer hospitalizations. In 2022–2023, experts estimate that flu vaccination prevented nearly 31,000 hospitalizations and 2,500 deaths among people ages 65 and older. COVID vaccination greatly lowered rates of hospitalizations and deaths among adults ages 65 years and older too. And in clinical trials, the new RSV vaccines had an efficacy of 83 to 89 percent in preventing symptomatic RSV in the lower respiratory tract in adults ages 60 years and older. The CDC’s latest data show that as of late March, 74 percent of adults age 65 years and older had gotten the flu vaccine and just 42 percent had received the updated COVID vaccine. Although coverage for influenza vaccine is trending slightly higher than at this point last year, COVID vaccine coverage remains about as low as last year. Among those ages 60 years and older, 24 percent had gotten an RSV vaccine. As of late March, only 43 percent of nursing home residents had received an updated COVID vaccine; as of December 10, 72 percent had received an influenza vaccine and 10 percent had received an RSV vaccine. The fact that nearly three quarters of older adults received a flu vaccine this season, as opposed to less than half for the COVID vaccine, shows us that we have a lot of work to do to help people get up-to-date on COVID vaccines. And there is more to be done to help people and their providers understand whether an RSV vaccine is right for them. The CDC surveyed unvaccinated older folks to better understand their reasons for not getting vaccinated, and the results varied. People 65 and older who said they were probably or definitely not going to get the influenza vaccine were concerned primarily about vaccine effectiveness and side effects and said they were not worried about the flu. For the COVID vaccine, participants most often shared concerns about heart-related or unknown serious side effects, followed by concerns about effectiveness and having “vaccine fatigue,” meaning they were likely burned out on vaccine information. The primary reasons for people age 60 and older not getting the RSV vaccine were not being worried about RSV, not knowing enough about RSV or the RSV vaccine, and the vaccine being “too new.” These reasons for not getting vaccinated and the differences across vaccines are perhaps understandable in the context of where we are in the vaccine rollouts. Influenza vaccines have been licensed in the U.S. since the 1940s. In contrast, COVID vaccines were introduced little more than three years ago, and while these vaccines have undergone the most rigorous safety monitoring in U.S. history, some people still have misconceptions about the vaccines’ safety. In addition, the vaccine fatigue expressed by respondents to the CDC survey is a genuine challenge. In the early days of COVID vaccines, older adults enthusiastically accepted vaccination. But over time, fewer and fewer seniors have been willing to get additional recommended doses. Many people are also less concerned about COVID itself, despite the fact that many people are still dying from it each day in the U.S. RSV vaccines were licensed in 2023—and from prior new vaccine rollouts we know that it can take years for vaccination coverage to increase. Moreover, instead of recommending that all adults 60 years and older get vaccinated, the CDC recommended that people and their health care providers have a conversation to determine if RSV vaccination is right for them. As a result, not all eligible adults are likely to get the vaccine. Plus it is hard for some people to access vaccines. On one hand there is ample supply of all three vaccines, and they are covered by Medicare and many private insurance plans at no out-of-pocket cost. Still, there are around 400,000 people age 65 and older who are uninsured. Nonetheless, the health care provider or facility has to absorb the up-front costs of purchasing vaccines and then seek reimbursement for vaccination. Furthermore, disparities in access to health care among ethnic and racial groups make getting respiratory vaccines challenging for some communities in the U.S. For example, during the 2022–2023 season, influenza vaccination coverage among adults ages 65 years and older ranged from 54 percent in American Indian/Alaska Native people to 71 percent in non-Hispanic white people. There also are barriers to receiving these three vaccines within a relatively short period of time. Even though the CDC says that influenza, COVID and RSV vaccines can be given at the same time, not everyone is open to that. Furthermore, each of these vaccines became available at different points in time during this past season, which made it more difficult for people to receive these vaccines at once. For some, it may not have been a priority or a possibility to return for a follow-up visit for additional vaccines that they were unable to receive on prior visits. Long-term care facilities face additional unique challenges to vaccinating residents, including the monumental task of strengthening vaccine confidence and demand not only among residents but also among staff at the facilities and family members involved in residents’ medical decisions. In addition, long-term care facilities may not have the necessary infrastructure, staffing and financial resources to routinely offer vaccines to residents. Furthermore, the end of the Public Health Emergency and transition from a federal COVID vaccine distribution system to a commercialized market ended certain regulatory flexibilities and continued the shift to more sustainable channels for vaccinating residents, though with fewer dedicated resources. The relatively high influenza vaccination coverage among older adults suggests that it is possible to get more older people vaccinated for all recommended vaccines. The CDC is working to improve access to adult vaccines through programs such as the Bridge Access Program, which provides COVID vaccines at no cost to uninsured or underinsured adults. The CDC is working to strengthen confidence in and demand for vaccines; to communicate the benefits of vaccination to the public, and to use data to target vaccination efforts. In addition, because a strong recommendation from a health care provider remains the leading reason why people choose to get vaccinated, the CDC has worked to equip providers with resources on vaccine recommendations and on having effective conversations with patients about vaccines. It will take ground-up efforts across every community, vaccination provider location and household around the U.S. to ensure that older adults get not only the respiratory virus vaccines but all recommended vaccines.
The views expressed in this article do not necessarily represent those of the Centers for Disease Control and Prevention.
This is an opinion and analysis article, and the views expressed by the author or authors are not necessarily those of Scientific American .
- Alzheimer's disease & dementia
- Arthritis & Rheumatism
- Attention deficit disorders
- Autism spectrum disorders
- Biomedical technology
- Diseases, Conditions, Syndromes
- Endocrinology & Metabolism
- Gastroenterology
- Gerontology & Geriatrics
- Health informatics
- Inflammatory disorders
- Medical economics
- Medical research
- Medications
- Neuroscience
- Obstetrics & gynaecology
- Oncology & Cancer
- Ophthalmology
- Overweight & Obesity
- Parkinson's & Movement disorders
- Psychology & Psychiatry
- Radiology & Imaging
- Sleep disorders
- Sports medicine & Kinesiology
- Vaccination
- Breast cancer
- Cardiovascular disease
- Chronic obstructive pulmonary disease
- Colon cancer
- Coronary artery disease
- Heart attack
- Heart disease
- High blood pressure
- Kidney disease
- Lung cancer
- Multiple sclerosis
- Myocardial infarction
- Ovarian cancer
- Post traumatic stress disorder
- Rheumatoid arthritis
- Schizophrenia
- Skin cancer
- Type 2 diabetes
- Full List »
share this!
April 15, 2024
This article has been reviewed according to Science X's editorial process and policies . Editors have highlighted the following attributes while ensuring the content's credibility:
fact-checked
peer-reviewed publication
trusted source
New vaccine strategy may mean the end of the line for endless boosters
by University of California - Riverside
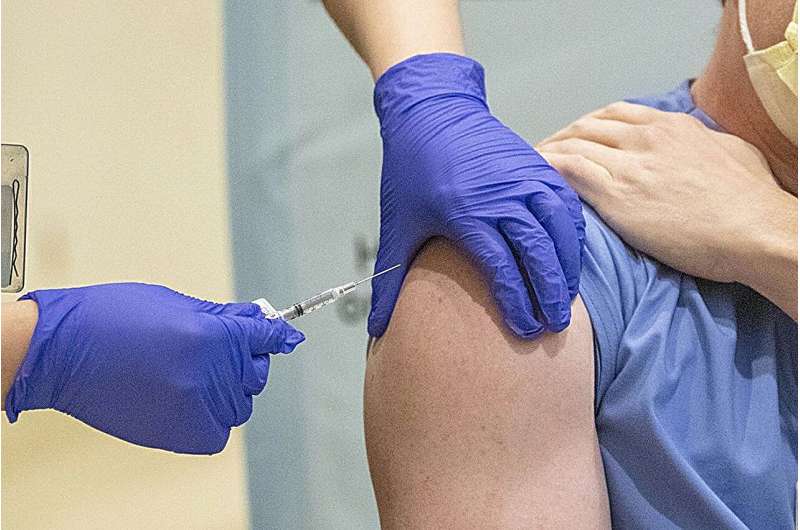
Scientists at UC Riverside have demonstrated a new, RNA-based vaccine strategy that is effective against any strain of a virus and can be used safely even by babies or the immunocompromised.
Every year, researchers try to predict the four influenza strains that are most likely to be prevalent during the upcoming flu season. And every year, people line up to get their updated vaccine, hoping the researchers formulated the shot correctly.
The same is true of COVID vaccines, which have been reformulated to target sub-variants of the most prevalent strains circulating in the U.S.
This new strategy would eliminate the need to create all these different shots because it targets a part of the viral genome that is common to all strains of a virus. The vaccine, how it works, and a demonstration of its efficacy in mice is described in a paper published in the Proceedings of the National Academy of Sciences .
"What I want to emphasize about this vaccine strategy is that it is broad," said UCR virologist and paper author Rong Hai. "It is broadly applicable to any number of viruses, broadly effective against any variant of a virus, and safe for a broad spectrum of people. This could be the universal vaccine that we have been looking for."
Traditionally, vaccines contain either a dead or modified, live version of a virus. The body's immune system recognizes a protein in the virus and mounts an immune response. This response produces T-cells that attack the virus and stop it from spreading. It also produces "memory" B-cells that train your immune system to protect you from future attacks.
The new vaccine also uses a live, modified version of a virus. However, it does not rely on the vaccinated body having this traditional immune response or immune active proteins—which is the reason it can be used by babies whose immune systems are underdeveloped, or people suffering from a disease that overtaxes their immune system. Instead, this relies on small, silencing RNA molecules.
"A host—a person, a mouse, anyone infected—will produce small interfering RNAs as an immune response to viral infection. These RNAi then knock down the virus," said Shouwei Ding, distinguished professor of microbiology at UCR, and lead paper author.
The reason viruses successfully cause disease is because they produce proteins that block a host's RNAi response. "If we make a mutant virus that cannot produce the protein to suppress our RNAi, we can weaken the virus. It can replicate to some level, but then loses the battle to the host RNAi response," Ding said. "A virus weakened in this way can be used as a vaccine for boosting our RNAi immune system."
When the researchers tested this strategy with a mouse virus called Nodamura, they did it with mutant mice lacking T and B cells. With one vaccine injection, they found the mice were protected from a lethal dose of the unmodified virus for at least 90 days. Note that some studies show nine mouse days are roughly equivalent to one human year.
There are few vaccines suitable for use in babies younger than six months old. However, even newborn mice produce small RNAi molecules, which is why the vaccine protected them as well. UC Riverside has now been issued a US patent on this RNAi vaccine technology.
In 2013, the same research team published a paper showing that flu infections also induce us to produce RNAi molecules. "That's why our next step is to use this same concept to generate a flu vaccine , so infants can be protected. If we are successful, they'll no longer have to depend on their mothers' antibodies," Ding said.
Their flu vaccine will also likely be delivered in the form of a spray, as many people have an aversion to needles. "Respiratory infections move through the nose, so a spray might be an easier delivery system," Hai said.
Additionally, the researchers say there is little chance of a virus mutating to avoid this vaccination strategy. "Viruses may mutate in regions not targeted by traditional vaccines. However, we are targeting their whole genome with thousands of small RNAs. They cannot escape this," Hai said.
Ultimately, the researchers believe they can 'cut and paste' this strategy to make a one-and-done vaccine for any number of viruses.
"There are several well-known human pathogens; dengue, SARS, COVID. They all have similar viral functions," Ding said. "This should be applicable to these viruses in an easy transfer of knowledge."
Explore further
Feedback to editors

Health improvements occurred worldwide since 2010 despite COVID-19 pandemic, but progress was uneven: Study
27 minutes ago

Study shows heart failure, not stroke is the most common complication of atrial fibrillation

Antipsychotics for dementia linked to more harms than previously acknowledged

Protecting brain cells with cannabinol: Research suggests CBN shows promise for treating neurological disorders

Calorie restriction study reveals complexities in how diet impacts aging

Ketamine produces wide variety of responses in the brain, researchers find
2 hours ago

Emotional radio ads may ease listeners' qualms, boosting support for organ donation

To understand cognition and its dysfunction, neuroscientists must learn its rhythms

New insights into the mechanisms of bacterial brain invasion during meningitis

Study finds lower relapse risk in triple-negative breast cancer with high immune cell levels
Related stories.

Researchers develop a nasal vaccine that prevents COVID-19 in preclinical studies
Nov 6, 2023

Single vaccine protects against three deadly strains of coronavirus
Oct 18, 2023

Measles-based vector vaccine protects mice against influenza A (H7N9) virus
Jul 7, 2023

New flu vaccine uses mRNA to target four viral proteins that change little between strains
Nov 1, 2022

Different types of COVID-19 vaccines: How they work
Feb 12, 2021

Universal flu vaccine with nanoparticles that protects against six different influenza viruses in mice
Jan 7, 2020
Recommended for you

Study links lack of diversity in Alzheimer's disease clinical trials to differences in amyloid levels
3 hours ago

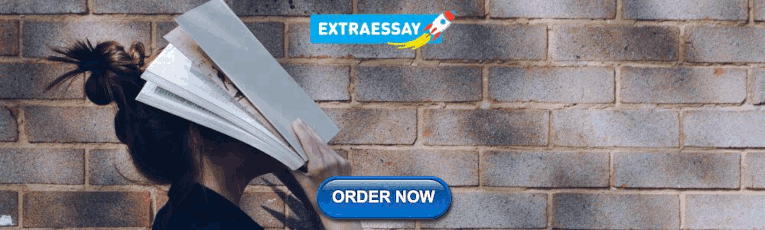
Researchers find glucose levels of nondiabetic people vary more than thought
8 hours ago

Mouse study shows how a father's diet can shape the health of his offspring
9 hours ago

Researchers find ethnic minorities are underrepresented in studies into multiple long-term health conditions
23 hours ago

Study on rats shows a junk food diet can cause long-term damage to adolescent brains
Apr 16, 2024

New treatment method using plasma irradiation promotes faster bone healing
Let us know if there is a problem with our content.
Use this form if you have come across a typo, inaccuracy or would like to send an edit request for the content on this page. For general inquiries, please use our contact form . For general feedback, use the public comments section below (please adhere to guidelines ).
Please select the most appropriate category to facilitate processing of your request
Thank you for taking time to provide your feedback to the editors.
Your feedback is important to us. However, we do not guarantee individual replies due to the high volume of messages.
E-mail the story
Your email address is used only to let the recipient know who sent the email. Neither your address nor the recipient's address will be used for any other purpose. The information you enter will appear in your e-mail message and is not retained by Medical Xpress in any form.
Newsletter sign up
Get weekly and/or daily updates delivered to your inbox. You can unsubscribe at any time and we'll never share your details to third parties.
More information Privacy policy
Donate and enjoy an ad-free experience
We keep our content available to everyone. Consider supporting Science X's mission by getting a premium account.
E-mail newsletter
'One and Done': Scientists Develop Vaccine That May Fight Any Viral Strain
'One and Done': Scientists Develop Vaccine That May Fight Any Viral Strain
By Dennis Thompson HealthDay Reporter

TUESDAY, April 16, 2024 (HealthDay News) -- Genetics-based “one-and-done” vaccines for the flu and COVID could prove more effective and easier to craft than current jabs, researchers report.
These new vaccines would target viruses using a different response to infection than what is prompted by current vaccines, researchers said.
Instead of teaching the immune system to create antibodies to fight off a specific virus, the new vaccine would instead teach the body to create small signaling RNA proteins that will shut down harmful viral spread.
This new approach could revolutionize current vaccine development, in which experts create vaccines based on predictions of which flu and COVID strains are likely to be most infectious, researchers argue.
U.S. Cities With the Most Homelessness

Instead, these RNA-promoting vaccines should be effective against all strains of a specific virus.
“What I want to emphasize about this vaccine strategy is that it is broad,” said researcher Rong Hai , a virologist with University of California, Riverside. “It is broadly applicable to any number of viruses, broadly effective against any variant of a virus and safe for a broad spectrum of people.”
“This could be the universal vaccine that we have been looking for,” Hai added in a university news release.
Traditionally, vaccines use dead or live but modified virus to prompt an immune response from the body. This immune response teaches the body how to recognize a germ and produce antibodies that specifically target and kill that bug.
The new vaccine also uses a live, modified version of a virus, but it doesn’t rely on the body having a traditional immune response that prompts the creation of antibodies, researchers said.
Instead, these vaccines teach the body to interfere with viral replication through the use of targeted RNA signaling proteins.
Everyone already has this natural response to infection, but viruses can produce their own proteins that block the body’s RNA response, explained researcher Showei Ding , a professor of microbiology at University of California, Riverside. The new vaccine teaches the body how to get around this viral defense.
There’s also little chance a virus could mutate to avoid this vaccine, Hia added.
“Viruses may mutate in regions not targeted by traditional vaccines. However, we are targeting their whole genome with thousands of small RNAs. They cannot escape this,” Hai said.
One such vaccine injection created for a mouse virus called Nodamura protected lab mice from lethal doses of the virus for at least 90 days, researchers report. Nine mouse days are roughly equivalent to one human year.
The new study was published April 15 in the Proceedings of the National Academy of Sciences .
University of California, Riverside, has been issued a U.S. patent on its new RNA vaccine technology, the researchers said.
The vaccine will be of particular help to newborns, who can’t receive current vaccines because their immune systems are underdeveloped, as well as people with diseases that compromise their immune systems, researchers said.
“That’s why our next step is to use this same concept to generate a flu vaccine, so infants can be protected,” Ding said. “If we are successful, they’ll no longer have to depend on their mothers’ antibodies.”
The new vaccine could also be delivered in the form of a spray, which will help people who are needle-shy.
“Respiratory infections move through the nose, so a spray might be an easier delivery system,” Hai said.
Ultimately, this strategy could be used to “cut and paste” vaccines for any number of viruses, the researchers noted.
“There are several well-known human pathogens; dengue, SARS, COVID. They all have similar viral functions,” Ding said. “This should be applicable to these viruses in an easy transfer of knowledge.”
More information
The U.S. Centers for Disease Control and Prevention has more on how traditional vaccines work .
SOURCE: University of California, Riverside, news release, April 15, 2024
Copyright © 2024 HealthDay . All rights reserved.
Join the Conversation
Tags: vaccines
America 2024

Health News Bulletin
Stay informed on the latest news on health and COVID-19 from the editors at U.S. News & World Report.
Sign in to manage your newsletters »
Sign up to receive the latest updates from U.S News & World Report and our trusted partners and sponsors. By clicking submit, you are agreeing to our Terms and Conditions & Privacy Policy .
You May Also Like
The 10 worst presidents.
U.S. News Staff Feb. 23, 2024

Cartoons on President Donald Trump
Feb. 1, 2017, at 1:24 p.m.

Photos: Obama Behind the Scenes
April 8, 2022

Photos: Who Supports Joe Biden?
March 11, 2020
Boeing Called Out for ‘Defective’ Planes
Laura Mannweiler April 17, 2024

Senate Kills Mayorkas Impeachment
Aneeta Mathur-Ashton April 17, 2024

Fed: Strong Economy Stalling Rate Cuts
Tim Smart April 17, 2024

The Implications of Trump Legal Wins
Lauren Camera April 16, 2024

The Week in Cartoons April 15-19
April 17, 2024, at 3:38 p.m.

Justices Weigh Jan. 6 Obstruction Charge

New ‘One-And-Done’ Vaccine Method Could Protect Infants—From Covid, Flu—With Just A Single Shot, Study Suggests
- Share to Facebook
- Share to Twitter
- Share to Linkedin
Researchers are pitching a new vaccine method for infants that offers continued protection with just a single dose, even if the virus mutates, according to a new study that could set the stage for “universal vaccines.”
A patient getting vaccine.
Vaccines for diseases like the flu are updated annually to accommodate for new variants, while vaccines for some diseases like COVID-19 are updated less frequently to target dominant variants of the virus circulating in the U.S.
There are different ways to create vaccines, but one of the most popular ways to make them is by including a weakened or inactive version of the virus, which in turn causes the body’s immune system to produce T-cells that attack the virus and prevent it from spreading.
This new vaccine strategy—tested on mice—also uses a modified version of a virus, but instead of relying on the body’s usual immune system response, it uses small interfering RNA molecules ( siRNA ), which stop the spread of disease, to create separate vaccines that target different diseases, according to a study published Monday in the Proceedings of the National Academy of Sciences.
Diseases thrive because they produce a protein that can block the production of siRNAs, but the new vaccine strategy creates and uses a mutant virus that can’t produce these proteins, which allows the body’s siRNAs to weaken the virus, regardless of if it mutates and makes a new variant.
The research team from the University of California, Riverside, believes because this strategy doesn’t rely on the body’s immune response to disease, it will also be suitable for babies, whose immune systems are still developing.
The researchers tested this method in baby mice and discovered they also produce siRNA, so they vaccinated them against a mouse disease called Nodamura, and they found the vaccine induced “rapid and complete protection” against the virus.
Crucial Quote
“It is broadly applicable to any number of viruses, broadly effective against any variant of a virus and safe for a broad spectrum of people,” Rong Hai, a study author and virologist at the University of California, Riverside, said in a statement. “This could be the universal vaccine that we have been looking for.”
Key Background
Though there are some approved vaccines for infants, vaccines for diseases like measles , COVID-19 and the flu can only be administered to people over the age of six months. This is because of their immature immune systems that cause a diminished response to these vaccines, and a potential lack of effectiveness, according to a study published in Vaccine. However, this group of people is one of the most susceptible to severe infection. Infants younger than six months have the highest risk of being hospitalized due to flu infection, according to the Centers for Disease Control and Prevention. In order to protect this population from infection, it’s recommended that all household members and people who come into close contact with them get vaccinated from these diseases. Some research shows vaccinated mothers may pass antibodies to fetuses through the umbilical cord, and newborns via breast milk that can continue to protect the babies from infection. Respiratory syncytial virus (RSV) vaccines given to pregnant mothers have proven to protect infants up to six months after birth, according to the CDC.
Though there aren’t any approved siRNA vaccines, researchers are working on ones that target COVID-19 and the flu. It was previously debated whether humans and other mammals use interfering RNA to kill viruses. However, the same team from the University of California, Riverside also conducted research in 2013 that discovered this theory was true. The team later went on to prove flu infection causes the body to produce interfering RNA, so they’re in the process of developing a flu vaccine that uses this strategy.
Surprising Fact
The researchers intend on creating this vaccine as a nasal spray rather than a shot. “Respiratory infections move through the nose, so a spray might be an easier delivery system,” Hai said. There’s already an approved nasal spray flu vaccine that’s shown to be as effective in children as flu shots, according to the CDC. Several research teams are working on nasal COVID-19 vaccines, and both China and India have approved the use of nasal sprays in the form of boosters.
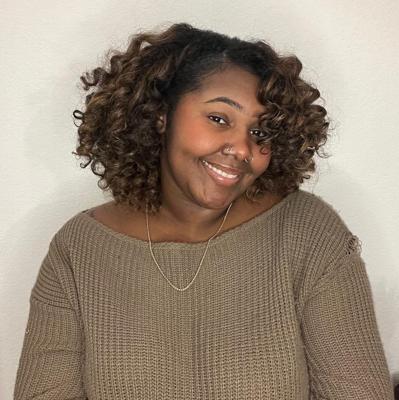
- Editorial Standards
- Reprints & Permissions
ORIGINAL RESEARCH article
Non-cross-reactive epitopes dominate the humoral immune response to covid-19 vaccination -kinetics of plasma antibodies, plasmablasts and memory b cells.
- 1 Institute of Immunology, University Medicine Greifswald, Greifswald, Germany
- 2 Institute for Infection Medicine, Kiel University and University Medical Center Schleswig-Holstein, Kiel, Germany
- 3 Department of Functional Genomics, Interfaculty Institute for Genetics and Functional Genomics, University Medicine Greifswald, Greifswald, Mecklenburg-Vorpommern, Germany
- 4 Friedrich Loeffler Institute of Medical Microbiology, University Medicine Greifswald, Greifswald, Mecklenburg-Vorpommern, Germany
- 5 Institute for Educational Quality Improvement (IQB), Berlin, Berlin, Germany
The final, formatted version of the article will be published soon.
Select one of your emails
You have multiple emails registered with Frontiers:
Notify me on publication
Please enter your email address:
If you already have an account, please login
You don't have a Frontiers account ? You can register here
COVID-19 vaccines are highly effective in inducing protective immunity. While the serum antibody response to COVID-19 vaccination has been studied in depth, our knowledge about the underlying plasmablast and memory B cell (Bmem) responses is still incomplete. Here, we determined the antibody and B cell response to COVID-19 vaccination in a naïve population and contrasted it with the response to a single influenza vaccination in a primed cohort. In addition, we analyzed the antibody and B cell responses against the four endemic human coronaviruses (HCoVs). Measurement of specific plasma IgG antibodies was combined with functional analyses of antibody-secreting plasmablasts and Bmems. The antibody and B cell responses to COVID-19 vaccination reflected the kinetics of a prime-boost immunization, characterized by a slow and moderate primary response and a faster and stronger secondary response. In contrast, the influenza vaccinees possessed robust immune memory for the vaccine antigens prior to vaccination, and the recall vaccination moderately boosted antibody production and Bmem responses. Antibody levels and Bmem responses waned several months after the 2nd COVID-19 vaccination, but were restored upon the 3rd vaccination. The COVID-19 vaccine-induced antibodies mainly targeted novel, non-cross-reactive S1 epitopes, while cross-reactive S2 epitopes were less immunogenic. Booster vaccination not only strongly enhanced neutralizing antibodies against an original SARS-CoV-2 strain, but also induced neutralizing antibodies against the omicron BA.2 variant. We observed a 100% seroprevalence against the S1 subunits of HCoVs, which was not affected by vaccination. Overall, by complementing classical serology with a functional evaluation of plasmablasts and memory B cells we provide new insights into the specificity of COVID-19 vaccine-induced antibody and B cell responses.
Keywords: COVID-19, Vaccination, antibody, plasmablast, memory B cell, HCoV, influenza, Original Antigenic Sin
Received: 06 Feb 2024; Accepted: 15 Apr 2024.
Copyright: © 2024 Wietschel, Fechtner, Antileo, Abdurrahman, Drechsler, Makuvise, Rose, Voß, Krumbholz, Michalik, Weiss, Ulm, Franikowski, Fickenscher, Bröker, Raafat and Holtfreter. This is an open-access article distributed under the terms of the Creative Commons Attribution License (CC BY) . The use, distribution or reproduction in other forums is permitted, provided the original author(s) or licensor are credited and that the original publication in this journal is cited, in accordance with accepted academic practice. No use, distribution or reproduction is permitted which does not comply with these terms.
* Correspondence: Silva Holtfreter, Institute of Immunology, University Medicine Greifswald, Greifswald, Germany
Disclaimer: All claims expressed in this article are solely those of the authors and do not necessarily represent those of their affiliated organizations, or those of the publisher, the editors and the reviewers. Any product that may be evaluated in this article or claim that may be made by its manufacturer is not guaranteed or endorsed by the publisher.

An official website of the United States government
The .gov means it’s official. Federal government websites often end in .gov or .mil. Before sharing sensitive information, make sure you’re on a federal government site.
The site is secure. The https:// ensures that you are connecting to the official website and that any information you provide is encrypted and transmitted securely.
- Publications
- Account settings
Preview improvements coming to the PMC website in October 2024. Learn More or Try it out now .
- Advanced Search
- Journal List
- Vaccines (Basel)

Influenza Viruses and Vaccines: The Role of Vaccine Effectiveness Studies for Evaluation of the Benefits of Influenza Vaccines
Claudia maria trombetta.
1 Department of Molecular and Developmental Medicine, University of Siena, 53100 Siena, Italy; [email protected] (E.M.); [email protected] (S.V.); [email protected] (S.M.)
Otfried Kistner
2 VisMederi srl, 53100 Siena, Italy; moc.iredemsiv@rentsik
Emanuele Montomoli
3 VisMederi Research srl, 53100 Siena, Italy
Simonetta Viviani
Serena marchi, associated data.
Not applicable.
Influenza is a vaccine preventable disease and vaccination remains the most effective method of controlling the morbidity and mortality of seasonal influenza, especially with respect to risk groups. To date, three types of influenza vaccines have been licensed: inactivated, live-attenuated, and recombinant haemagglutinin vaccines. Effectiveness studies allow an assessment of the positive effects of influenza vaccines in the field. The effectiveness of current influenza is suboptimal, being estimated as 40% to 60% when the vaccines strains are antigenically well-matched with the circulating viruses. This review focuses on influenza viruses and vaccines and the role of vaccine effectiveness studies for evaluating the benefits of influenza vaccines. Overall, influenza vaccines are effective against morbidity and mortality in all age and risk groups, especially in young children and older adults. However, the effectiveness is dependent on several factors such as the age of vaccinees, the match between the strain included in the vaccine composition and the circulating virus, egg-adaptations occurring during the production process, and the subject’s history of previous vaccination.
1. Introduction
Influenza disease, usually called “the flu”, is a contagious respiratory illness caused by influenza viruses. The common symptoms are fever, aches, chills, chest discomfort, cough, and headache [ 1 ]. The incubation period is very short, typically from 1 to 4 days [ 2 ]. While the majority of infected subjects recover, some develop complications, particularly at-risk groups such as pregnant women, young children, the elderly, and individuals with chronic medical conditions [ 1 , 3 ]. One of the most common influenza-related complications is a secondary bacterial infection by Streptococcus pneumoniae , which increases the morbidity and mortality of influenza infection [ 4 , 5 ].
The World Health Organization (WHO) estimates that influenza alone results in 290,000–650,000 deaths each year due to respiratory diseases without taking into account deaths from other potentially influenza-related diseases [ 6 ]. Before the COVID-19 pandemic, Italy was experiencing peaks in influenza-related death rates, especially among the elderly during some winter seasons. An Italian study conducted over the influenza seasons 2013–2014 to 2016–2017 reported a high proportion of deaths among the elderly (seasons 2014–2015 and 2016–2017) and also high rates in children between 0 and 4 years [ 7 ]. Vaccination is still the most effective way of preventing and controlling influenza morbidity and mortality and as a consequence the WHO recommends 75% vaccine coverage for the vulnerable risk group of elderly persons [ 8 ]. As reported by the Italian Ministry of Health, in the 2020–2021 influenza season, vaccination coverage among subjects aged 65 years and older was 65.3%, higher than the previous years but still not optimal [ 9 ].
Influenza viruses spread mainly from person to person through droplets generated by sneezing, coughing and talking [ 2 ]. While influenza viruses are globally detected all year round, in temperate climates influenza epidemics occur during winter: November–March in the Northern hemisphere and May–September in the Southern hemisphere [ 2 , 10 , 11 , 12 ]. By contrast, influenza seasonality is less defined in tropical regions [ 13 ]. Although the seasonality of influenza in temperate regions has been well characterized, the factors influencing seasonality are still under study. Crowding, cold weather, reduced solar radiation, and low indoor humidity are usually related to influenza epidemics. In addition, intrinsic host factors, which could impact the immune system such as melatonin linked to light/dark cycles and vitamin D, may play a role in influenza seasonality [ 12 , 13 , 14 ].
Influenza viruses are enveloped viruses belonging to the Orthomyxoviridae family. There are four genera (A, B, C, and D), which are classified on the basis of antigenic differences in the nucleoprotein and matrix protein [ 15 ]. Influenza A viruses (IAVs) infect avian and mammalian species with swine and poultry constituting the two key reservoirs. Influenza B viruses (IBVs) are exclusive to humans, apart from seals. Influenza C viruses (ICVs) are mainly human pathogens and are responsible for lower respiratory tract infections, especially during childhood. However, they have also been detected in pigs, dogs, and cattle. The most recent virus is the influenza D virus (IDV), which was first isolated in 2011 from a swine with respiratory disease. However, both epidemiological and serological studies support the hypothesis that cattle are the natural reservoir. To date there is no direct evidence of IDV disease in humans, but antibodies against IDV have been detected, underlining the potential ability of this virus to infect and elicit an immune response in humans and especially in people working with animals and/or exposed to cattle [ 15 , 16 , 17 , 18 , 19 , 20 ].
IAVs are further classified in subtypes on the basis of the combinations of the two main surface glycoproteins: haemagglutinin (HA) and neuraminidase (NA). To date, 18 HAs and 11 NAs have been identified. HA constitutes roughly 80% of the virus surface and plays a key role in the attachment and entry of the virus into the host cell. The antibody response to influenza virus glycoproteins is mostly directed against HA. On the basis of the HA expressed, influenza viruses are further classified into two phylogenetic groups: group 1 and group 2 [ 21 ]. NA is responsible for release of the viral progeny from an infected cell. NA antibodies are not able to prevent infection but may contribute to mitigating the severity and duration of the disease [ 15 , 22 , 23 , 24 ].
IBVs are divided into two antigenic lineages (B/Victoria and B/Yamagata), which diverged in the 1970s and have co-circulated globally in humans since 1985 [ 25 ]. The other influenza viruses currently circulating in humans are type A, subtypes H1N1 and H3N2.
Influenza A and B viruses are subject to frequent mutations of their glycoprotein sequences—mainly those of HA and, at a slower rate, those of the NA—owing to the lack of proofreading polymerase activities [ 22 , 26 ]. “Antigenic drift” refers to minor antigenic changes that enable the virus to escape the host’s immune response and is responsible for annual influenza epidemics. Antigenic drift can sometimes involve changes in the glycosylation patterns of viral glycoproteins. The H3N2 viruses evolve particularly rapidly and more unpredictably than other seasonal influenza viruses. “Antigenic shift”, which occurs only in IAVs, consists of abrupt, major changes in HA or HA/NA, resulting in new influenza subtypes unknown to the human immune system resulting in the lack of immunological protection; the new subtype therefore may have pandemic potential. Antigenic shift may be the result of direct mutational changes of a zoonotic influenza enabling the virus to infect humans directly and to support efficient human-to-human transmission. Alternatively, it can occur through reassortment between zoonotic influenza viruses and seasonal human influenza viruses. The last one means that two influenza viruses infect a common host, such as a pig, resulting in a new influenza virus that has some antigenic determinants of one virus and the host tropism and pathogenicity of the other [ 15 , 24 , 27 ]. Influenza pandemics occur approximately every 10 to 40 years. However, as we have learned from the COVID-19 pandemic, it is still not possible to predict when, where, and how severely they will strike [ 15 , 28 ]. The first pandemic of the XXI century was caused by a new influenza A/H1N1 virus of swine origin, which appeared in Mexico in March and early April 2009 which substituted the former human seasonal influenza H1N1 virus. This pandemic was relatively mild and affected children and young and middle-aged adults more severely than other groups. This unusual pattern of age-related morbidity and mortality was due to pre-existing cross-protection against the new H1N1 virus in subjects older than 60 years. The most devastating pandemic in human history was the so-called “Spanish Flu” in 1918–1919, which caused almost 50 million deaths worldwide and was dubbed “the mother of all pandemics”. Since that time, all influenza A pandemics have been caused by descendants of the 1918 virus [ 29 , 30 , 31 , 32 , 33 ]. The other two great pandemics were the 1957 “Asian Flu”, caused by an H2N2 virus, and the 1968 “Hong Kong Flu”, caused by an H3N2 virus that replaced the H2N2 and which still circulates worldwide as seasonal IAV [ 34 , 35 , 36 ].
Pandemics are quite difficult to predict. A key step in pandemic preparedness is the prompt detection of novel influenza strains as they emerge and before being efficiently transmitted among humans. Some influenza viruses are of particular concern such as avian viruses, although they have not yet caused a pandemic. Since the first outbreak of avian influenza in Hong Kong in 1997, the WHO has reported a total of 239 cases of human infection with A/H5N1 virus, as of 3 February 2022, with 134 deaths (case fatality rate: 56%) [ 37 ]. In addition to H5N1, H7N9, and H10N8, other avian viruses, such as H10N3, are potential pandemic candidates. This highlights the importance of surveillance in both humans and animals, such as wild birds and poultry, which is still the key to controlling the emergence of novel avian influenza strains [ 38 , 39 ]. Notably, at the beginning of 2022, no new cases of human infection by the H7N9 avian influenza virus were reported in the Western Pacific Region. Since early 2013, laboratory-confirmed human infections have totaled 1568, with 616 deaths [ 40 ]. Of 1568 cases, mutations in the HA gene have been reported in 33 cases, providing evidence of a change to pathogenicity in poultry. As yet, however, there is no evidence of sustained human-to-human transmission, and human infections are unusual. Nevertheless, careful monitoring is crucial in order to promptly identify viral changes and transmission patterns that could make the virus a threat to humans [ 34 , 41 , 42 , 43 , 44 ].
2. Annual Update of Seasonal Influenza Vaccines
Owing to the evolving nature of influenza viruses, the composition of vaccines needs annual evaluation in order to include the seasonal viruses predicted to circulate during the next influenza season. Influenza vaccines may be trivalent, containing the A/H1N1 virus, A/H3N2 virus, and one lineage of the B virus, or quadrivalent, containing both B lineages. Since the 2013–2014 influenza season in the Northern Hemisphere, the WHO has recommended the inclusion of both B lineages, as this is deemed to provide broader protection against IBVs. Indeed, the inclusion of one B lineage confers limited protection against the other lineage. Moreover, in some influenza seasons (such as 2001–2002 and 2010–2011) the predominant circulating lineage was different from the one included in the vaccine, resulting in a limited vaccine effectiveness (VE) [ 41 , 45 , 46 , 47 ]. Since 1998, the WHO has held twice-yearly consultations with vaccine regulatory agencies, vaccine manufacturers, members of the WHO Global Influenza Surveillance and Response System (GISRS), global public health laboratories, and academia: one in February for the Northern hemisphere and one in September for the Southern hemisphere. The WHO recommendations provide a guide to which influenza viruses should be included in influenza vaccines for use in the next influenza season. However, it is the responsibility of each national regulatory authority to approve or modify the vaccine composition [ 48 , 49 , 50 ]. The GISRS network, a global system of public health institutions, is coordinated by the WHO, and currently consists of 148 National Influenza Centres (NICs), 7 WHO Collaborating Centres (CCs) for Influenza, 4 WHO Essential Regulatory Laboratories (ERLs), and 13 H5 Reference Laboratories. The GISRS plays a key role in global influenza risk assessment and performs year-round virological surveillance. It has been estimated that the GISRS processed an average of 3.4 million specimens every year from 2014 to 2019, a figure that increased to 6.7 million in 2020 and 2021. The WHO recommendations of viruses for inclusion in the annual seasonal vaccines are based on GISRS surveillance [ 48 ].
Vaccine production takes 6 to 8 months from the WHO recommendations. This is a tight schedule and a complex process with limited flexibility and requires compromises. Consequently, vaccine manufacturers sometimes prefer to start the production of at least one antigen before the official recommendation is issued, in order to be able to manage unexpected events. However, the current system does not allow manufacturers to anticipate the WHO recommendations; thus, in the event of mismatch in April/May, there is not enough time to thoroughly investigate the viral variant that has emerged and, if necessary, to prepare a well-matched vaccine. This could impact on the VE, as happened during the 2014–2015 season in the Northern hemisphere, when an antigenic variation of the H3N2 virus emerged and only little or no VE was observed [ 24 , 46 , 50 , 51 ].
However, other crucial factors contribute to the timely production, evaluation, and delivery of vaccines before the next influenza season. First, the preparation of the Candidate Vaccine Virus (CVV). The CVV is a virus prepared by the CCs for potential use in vaccine manufacturing and should be antigenically similar to the virus recommended in the next influenza season. Since the majority of vaccines are still produced in eggs, the CVV needs to replicate well in eggs. CVVs are crucial for the timely production of egg-based vaccines. In the case of cell-based manufacturing, a different CVV is prepared, while influenza vaccines based on recombinant DNA technology do not require CVVs as they are based on the genetic information of the recommended vaccine viruses [ 3 , 52 ].
Once the CVVs have been ascertained to be antigenically related to the recommended vaccine strains (defined as “-like”), they are supplied to manufacturers, who generate “seed viruses” for the production of inactivated vaccines. A key step of the production process is the availability of “high-yield” (hy) CVV. CVV wildtype viruses poorly grown in eggs will extend the time to produce a sufficient amount of vaccine antigen. The hy, or high-growth reassortant (hgr), was proposed by Kilbourne in 1969 and developed by co-infection of A/PR/8/34 (PR8), a hy donor virus, along with the recommended wild type “target” virus. The generation of hy reassortant vaccine seed viruses is the result of combining the HA and NA from the wild type “target” virus with 1–6 of the remaining genes from the hy donor virus PR8 [ 50 , 51 ].
Other factors which can impair, or delay vaccine production include the timely production of reagents for determination of the HA antigen content in the vaccine and ferret antisera for confirmation of antigenic identity [ 46 , 50 ].
The WHO GISRS, in collaboration with animal health partners, also performs surveillance for zoonotic events, and decisions are made at least twice a year regarding the need to develop CVVs for the purpose of pandemic preparedness [ 53 ]. Over the last 20 years, several zoonotic influenza events have been detected. The selection and development of zoonotic CVVs are aimed at maintaining a bank of viruses that can be promptly used for vaccine development and also at aiding manufacturers that want to develop pilot lots of vaccines [ 41 , 52 ].
3. Licensed Seasonal Influenza Vaccines
The degree of protection conferred by vaccination depends on a complex interplay between vaccine composition and circulating influenza viruses, the age of vaccinees and their history of previous exposure to influenza viruses, and/or influenza vaccinations, as well as product-specific factors such as the vaccine formulation.
To date, three types of influenza vaccines have been licensed: inactivated, live-attenuated, and recombinant HA vaccines. The advantages and disadvantages of licensed seasonal influenza vaccines are summarized in Table 1 .
Summary of advantages and disadvantages of licensed seasonal influenza vaccines.
The inactivated influenza vaccines (IIVs) are the most widely used ones and can be whole virus, split virus, or subunit vaccines; they are administered by intramuscular or subcutaneous injection. Split and subunit IIVs can be administered to all age groups from six months onwards. Whole virus vaccines have been extensively used in humans but are no longer in use in most parts of the world owing to their relatively high reactogenicity. Subunit or split virus vaccines are the most widespread [ 15 , 23 , 46 , 54 ]. The latter are prepared by disrupting the viral membrane chemically. Subunit vaccines contain only the HA and NA glycoproteins of the virus. Currently, none of the licensed vaccines are adjuvanted, with the exception of an MF-59 adjuvanted subunit vaccine licensed for persons of 65 years of age and older to overcome the weakened immune response in this age group, also known as immunosenescence [ 55 ]. Another vaccine licensed for people of 60 (Europe) or 65 (USA) years of age and older is high-dose IIV, which contain a four times higher amount of the HA antigen than the standard dose [ 56 , 57 ].
IIVs are mainly produced in embryonated hens’ eggs, harvested from the allantoic cavity and manufactured according to the type of vaccine. Egg-based production is a long- and well-established traditional system. Its main advantages are the availability of extensive safety data, cost-effectiveness, and high yields of influenza virus antigens. On the other hand, it has several limitations, which have prompted the development and implementation of other platforms. One major issue is the need for a huge number of embryonated hens’ eggs; one or two eggs are usually required for one dose of vaccine. Moreover, subjects with an egg allergy are not recommended to receive this kind of vaccine, owing to the theoretical risk of an anaphylactic reaction, since small amounts of egg proteins may be present in the vaccine. Finally, some viruses, such as H3N2, grow poorly in eggs and could undergo some egg-adaptive changes that could reduce the VE [ 46 , 54 ]. The current alternative for vaccine production is the cell-culture platform. The main advantage of this platform is its independence from the supply of eggs, a crucial aspect in the event of an avian pandemic, as the cells can be cryo-conserved and used at any time when needed, in contrast to the supply of eggs which needs a diligent advance planning for at least 6 months. Another advantage is the freedom of the vaccine from any egg component. Another important advantage of the cell culture platform is in overcoming the risk of egg-adapted mutations which can affect the antigenicity of vaccine viruses. A cell-based quadrivalent IIV produced by Seqirus has been approved and is now widely available [ 57 , 58 ]. However, this production system also has some disadvantages such as shorter experience, the need to qualify production facilities, an extended quality control program including testing for adventitious agents, and higher production costs [ 15 , 46 ]. All current IIVs are standardized with regard to HA content, but not to NA content [ 59 ].
Live influenza vaccines (LAIVs) are administered intranasally, thereby miming natural infection and inducing broader humoral and cellular immune responses than IIVs, including additional mucosal IgA responses in the upper respiratory tract. These attenuated viruses are cold-adapted and are able to replicate at 25 °C, the temperature of the nasal passage, but not at a temperature higher than 35 °C, that of the respiratory tract. LAIVs can provide protection against both well-matched and non-matching influenza strains. These vaccines are administered to healthy subjects, aged 2–49 years, according to the country-specific regulations; however, the use of live viruses makes these vaccines unsuitable for immunocompromised subjects [ 15 , 46 ].
The first vaccine produced by a modern recombinant DNA technology was Flublok ® , which was developed by Protein Science, Meriden, CT, and acquired by Sanofi Pasteur. Initially approved by the Food and Drug Administration in 2013 for use in adults aged 18–49 years, the quadrivalent version replaced the previous one in 2017 for use in adults aged 18 years and older. The quadrivalent vaccine, named Supemtek ® , was also authorized by the European Medicines Agency in 2020 [ 60 , 61 , 62 ]. This technology offers several important advantages; indeed, it does not require eggs or CVVs, and the manufacture of the HA of the target virus starts from the viral RNA sequence by in vitro synthesis of the corresponding DNA. This DNA is cloned in a baculovirus, preferably Autographa californica multiple-capsid nuclear polyhedrosis virus [ 63 , 64 , 65 ]. This approach may be particularly important, allowing a prompt response in the event of a pandemic, based on sequence information rather than the availability of an infectious virus isolate. Clinical studies performed with such vaccines produced by recombinant DNA technology have shown a good immunogenicity and tolerability in younger and elderly adults, the latter being important as these vaccines contain three times more HA antigens than IIVs [ 65 , 66 , 67 , 68 , 69 , 70 , 71 , 72 , 73 ].
Several different types of vaccines based on new technologies and platforms (e.g., plant-based, vector-based, VLP/NP technologies, nucleotide-based technologies, recombinant replication-deficient LAIVs) are now under development with a view to producing next-generation vaccines, in order to complement for or to overcome the drawbacks of the current technologies [ 46 , 74 ]. One of the most appealing approaches for next-generation vaccines is the development of a universal influenza vaccine aimed at providing robust and long-lasting protection against multiple subtypes of influenza viruses. As reported by the National Institute of Allergy and Infectious Diseases, a universal vaccine should protect against group 1 and 2 influenza viruses, should be at least 75% effective, the protection should last at least one year and should be suitable for all age groups. Ideally, the administration of a universal vaccine would avoid the need for annual reformulation and re-administration of seasonal vaccines. So far, the stem of the HA has been identified as the ideal target for antibodies induced by a universal vaccine since it remains unchanged. However, other conserved parts have been explored such as the NA, the ectodomain of the ion channel M2, and the internal viral proteins [ 75 , 76 ]. Another innovative approach is represented by the respiratory vaccine program launched by Moderna. The aim is to develop a combination respiratory vaccine candidate (mRNA-1230) targeting SARS-CoV-2, influenza viruses, and respiratory syncytial virus. This vaccine is designed as an annual booster in order to protect older adults against all three viruses at once and maintain immunogenicity over the years [ 77 ].
4. Influenza Vaccine Effectiveness
Two criteria are used to assess the performance of influenza vaccines: efficacy and effectiveness. Vaccine efficacy refers to the reduced risk of infections and subsequent disease by vaccination in ideal circumstances such as randomized placebo-controlled clinical trials. By contrast, VE refers to the reduced risk of clinical outcomes (e.g., disease incidence, hospital admission) among subjects vaccinated under “real-word” conditions and it is usually evaluated through observational studies. Although randomized placebo-controlled clinical trials are considered the “the gold standard” for measuring vaccine efficacy, they are expensive, and often pose ethical challenges. In addition, efficacy studies with influenza vaccines have a high risk of failure based on differences in attack rates and vaccine matches, resp. mismatches in different seasons. Therefore, the evaluation of VE by performing observational studies is increasingly considered to be a more appropriate approach than efficacy studies. Since 2004–2005, the most widely used method of estimating the VE of seasonal influenza vaccines has been the test-negative design (TND). In this case, patients with signs and symptoms that meet predefined clinical definitions are tested for the presence of influenza viruses; they are classified as cases if they test positive and controls if negative [ 78 , 79 , 80 , 81 , 82 , 83 ].
The VE of current influenza vaccines is suboptimal, having been estimated as 40% to 60% when the vaccines strains are antigenically well-matched with the circulating viruses [ 3 , 84 ].
VE is dependent on several factors such as the age of vaccinees, the match between the strain included in the vaccine composition and the circulating virus, egg-adaptations occurring during the production process, and the subject’s history of previous vaccination as recently supposed [ 3 , 79 , 82 , 83 ].
Vaccine impact is referred to as the reduction in disease incidence where some subjects have received vaccination [ 79 , 80 ].
4.1. Effectiveness in Children
Children younger than 5 years old, and especially those younger than 2 years, are at higher risk of developing serious influenza-related complications. The Centers for Disease Control and Prevention (CDC) estimates that from the 2010–2011 to the 2019–2020 influenza seasons, influenza-related hospitalizations among children younger than 5 years ranged from 7000 to 26,000 in the United States, while influenza-related deaths in children ranged from 37 to 199 from the 2004–2005 to the 2019–2020 influenza seasons [ 85 ].
Children may also play a key role in the spread of influenza infection, shedding the virus in greater quantities and for longer duration than adults. Specifically, IBVs are more likely to affect children and to cause their hospitalization or death [ 24 , 86 , 87 ].
Influenza vaccination is recommended for children aged 6 months and older; two doses at least 4 weeks apart are recommended for children aged 6–59 months at initial vaccination, while children aged 6–35 months receiving a pediatric dose [ 88 ].
Overall, it has been estimated that IIVs are able to reduce the risk of influenza in children aged 2–16 years from 30% to 11%, and LAIVs from 18% to 4% in children aged 3–16 years [ 89 ].
An overview on studies on VE conducted in children is shown in Table 2 .
Overview of studies on VE conducted in children.
With regard to IIVs and children aged up to 59 months, VE varies from moderate to effective depending on the influenza season considered and, in some cases, on diagnostic sensitivity. A study performed to assess VE in preventing laboratory-confirmed influenza infection during the 2011–2012 influenza season in China, a season characterized by a considerable mismatch of H3N2 and B viruses [ 98 ], estimated a VE of 67% (95% CI: 41; 82), indicating that vaccinated children were considerably protected from developing influenza that would have required medical attention [ 90 ]. Moreover, according to the authors, no significant differences were found between children aged 6–35 months (72%, 95% CI: 43; 86) and those aged 36–59 months (50%, 95% CI: −46.3; 82.9). However, a study performed in the Republic of Korea in the influenza season 2017–2018 showed that the VE in the older age group (36–59 months) was higher than that in the younger age group (6–35 months), with 59.3% (95% CI: 8.8; 81.9) versus 44.1% (95% CI: −0.2; 67.8), respectively [ 91 ]. These numbers emphasize that it can be difficult to draw valid conclusion when comparing VE data of different seasons, resp. different regions. In addition, VE may also vary according to the diagnostic tools, such as a rapid influenza diagnostic test and the reverse transcription polymerase chain reaction (RT-PCR), the latter being considered as the gold standard for molecular detection of influenza viruses [ 99 ]. In the Republic of Korea study the adjusted VE was 53.4% (95% CI: 25.3; 70.5) according to RT-PCR and 14.8% (95% CI: −4.4; 30.0) according to the rapid influenza diagnostic test (RIDT). When the different virus subtypes were considered, the VE based on RT-PCR gave 68.8% (95% CI: 38.7; 84.1) against IAVs and 29.7% (95% CI: −35.1; 61.8) against IBV. This study was performed in the 2017–2018 season, which was characterized by a mismatch between the circulating IBV (B/Yamagata-lineage) and the vaccine strain (B/Victoria-lineage); this probably explained the low VE against IBV. This is also supported by the VE data based on the RIDT of 24.2% (95% CI: 3.1; 40.2) against influenza A and a negative VE value of −5.1% (95% CI: −42.6; 21.4) against influenza B. Another study in the US using the Department of Defense’s Data Base DoDGRS evaluated the VE against medically-attended, laboratory-confirmed influenza infections in children aged 6 months to 17 years (6–11 months; 12–23 months; 2–4 years; 5–8 years; 9–12 years and 13–17 years) over four influenza seasons (from 2016–2017 to 2019–2020) [ 92 ]. The VE against any type of influenza was low and non-significant in the youngest children (aged 6–12 months) and high in the 12–23- month age-group; it then declined linearly with increasing age with an overall VE of 42% (95% CI: 37; 47). The study also showed that the VE was also very type/subtype- and strain-specific, with the highest value of 55% (95% CI: 47; 61) against influenza A/H1N1pdm09, followed by 49% (95% CI: 41; 55) against influenza B, and being the lowest against A/H3N2 with only 37% (95% CI: 28; 45) [ 92 ]. Another study evaluating the VE among children aged 6 to 72 months during two influenza seasons (2016–2017 and 2017–2018) reported an overall VE of 58% (95% CI: 31; 74). When analyzed by age groups, 6–35 months and 36–72 months, the lowest VE (28%, 95% CI: −80; 71) was found in the youngest group [ 93 ].
Recently, it was considered that repeated vaccinations can impair the antibody response, especially in the elderly; however, this consideration is still a topic of controversial discussions [ 100 , 101 , 102 , 103 , 104 , 105 , 106 ]. The mechanism behind this phenomenon is poorly understood, however different explanations have been proposed. One is the “negative interference” or “the antigenic distance hypothesis” based on the match between the virus strains present in the prior and current vaccine and the antigenic difference with the drifted circulating virus. In this circumstance, the immune system could be too focused on the viruses contained in the vaccines and less effective against the drifted one. Another explanation may be the antibody sequestration meaning that antibodies developed following the prior vaccination bind to subsequent vaccine antigens and prevent the exposure to the immune system. Other studies have reported that repeat vaccination with the same influenza vaccines reduces antibody-affinity maturation and decreases the B-cell response [ 107 , 108 , 109 , 110 , 111 , 112 , 113 , 114 ]. This has been at least partially addressed in two studies [ 94 , 95 ]. A meta-analysis of 28 suitable published studies in children between 6 months and 17 years of age showed that influenza vaccination offered higher protection in children who were fully vaccinated (VE of 61.79%; 95% CI: 54.45; 69.13), compared to those who were partially vaccinated (33.91%; 95% CI: 21.12; 46.69) against any influenza type [ 94 ] and of 53% (95% CI: 45; 60) in 2016–2017 and 2017–2018 influenza seasons [ 95 ]. Overall, both studies [ 94 , 95 ] concluded that influenza vaccination provided substantial protection in children, even in seasons characterized by a high disease burden and the predominant circulation of mismatched viruses, such as H3N2 [ 95 ]. However, another study found an overall VE of 63% (95% CI: 38; 78) against critical illness due to any influenza virus, with no difference in protection between age-groups or between fully and partially vaccinated children [ 96 ]. Interestingly, this VE estimate was made during the 2019–2020 influenza season, which was characterized by a mismatch between the predominant influenza viruses and the vaccine strain; it therefore underlined the benefit of vaccination in reducing the risk of life-threatening influenza in children.
VE in subjects <17–18 years ranged from 42% (95% CI: 37; 47) [ 92 ] to 33% (95% CI: 24.3; 40.7) [ 97 ] over four consecutive influenza seasons, from 2016–2017 to 2019–2020 [ 92 ] and from 2012–2013 to 2015–2016 [ 97 ]. The consensus is that vaccination is protective in children, though age-related differences were found in some cases and the lowest protection was observed against H3N2 [ 92 , 94 , 95 , 96 , 97 ].
Overall, the question on the need of influenza vaccination in children is still open. On the one side, there is evidence of moderate IIV VE in children against medically, laboratory-confirmed influenza infection, to prevent substantial influenza associated life-threatening illness requiring invasive mechanical ventilation, a strong predictor of death, to reduce the overall risk of influenza-associated hospitalizations, and finally of a possible indirect benefit to reduce the transmission to (high-) risk groups such as elderly adults. On the other hand, VE seems to be variable and suboptimal, with differences being related to the season, child’s age, antigenic match/mismatch, and the manufacturing process. In addition, since vaccination is recommended for children aged 6 months and older, a promising strategy may be maternal immunization [ 86 , 92 , 95 , 96 , 97 , 115 , 116 ].
4.2. Effectiveness in Adults and the Elderly
While influenza may be serious and can affect anyone, adults aged 65 years and older have a higher risk of developing serious influenza-related complications than young and healthy adults. The CDC estimates that, in recent years, between 70% and 85% of seasonal influenza-related deaths and 50–70% of hospitalizations have occurred in subjects aged ≥65 years, defined as older adults/elderly [ 117 , 118 ]. Vaccination is therefore strongly recommended for the elderly, in order to prevent the disease and its related complications.
An overview on studies on VE conducted in adults and the elderly is shown in Table 3 .
Overview of studies on VE conducted in adults and the elderly.
VE in the elderly has been evaluated in several influenza seasons from 2010–2011 to 2019–2020, and several studies have suggested that it exerts an overall protective effect even in elderly people with cardiovascular and/or respiratory diseases and those over 75 years old [ 119 , 120 , 124 , 125 , 130 ]. For instance, one study evaluated VE over eight seasons, and found that, in every season, vaccination reduced the risk of severe influenza disease in vaccinated subjects aged ≥65 years by 16% (95% CI: 12; 19) to 48% (95% CI: 41; 54) [ 119 ]. A study performed in China (Beijing) during the 2013–2014 influenza season reported that VE was favourable (59%, 95% CI: −79; 90) against H1N1, suboptimal against H3N2 (22%, 95% CI: −253; 83) and not present against B viruses (−20%, 95% CI: −239; 58); this last finding was attributed to genetic-lineage mismatch with the vaccine reference component for the B/Yamagata-lineage strain [ 120 ]. The same was observed during the 2015–2016 season and was explained by a mismatch between the circulating B-Victoria lineage and the B-Yamagata lineage viruses included in the vaccine [ 121 ].
Differences have also been observed according to the type of vaccine administered to the elderly. Adjuvanted IIVs seem to yield higher VE values than non-adjuvated IIVs, more effectively reducing the risk of hospitalization for influenza or pneumonia and significantly reducing influenza-related medical consultations [ 122 , 131 ]. In addition, adjuvanted IIVs are reported to significantly reduce influenza-related medical consultations in adults aged ≥65 years with at least one medical condition, in comparison with subjects receiving conventional non-adjuvanted IIVs [ 123 ]. Notably, these findings were observed during the 2017–2018 and 2018–2019 influenza seasons, which were characterized by “high” and “moderate” disease severity, respectively. The overall VE in subjects aged ≥65 years was 17% (95% CI: −14; 39) in 2017–2018 and 12% (95% CI: −31; 40) in 2018–2019 [ 132 , 133 ]. Moreover, the high-dose IIV also showed the potential to reduce hospitalizations and emergency room visits for influenza and pneumonia in the elderly over six influenza seasons (from 2012–2013 to 2017–2018) [ 134 ].
Further studies have compared VE between adults and the elderly, with different conclusions [ 124 , 125 , 126 , 127 ]. One comparison of VE over five influenza seasons (from 2011–2012 to 2015–2016) between a reference group of adults aged 18–49 years and elderly (65–74 years, ≥75 years, and ≥65 years) found an overall VE of 48% (95% CI: 41; 54) against H1N1 in the adults and of 49% (95% CI: 22; 66) in the elderly, of 55% (95% CI: 45; 63) and 62% (95% CI: 44; 74) against B viruses, and of 21% (95% CI: 9; 32) and 14% (95% CI: −14; 36) against H3N2, respectively. Excluding the 2014–2015 season, during which the circulating virus was antigenically different from the seasonal vaccine strain, the VE increased to 35% (95% CI: 22; 46) in adults and 17% (95% CI: −26; 45) in elderly. Overall, no consistent pattern of lower VE in any of the elderly subgroups compared to among adults was found, suggesting that influenza vaccination provided similar levels of protection in both the elderly and adults [ 125 ]. A further study reported a moderate VE (42.8%, 95% CI: 23.8; 57.0) against influenza-related hospitalization in subjects aged ≥16 years with a lower trend in adults aged 16–64 years (33.2%, 95% CI: −6.7; 58.2) [ 126 ]. Conversely, another two studies concluded that the protection provided by influenza vaccines was moderate against influenza-associated hospitalizations and medically-attended laboratory-confirmed infection among adults, while the VE against H3N2 was low in the elderly, especially in mismatched seasons [ 92 , 124 ]. The overall VE was 51% (95% CI: 44, 58) among adults, 48% (95% CI: 37; 89) against H1N1, 37% (95% CI: 24; 50) against H3N2, and 38% (95% CI: 23; 53) against B viruses, while it was significantly lower (37%) (95% CI: 30; 44) in the elderly. The biggest differences between matched and mis-matched strains were seen in persons from 65 years onwards when the VE against A(H3N2) was 43% (95% CI: 33; 53) in seasons when circulating and vaccine strains were antigenically similar and 14% (95% CI: −3; 30) when A(H3N2) variant viruses predominated which results in a negligible protection against hospitalization during seasons with mismatched H3N2 viruses [ 124 ]. A lower VE against H3N2, in addition to H1N1, in adults aged ≥65 years old was also reported in another study [ 127 ], clearly demonstrating the lower VE of IIV in this specific age-group. The reduced VE could be explained by immunosenescence, an age-related decline in the normal function of the immune system [ 135 ]. However, other factors and mechanisms may also influence the immune response to influenza vaccines and need further investigation. Moreover, influenza vaccination has been associated with a 41% reduction in the risk of hospitalization for influenza illness, with an overall VE of 41% (95% CI: 27; 52) against all influenza viruses among adults (median age 63 years) in 2019–2020 [ 128 ]. In the same season, a significant VE (53%, 95% CI: 30; 69) among working adults in Japan was reported; this contrasted with a low VE of 28% (95% CI: −5; 50) in season 2017–2018 and an even negative VE of −11% (95% CI: −42; 14) in season 2018–2019, which testifies to the fact that effectiveness of influenza vaccines may substantially vary from season to season according to the circulating influenza viruses, antigenic matches and mismatches, pre-existing immunity, and different age-groups [ 129 ].
5. Conclusions
It is commonly accepted in the scientific and medical communities that vaccination remains the most effective method of controlling the morbidity and mortality of seasonal influenza, especially with respect to (high-) risk groups such as young children, elderly adults, persons with chronic medical conditions, and immunocompromised persons. However, it is very difficult to evaluate the efficacy of influenza vaccines in these risk groups as placebo-controlled efficacy studies are for ethical reasons not accepted by most of the Regulatory Authorities and Ethic Committees.
So-called effectiveness studies which investigate the positive effects of influenza vaccines in the field are an excellent alternative. However, the results of such effectiveness studies are very divergent, and results are sometimes even contradictive. Nevertheless, the selection of single studies or meta-analyses of a variety of studies confirm that in general influenza vaccines are effective against morbidity and mortality in all age and risk groups, especially in young children and elderly adults. However, the outcome of such effectiveness studies is very dependent on influenza virus-specific parameters, mainly the antigenic drift, especially of the A-strains or the differently circulating B-strains of the two divergent lineages B-Victoria and B-Yamagata. The negative impact of the latter was overcome by the introduction of the quadrivalent vaccines with representative vaccine strains of both lineages. A more challenging issue is the antigenic drift of the A strains, especially for H3N2 viruses, which can result in a complete lack of protection in specific seasons. This issue can be exacerbated by the conventional influenza vaccine production technology of embryonated hens’ eggs which carries the risk of antigenically mis-matched vaccine virus by the introduction of egg-adapted mutations. This issue can be overcome by new technologies such as cell culture or the use of recombinant vaccines which ensure the antigenic similarity of the vaccine strains with the wildtype viruses circulating in nature. Nevertheless, even these vaccines may face the limitations of potential mismatches between the circulating strains and the WHO-recommended vaccine strains caused by antigenic drift, especially during an influenza season. However, novel technologies, namely recombinant RNA/DNA-platforms, may offer a higher flexibility in addressing antigenic changes during an influenza season potentially allowing the adaptation of the vaccine during the season. This, of course, would also require higher flexibility and accelerated responses of Regulatory Authorities.
Finally, the experience based on a variety of effectiveness studies would strongly raise the bar for so-called “universal influenza vaccines” or, more precise, “influenza vaccines with a broadened and longer-lasting immunity” would require studies over several seasons without any prediction of the importance of the antigenic drift over these seasons carrying a high risk of failure within one specific season. Therefore, such studies most likely cannot be performed by individual vaccine producers but may need the intensive support of all stakeholders involved in the timely production of seasonal influenza vaccines, i.e., the WHO, International Institutions, the scientific community, and, last, but not least, Regulatory Authorities worldwide.
Funding Statement
This research received no external funding.
Author Contributions
Conceptualization, S.V.; writing—original draft preparation, C.M.T.; writing—review and editing, O.K., E.M., S.V. and S.M. All authors have read and agreed to the published version of the manuscript.
Institutional Review Board Statement
Informed consent statement, data availability statement, conflicts of interest.
E. Montomoli is an external consultant and Chief Scientific Officer of VisMederi srl and VisMederi Research srl.
Publisher’s Note: MDPI stays neutral with regard to jurisdictional claims in published maps and institutional affiliations.
- Jacobs School of Medicine and Biomedical Sciences
- School of Engineering and Applied Sciences
- UB Directory
- Department of Biomedical Engineering >
- News and Events >
- Latest News >
Vaccine in Development Could Offer Better Flu Protection
Published April 6, 2024
Related Links
- Jonathan Lovell
The Good Men Project reposted an article from Futurity that reported on a study, co-authored by Jonathan Lovell in which UB researchers are developing a recombinant flu vaccine that would offer several advantages over conventional vaccines.
UB Research News
- 4/17/24 Did RNA droplets help originate life on Earth?
- 4/17/24 Happier neighborhoods start in the front yard
- 4/16/24 Charting family history of world's most popular coffee
- 4/16/24 New study probes macrophages’ role in pulmonary fibrosis
- 4/15/24 Nouh named fellow of ASME
Thank you for visiting nature.com. You are using a browser version with limited support for CSS. To obtain the best experience, we recommend you use a more up to date browser (or turn off compatibility mode in Internet Explorer). In the meantime, to ensure continued support, we are displaying the site without styles and JavaScript.
- View all journals
- Explore content
- About the journal
- Publish with us
- Sign up for alerts
- Published: 28 June 2018
- Florian Krammer 1 ,
- Gavin J. D. Smith 2 , 3 ,
- Ron A. M. Fouchier 4 ,
- Malik Peiris 5 , 6 ,
- Katherine Kedzierska 7 ,
- Peter C. Doherty 7 , 8 ,
- Peter Palese 1 , 9 ,
- Megan L. Shaw 1 ,
- John Treanor 10 ,
- Robert G. Webster 11 &
- Adolfo García-Sastre 1 , 9 , 12
Nature Reviews Disease Primers volume 4 , Article number: 3 ( 2018 ) Cite this article
118k Accesses
797 Citations
164 Altmetric
Metrics details
- Influenza virus
- Respiratory tract diseases
- Viral pathogenesis
Influenza is an infectious respiratory disease that, in humans, is caused by influenza A and influenza B viruses. Typically characterized by annual seasonal epidemics, sporadic pandemic outbreaks involve influenza A virus strains of zoonotic origin. The WHO estimates that annual epidemics of influenza result in ~1 billion infections, 3–5 million cases of severe illness and 300,000–500,000 deaths. The severity of pandemic influenza depends on multiple factors, including the virulence of the pandemic virus strain and the level of pre-existing immunity. The most severe influenza pandemic, in 1918, resulted in >40 million deaths worldwide. Influenza vaccines are formulated every year to match the circulating strains, as they evolve antigenically owing to antigenic drift. Nevertheless, vaccine efficacy is not optimal and is dramatically low in the case of an antigenic mismatch between the vaccine and the circulating virus strain. Antiviral agents that target the influenza virus enzyme neuraminidase have been developed for prophylaxis and therapy. However, the use of these antivirals is still limited. Emerging approaches to combat influenza include the development of universal influenza virus vaccines that provide protection against antigenically distant influenza viruses, but these vaccines need to be tested in clinical trials to ascertain their effectiveness.
Introduction
Influenza is an infectious respiratory disease; in humans, it is caused by influenza A (genus influenzavirus A ) and influenza B (genus influenzavirus B ) viruses ( influenzavirus C and influenzavirus D genera are also known). Symptoms associated with influenza virus infection vary from a mild respiratory disease confined to the upper respiratory tract and characterized by fever, sore throat, runny nose, cough, headache, muscle pain and fatigue to severe and in some cases lethal pneumonia owing to influenza virus or to secondary bacterial infection of the lower respiratory tract. Influenza virus infection can also lead to a wide range of non-respiratory complications in some cases — affecting the heart, central nervous system and other organ systems 1 , 2 . Although characterized by annual seasonal epidemics, sporadic and unpredictable global pandemic outbreaks also occur that involve influenza A virus strains of zoonotic origin. Pandemic influenza occurs every 10–50 years and is characterized by the introduction of a new influenza A virus strain that is antigenically very different from previously circulating strains; the lack of pre-existing immunity in humans is often associated with the severity of the infection and an increase in mortality.
All influenza viruses are enveloped negative-sense single-strand RNA viruses with a segmented genome. Influenza A and influenza B viruses contain eight RNA segments, which encode RNA polymerase subunits, viral glycoproteins (namely, haemagglutinin (HA), with its distinct globular ‘head’ and ‘stalk’ structures, which facilitate viral entry, and neuraminidase (NA), which facilitates viral release), viral nucleoprotein (NP), matrix protein (M1) and membrane protein (M2), the nonstructural protein NS1 and nuclear export protein (NEP) (Fig. 1 ). The HA and NA viral proteins are the most antigenically variable, and in the case of influenza A virus, they are classified into antigenically diverse subtypes. These two viral glycoproteins are located at the surface of the virus particle and are the main targets for protective antibodies induced by influenza virus infection and vaccination. Each influenza virus isolate is named according to its type or genus, host and place of isolation, isolate number and year of isolation (Box 1 ). Influenza C and influenza D viruses have only seven RNA segments and do not seem to cause substantial disease in humans. However, influenza C virus infections can cause influenza-like illness and hospitalizations in some instances, especially in children 3 .
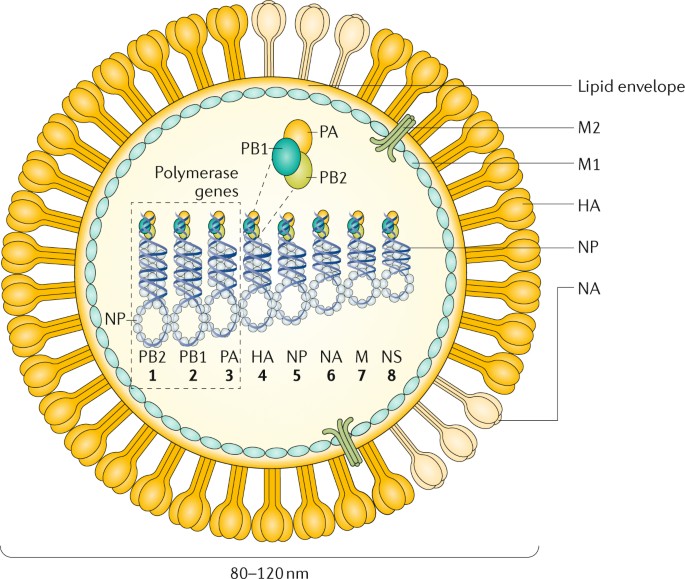
The figure represents an influenza A virus particle or virion. Both influenza A and influenza B viruses are enveloped negative-sense RNA viruses with genomes comprising eight single-stranded RNA segments located inside the virus particle. Although antigenically different, the viral proteins encoded by the viral genome of influenza A and influenza B viruses have similar functions: the three largest RNA segments encode the three subunits of the viral RNA-dependent RNA polymerases (PB1, PB2 and PA) that are responsible for RNA synthesis and replication in infected cells; two RNA segments encode the viral glycoproteins haemagglutinin (HA, which has a ‘stalk’ domain and a ‘head’ domain), which mediates binding to sialic acid-containing receptors and viral entry, and neuraminidase (NA), which is responsible for releasing viruses bound to non-functional receptors and helping viral spread. The RNA genome is bound by the viral nucleoprotein (NP), which is encoded by RNA segment 5. RNA segments 6 and 8 encode more than one protein, namely, the matrix protein (M1) and membrane protein (M2) — BM2 in the case of influenza B — and the nonstructural protein NS1 (not shown) and nuclear export protein (NEP). The M1 protein is thought to provide a scaffold that helps the structure of the virion and that, together with NEP, regulates the trafficking of the viral RNA segments in the cell; the M2 protein is a proton ion channel that is required for viral entry and exit and that, together with the HA and NA glycoproteins, is located on the surface of the virus anchored in a lipid membrane derived from the infected cell. Finally, the NS1 protein is a virulence factor that inhibits host antiviral responses in infected cells. The influenza viruses can also express additional accessory viral proteins in infected cells, such as PB1–F2 and PA-x (influenza A), that participate in preventing host innate antiviral responses together with the NS1 protein or NB (influenza B), the function of which is unknown. NS1, NEP, PB1–F2 and PA-x are not present in the virus particle or are present in only very small amounts. NB is a unique influenza B virus surface protein anchored in the lipid membrane of the virus particles.
The segmented nature of the influenza viral genome enables reassortment, that is, interchange, of genomic RNA segments when two viruses of the same type (that is, two influenza A viruses or two influenza B viruses) infect the same cell. A unique characteristic of influenza A viruses is that they circulate not only in humans but also in domestic animals, pigs, horses and poultry and in wild migratory birds (>100 species of ducks, geese, swans, gulls, waders and wild aquatic birds are considered natural reservoirs 4 , 5 ). A total of 16 antigenically different HA and 9 antigenically different NA serotypes or subtypes (18 HA and 11 NA, if including bat influenza A-like viruses, which are phylogenetically close to influenza A virus but unable to reassort with influenza A viruses 6 , 7 ) have been identified among the different avian strains of influenza A virus 8 . These animal reservoirs provide a source of antigenically diverse HA and NA genes that can be exchanged between viral strains by reassortment after co-infection of the same host, increasing virus diversity and in some instances leading to the generation of human pandemic influenza virus strains with HA and/or NA derived from animal strains. The HA subtypes can be grouped into two groups on the basis of the phylogeny of the HA molecule. Within each group, the domain encoding the HA stalk is antigenically similar. By contrast, influenza B and influenza C viruses are not divided into different subtypes and are restricted to humans, with no known animal reservoirs, although limited spillover to seals and pigs has occurred, respectively 9 , 10 . However, influenza B viruses have recently diverged into two antigenically different lineages (B/Victoria/2/1987-like and B/Yamagata/16/1988-like) that are currently co-circulating in humans, which prompted the inclusion of these two lineages, in addition to the influenza A H1N1 and H3N2 viruses, in many of the seasonal human influenza vaccines. Influenza C viruses are usually associated with very mild or asymptomatic infections in humans. In addition, influenza D virus is distantly related to influenza C virus, and it has been isolated from pigs and cows 11 , 12 .
In 2009, the first influenza virus pandemic of this century was caused by a novel H1N1 influenza A virus reassortant that was previously circulating in pigs 13 , 14 . Vaccines were not available on time to contain the first waves of infection. Current influenza antiviral adamantane drugs that target the viral M2 ion channel and inhibitors of NA enzymes have limitations, as viral resistance against M2 inhibitors is prevalent in the currently circulating human influenza A H1N1 and H3N2 strains; viruses resistant to the NA inhibitor oseltamivir have been prevalent among influenza A H1N1 strains since just before the 2009 pandemic 15 . Intriguingly, the adamantane-resistant, oseltamivir-sensitive 2009 pandemic influenza A H1N1 viruses replaced the previously circulating oseltamivir-resistant, adamantane-sensitive seasonal influenza A H1N1 virus that circulated globally in 2008–2009. Although detection of the currently circulating influenza A H1N1 viruses harbouring the NA-H275Y mutation, which imparts oseltamivir resistance, has been noted, emergence of resistance to NA inhibitors is not a major public health problem today 16 . Current seasonal influenza vaccines have only suboptimal effectiveness across all age groups, particularly in elderly individuals (a high-risk group for severe influenza virus infection). Thus, there is a major need to better understand the biology of influenza virus infections to develop new and more-effective antiviral agents and vaccines.
In this Primer, we discuss the biological features of influenza viruses and their effects on human and animal health, as well as the mitigation strategies to reduce the burden of influenza. We focus more on influenza A viruses because of their unique pandemic potential compared with other influenza viruses.
Box 1 Influenza virus nomenclature
Each influenza virus isolate receives a unique name according to a set of rules. First, the name denotes the type of influenza virus (A, B, C or D), followed by the host species from which the virus was isolated (if not specified, the isolate is considered human), the geographical location at which the virus was isolated, the isolate number and the year of isolation. In the case of influenza A viruses, the haemagglutinin (HA) and neuraminidase (NA) subtype is also usually indicated after the viral isolate name. For example, influenza A/Turkey/Ontario/6118/1968 (H8N4) virus is an influenza A virus isolated from a turkey in Ontario in 1968, isolate number 6118; the virus isolate has an HA from the HA antigenic subtype 8 and an NA from the NA antigenic subtype 4.
Epidemiology
Influenza in humans.
The major burden of disease in humans is caused by seasonal epidemics of influenza A and influenza B viruses, with most of the infections occurring in children, although most of the severe cases involve very young or elderly individuals. Seasonal influenza A H1N1 and H3N2 currently circulate in humans, but influenza A H2N2 viruses were the only human circulating influenza A viruses from 1957 to 1968. Before 1918, conclusive evidence of the circulating subtypes is not available. In addition to seasonal epidemics, the introduction of influenza A viruses from either avian or swine populations has led to four pandemics since 1918, with those viruses subsequently becoming seasonal epidemic strains in subsequent years (Fig. 2 ). During pandemics, influenza viruses spread very quickly from the point of origin to the rest of the world in several waves during the year (Fig. 2 ) owing to the lack of pre-existing immunity, which could also contribute to increased virulence. Studies on the transmission of seasonal influenza A virus in humans have proposed that populations in southeast Asia, eastern Asia and/or the tropics act as permanent sources for seeding seasonal epidemics 17 , 18 , whereas other studies indicate that multiple geographical regions might act as seed populations for virus migration 19 . However, a lack of sufficient sequence data from areas such as Africa, India and South America currently prevents a complete understanding, as viral sequences, which are not routinely obtained during diagnosis, are required to ascertain the relationships between viruses spreading in different areas of the world. Seasonal influenza virus outbreaks typically occur in the winter months, when low humidity and low temperatures favour transmission; two ‘influenza seasons’ occur per year: one in the Northern Hemisphere and one in the Southern Hemisphere. However, unlike temperate regions, seasonal influenza patterns are very diverse in tropical countries. Climate factors, including minimum temperature, hours of sunshine and maximum rainfall, seem to be the strongest predictors of influenza seasonality 20 . Seasonal influenza B viruses co-circulate in humans with influenza A viruses and follow the same patterns of transmission.
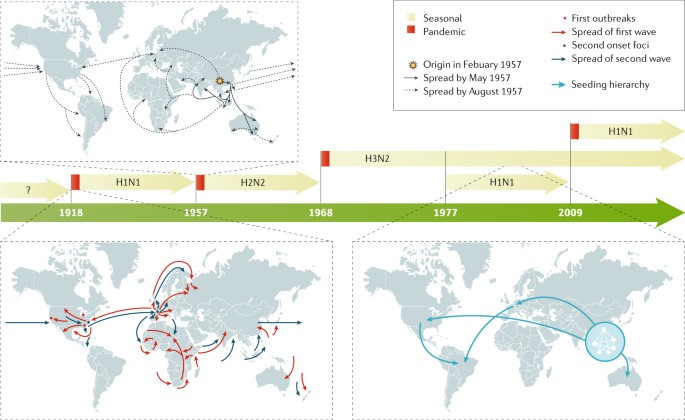
In the past 100 years, four pandemics of human influenza have occurred, with the 1918 pandemic caused by an influenza A H1N1 virus being the most devastating, as it was associated with >40 million deaths 236 . Influenza A H2N2, H3N2 and H1N1 viruses caused the 1957, 1968 and 2009 pandemics, respectively. In 1977, influenza A H1N1 restarted circulation in humans without causing a pandemic, as the strain was similar to that which preceded the 1957 influenza A H2N2 pandemic. By contrast, the 2009 pandemic influenza A H1N1 virus was antigenically very different to the previous seasonal influenza A H1N1 viruses and replaced them as the circulating influenza A H1N1 strain. Examples of the spreading of human influenza A viruses in the world are shown for pandemic 1918 and 1957 viruses and for seasonal H3N2 viruses 18 . For pandemic virus outbreaks, the arrows indicate the first and second waves of transmission. For seasonal influenza A H3N2 spread, the arrows indicate the seeding hierarchy of seasonal influenza A (H3N2) viruses over a 5-year period, starting from a network of major cities in east and southeast Asia; the hierarchy within the city network is unknown. Seasonal influenza B viruses (not shown) are co-circulating in humans with influenza A viruses.
In the United States and from 2010 to 2017, the Centers for Disease Control and Prevention (CDC) has estimated that influenza virus infection has resulted in between 9.2 million and 35.6 million illnesses and between 140,000 and 710,000 hospitalizations 21 . In a typical year, 3–5 million cases of severe illness are caused by seasonal influenza virus infection in the world. Children seem to be the main human transmitters of influenza viruses, as illustrated by studies showing that vaccination of children reduces the incidence of severe influenza virus infections in elderly individuals 22 . Incidence of influenza virus infections increases during pandemic years owing to the lack of pre-existing immunity against the new virus, but severity varies depending on the pandemic virus itself, with the 1918 influenza A H1N1 pandemic being the most severe of the past 100 years.
Zoonotic human infections with influenza A virus strains of swine and avian origins are rare, with specific strains, such as the avian H5N1 and H7N9 and the so-called swine H3N2 variant viruses, being the most prevalent but confined to a limited number of human cases in specific geographical areas, with only limited human-to-human transmission. Prior age-related immunological imprinting in specific age groups acquired by exposure to viruses of the same or related subtype is associated with reduced incidence of disease with novel subtype influenza viruses 23 .
Influenza A and influenza B viruses cause epidemic seasonal infections, resulting in ~500,000 deaths annually worldwide 24 , with the most recently calculated estimates being 291,243–645,832 deaths per year during the 1999–2015 period 25 . Seasonal influenza virus-associated deaths in the United States range from 5,000 to 52,000 people per year, depending on the year 26 . Several comorbidities are known to increase the risk of lethal influenza virus infection. In addition, pregnancy and age are known risk factors, with very young (<1 year of age) and elderly (>65 years of age) individuals being the most vulnerable populations. Although mortality is low in children >2 years of age and young adults, a substantial number of deaths owing to influenza virus infection have been recorded in young individuals with no known predisposition factors. Approximately 100 children die each year from influenza in the United States; this number has been stable since 2010 (ref. 27 ). Severe disease and/or mortality in patients with influenza virus infection are in general due to either virus-induced pneumonia or secondary bacterial superinfection. Primary viral pneumonia is characterized by high levels of viral replication in the lower respiratory tract accompanied by strong pro-inflammatory responses, also referred to as cytokine storm.
Risk factors
Young children who have not had multiple previous exposures to influenza viruses are fairly susceptible to infection and typically have higher fever and more-severe symptoms and shed larger number of virus particles for longer periods of time. Older adults, either because of immunosenescence, concurrent chronic illnesses or both, are also at risk of more-severe illness, hospitalization and infectious complications 28 . In addition to the extremes of age, other groups at risk of severe disease, hospitalization or death include those with chronic pulmonary or cardiac conditions, diabetes mellitus or immunocompromising conditions 29 , 30 . Possibly because of difficulty handling respiratory secretions, individuals with neuromuscular disorders are also recognized as being at increased risk 31 . Pregnancy has long been recognized as a risk factor for severe influenza disease, possibly because of the increased cardiopulmonary demands of pregnancy or the effects of pregnancy on the immune system; the risk increases by trimester and persists into the post-partum period 32 . During the 2009 pandemic with influenza A H1N1, morbid obesity with body mass index >35–40 kg per m 2 was also recognized as an important risk factor 33 . Obesity is associated with excessive influenza virus replication, increased severity of secondary bacterial infections and reduced vaccination efficacy 34 . Finally, the role of host genetic factors in disease remains poorly studied, but polymorphisms and nonsense mutations in several host genes (such as IFITM3 and IRF7 , which encode genes involved in the interferon pathway) have been shown to predispose to severe disease 35 , 36 , 37 .
Influenza in animals
Infection of waterfowl with low pathogenic avian influenza A (LPAI) viruses, called as such owing to their lack of lethality in poultry, produces little or no clinical symptoms 38 . However, upon transmission to poultry, LPAI can cause substantial disease symptoms; in addition, viruses with H5 and H7 subtypes can become highly pathogenic (that is, lethal) in poultry. Outbreaks of highly pathogenic avian influenza A (HPAI) throughout Eurasia and northern Africa are the major influenza disease burden in animals, with >400 million birds killed and economic losses totalling US$20 billion in the first 10 years of the 21st century 39 . Outbreaks of HPAI in poultry have also caused considerable problems in the United States, with the HPAI H5N x (where x = 1–9) viruses, derived by reassortment of the Asian HPAI H5N1 viruses, being a recent example 40 . Recently, LPAI H7N9 viruses circulating in China have become HPAI through the acquisition of a multibasic cleavage site in their HAs and have also caused some self-limiting infections in humans 41 . Influenza A viruses can also cause mild to severe respiratory infection in a variety of mammals, including swine, horses and dogs; additionally, an outbreak of an influenza A virus of avian origin was detected in cats in New York, as first reported in December 2016. From its first report until February 2017, the influenza A H7N2 feline influenza A virus infected ~500 shelter cats and a veterinarian who treated one of the infected cats 42 .
Mechanisms/pathophysiology
Human influenza viruses are transmitted through the respiratory route, whereas avian influenza viruses in wild birds are transmitted through the faecal–faecal, faecal–oral or faecal–respiratory routes. Depending on the route of transmission, the virus targets epithelial cells of the respiratory or intestinal tract for infection and productive replication. In addition, some avian influenza A viruses, especially those of the H7 subtype, have been associated with human infections of the eye and conjunctivitis (inflammation of the conjunctiva) 43 . Severity of infection in humans is associated with replication of the virus in the lower respiratory tract, which is accompanied by severe inflammation owing to immune cell infiltration.
Transmission in animals
The main reservoir of diverse strains and subtypes of influenza A virus is wild birds, especially migratory ducks and geese. Indeed, domestic ducks raised on open ponds are the logical intermediaries between the reservoirs of influenza viruses in wild aquatic birds and other domestic poultry (Fig. 3 ). Transmission between birds can occur directly from contaminated water. Although most of the 16 HA and 9 NA influenza A subtypes cause asymptomatic infections in avian species, H5N x and H7N x subtypes can evolve into HPAI strains through the acquisition of a novel cleavage site in HA (which enables HA maturation by ubiquitous host proteases and spread of the virus outside the respiratory and intestinal epithelia to multiple organs, including the brain) and can cause lethal infections in chicken, turkey and some breeds of domestic duck 44 , 45 . Transmission of influenza viruses to multiple domestic poultry species occurs through so-called backyard farming, whereby species are raised together and in live poultry markets; subsequent transmission to commercial avian farms can occur through the lack of biosecurity (that is, procedures or measures designed to isolate and protect animals and humans from contact with infectious viruses) and because of viral spread through live markets 46 (Box 2 ).
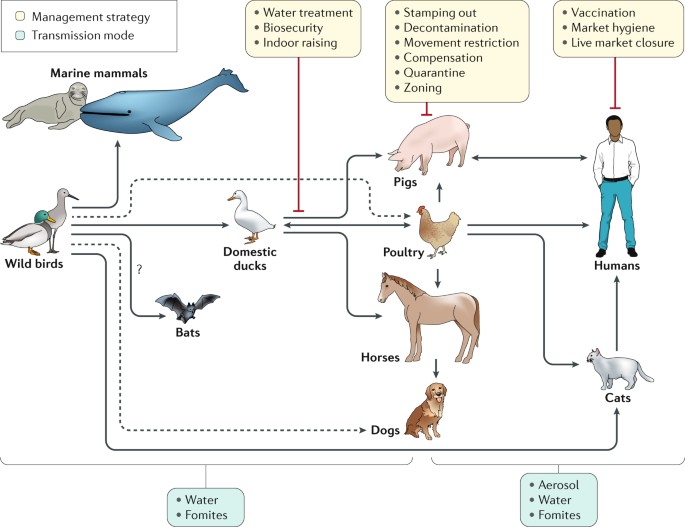
Influenza A viruses have been found in multiple species all seemingly derived from viral ancestors in wild birds, with the possible exception of bat influenza-like virus, which is of still uncertain origin. Influenza viruses from wild birds can spill over through water or fomites to marine mammals and to domestic free-range ducks. Transmissions to other avian species (for example, poultry) from domestic ducks or directly from wild birds can also occur from contaminated water. Transmission from ducks to other species occurs through ‘backyard’ farming, whereby the animals are raised together, and in live poultry and/or animal markets. Transmission from backyard to commercial farms can occur via lack of biosecurity and via spread through live markets 46 . Humans can be infected with poultry and swine influenza viruses through aerosols, fomites or contaminated water. However, in most instances these infections do not result in subsequent human-to-human transmission. Human-to-human transmission of seasonal or pandemic human viruses can be mediated by respiratory droplets, aerosols or self-inoculation after touching of fomites. Additional virus adaptations would be required for sustainable human-to-human transmission of animal influenza viruses. Other domestic animals known to be susceptible to influenza virus infections are dogs and cats. Dashed lines represent transmission that bypasses a domestic duck intermediate.
Influenza in pigs is a respiratory disease akin to influenza in humans, with high fever and pneumonia caused by influenza A H1N1, H3N2 and H1N2 subtypes 47 . Some strains of influenza A virus have been known to be transmitted by aerosol spread from humans to pigs and vice versa, including the pandemic 2009 influenza A H1N1 strain 48 and the influenza A H3N2 variant that transmits from pigs to children 49 . In horses, infection causes respiratory disease and is spread by aerosol; two lineages of influenza A H3N8 are primarily responsible. In 2004, the equine influenza A H3N8 strain spread to dogs 42 , 50 . In 2006 in Asia, an avian H3N2 influenza A virus was also detected as being transmitted to dogs 51 .
Box 2 Avian (poultry) influenza
The influenza viruses causing disease outbreaks in domestic poultry are the highly pathogenic avian influenza A (HPAI) H5N x and H7N x (where x = 1–9) and the low pathogenicity avian influenza A (LPAI) H6N1, H7N9 and H9N2 strains 228 . The major global problem for farmers of gallinaceous poultry (for example, chicken, quail and turkey) is the emergence of H5 and H7 HPAI viruses from LPAI precursors that must be reported to the World Organisation for Animal Health (OIE) and can result in trade embargoes. In poultry, the HPAI strains are transmitted both by aerosol and faecal contamination and cause systemic haemorrhagic disease and death 229 . Between 2003 and 2017, the HPAI H5N1 strain infected 860 humans and caused 545 deaths 230 . The LPAI H7N9 strain that emerged in China in 2013 causes asymptomatic infections of quail and chicken 231 , but from 2013 to 2017, a total of 1,564 laboratory-confirmed human infections with this virus were reported in China 232 . To complicate matters, some LPAI H7N9 viruses have recently become HPAI 233 . But perhaps the most insidious influenza virus in domestic poultry on the basis of its prevalence and ability to reassort is H9N2, which emerged in Asia in the early 1990s; this virus causes asymptomatic infection in poultry, was the donor of six internal RNA segments to both HPAI H5N1 and LPAI H7N9 and was occasionally transferred to pigs and humans 234 , 235 .
Cell entry and viral replication
At the cellular level, influenza virus replication (Fig. 4 ) mainly takes place in epithelial cells of the respiratory tract in humans and other mammals and in epithelial cells of the intestinal tract in birds. The cellular cycle of the virus starts with binding to the target cell. This binding is mediated by the viral HA (which binds to sialic acids present in the oligosaccharides of the glycoproteins at the cellular surface) and is also responsible for the haemagglutination caused by virus particles when incubated with red blood cells. HAs from human influenza viruses bind preferentially to sialic acids linked by an α2,6 linkage to the rest of the oligosaccharide, whereas those from most of the avian influenza viruses favour binding to α2,3-linked sialic acids, as these bonds are the most abundant sialic linkages in the epithelial cells of the human upper respiratory tract and of the avian intestinal tract, respectively 52 , 53 . After binding, the virus is internalized in an endosome, and the endosome is trafficked and acidified, which triggers a conformational change in the viral HA that induces the fusion of the viral envelope with that of the endosome. As the endosomal pH varies between host species, the pH stability of HA is one of the determinants of viral tropism.
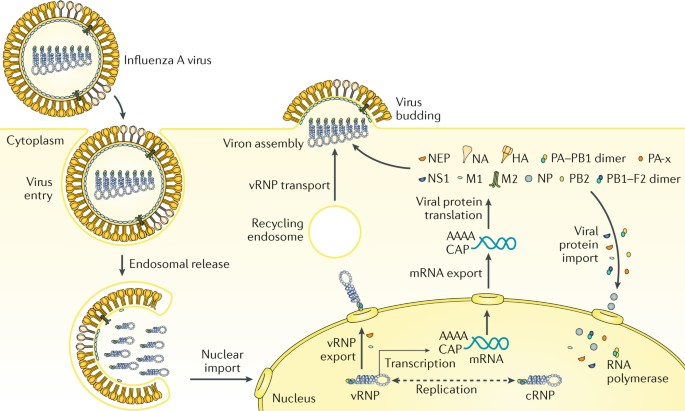
Influenza virus enters the cell by endosomal uptake and release, and its negative-sense genetic material in the form of viral ribonucleoproteins (vRNPs) is imported to the nucleus for transcription of mRNA and replication through a positive-sense complementary ribonucleoprotein (cRNP) intermediate. Viral mRNA is translated into viral proteins in the cytoplasm, and these are assembled into new virions together with the newly synthesized vRNPs. PB1–F2 is shown here as a dimer, but can also be multimeric. HA, haemagglutinin; M1, matrix protein; M2, membrane protein; NA, neuraminidase; NEP, nuclear export protein; NP, nucleoprotein; NS1, nonstructural protein; PB1, PB2 and PA, viral RNA polymerases.
Fusion results in the release of virus contents, namely, its genetic material in the form of eight viral ribonucleoproteins (vRNPs), into the cytoplasm. vRNPs are subsequently imported into the nucleus of the infected cells, where transcription and replication of the viral RNA takes place through the enzymatic activities of the viral polymerase complex attached to the vRNPs. Viral RNA replication occurs through a positive-sense intermediate, known as the complementary ribonucleoprotein (cRNP) complex. Viral RNA transcription results in positive-strand mRNAs that are capped and polyadenylated and exported into the cytoplasm for translation into viral proteins. Newly synthesized viral polymerases (PB1, PB2 and PA) and viral NP are imported to the nucleus to further increase the rate of viral RNA synthesis, whereas virus membrane proteins HA, NA and M2 traffic to and get inserted into the plasma membrane. Newly synthesized HA needs to be cleaved into HA1 and HA2 polypeptides by cellular proteases to be functional. The cleavage site of HA is responsible for the tissue tropism of the virus, with all influenza viruses having a cleavage site recognized by extracellular proteases present in respiratory and intestinal epithelial cells, except for the HPAI viruses, which contain a multibasic cleavage site in HA that is recognized by ubiquitous proteases. Viral nonstructural proteins NS1, PB1–F2 (which can be dimeric or multimeric) and PA-x regulate cellular processes to disarm host antiviral responses. Viral M1 and NEP localize to the nucleus at late stages of viral infection, bind to vRNPs and mediate their export to the cytoplasm, where, through interactions with the recycling endosome, they migrate to the plasma membrane and are bundled into the eight vRNPs. Budding of new virions takes place, resulting in the incorporation of the vRNPs into new virus particles with a membrane derived from the host plasma membrane and containing the viral transmembrane proteins. NA activity prevents non-productive binding of HA of new virions to receptors bearing sialic acid present in the viral glycoproteins and in the membrane of the infected cells, facilitating viral spread. Viral replication results in cell death with pathological implications. In addition, viral products induce a pro-inflammatory response that is responsible for the recruitment of innate and adaptive immune cells, which clear and eliminate the virus, but that in excess induces immunopathology and pneumonia.
Antigenic drift
Influenza viruses are capable of evading the antibody-mediated immunity induced during previous infections or vaccinations by gradually accumulating mutations in HA and NA 5 (Fig. 5 ). This process, known as antigenic drift, necessitates frequent updates of influenza vaccines to ensure sufficient antigenic relatedness between the vaccine and emerging virus variants. Because HA is currently the main component of the inactivated influenza vaccines, human and animal influenza virus surveillance programmes are in place to monitor antigenic changes of the virus, primarily using the haemagglutination inhibition assay (a surrogate assay to measure the inhibition of the interaction between HA and host cell receptors) and viral RNA sequence analyses 54 , 55 . Increased attention to antigenic drift of NA, measured using neuraminidase inhibition assays, could further increase the antigenic match between vaccines and circulating viruses 56 , 57 , 58 . In addition, antigenic drift of T cell epitopes has been observed but not as frequently as antibody-mediated drift 59 . Computational methods, so-called antigenic cartography, enable quantitative analyses and visualizations of haemagglutination inhibition assay data and neuraminidase inhibition assay data 60 .
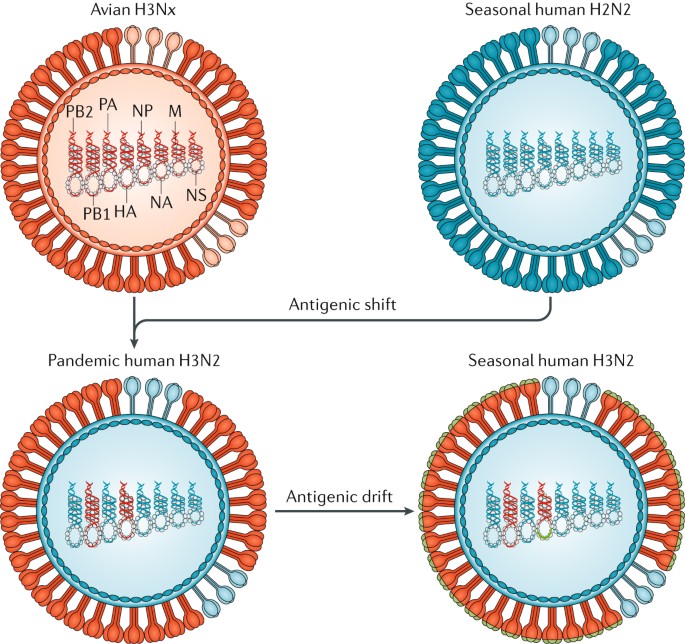
In 1968, co-infections between an avian influenza A H3N x (where x = 1–9) virus and the seasonal human influenza A H2N2 viruses resulted in the exchange of viral segments (reassortment) and the selection of the pandemic human influenza A H3N2 virus, with the RNA polymerase PB1 and haemagglutinin (HA) RNA segments derived from the avian virus and the rest of the segments derived from the human virus. The lack of pre-existing immunity to the antigenically novel H3 HA in humans facilitated human transmission. Similar reassortment processes have taken place during other influenza A virus pandemics. Once H3N2 became established in humans, the virus began to drift, as is the case with all other human seasonal influenza viruses. During drift, small antigenic changes in the HA protein generated by mutation are selected to increase immune evasion, although not as dramatically as during shift. M, RNA encoding M1, matrix protein, and M2, membrane protein; NA, RNA encoding neuraminidase; NP, RNA encoding nucleoprotein; NS1, RNA encoding nonstructural protein; PB2 and PA, RNA encoding RNA polymerases.
These methods to quantify antigenic drift of influenza virus strains, along with site-directed mutagenesis studies, have revealed that substantial antigenic drift can be caused by one or very few amino acid substitutions adjacent to the functional sites in the head (but not the stalk) of the HA protein 61 , 62 , 63 . The antigenic evolution of influenza viruses was shown to be more rapid in human influenza viruses than in swine and equine viruses, which is presumably related to the size, mixing and age-structure (that is, humans have a longer lifespan than swine and horses) of the host populations 60 , 63 , 64 . In wild birds, in which numerous avian influenza A virus HA and NA subtypes are circulating, within-subtype antigenic variation seems to be limited. However, upon introduction in poultry, antigenic variation within avian virus subtypes — perhaps related to a selective antibody pressure imposed by vaccine use — has been noted 62 .
Antigenic shift
In contrast to antigenic drift, antigenic shift refers to drastic changes in the antigenicity of the HA of circulating influenza A viruses; antigenic shift is associated with influenza A pandemics. The HA — and sometimes the NA — molecules of pandemic viruses are derived from antigenically diverse animal strains of influenza virus, which can be acquired by human influenza strains through reassortment (Fig. 5 ). Pandemic outbreaks are usually associated with the extinction of the previous circulating strains. However, in 1977, influenza A H1N1 viruses, not seen in humans since the 1957 influenza A H2N2 pandemic, started to co-circulate with influenza A H3N2 viruses 65 . The 2009 influenza A H1N1 pandemic was caused by an influenza A H1N1 virus that was antigenically very different from the seasonal influenza A H1N1 virus circulating at the time and resulted in the extinction of the previous influenza A H1N1 human lineage, but it did not result in the extinction of the influenza A H3N2 viruses. Since 2009, influenza A H3N2 and influenza A H1N1 viruses derived from the 2009 pandemic virus and two lineages of influenza B virus are co-circulating in humans.
The limited number of pandemic events that have happened makes it very difficult to predict the next pandemic. Human influenza A virus infections with antigenically diverse avian H5N1, avian H7N9, swine H3N2 and other animal influenza viruses are constantly detected in geographical regions where these strains are prevalent owing to the contact of infected poultry or swine with humans. However, no cases of sustained human-to-human transmission have been associated with these viruses, indicating that further adaptations need to take place for these viruses to become transmissible in humans. How feasible it is for these viruses to become adapted to human transmission and retain virulence is unclear. Only a few changes are needed for influenza A H5N1 viruses to become transmissible in ferrets, a host that is often used as an animal model of human influenza virus infection 66 , 67 , 68 .
Adaptations to new hosts
Aquatic waterfowl (for example, orders Anseriformes and Charadriiformes) host influenza A viruses of all the HA (H1–H16) and NA (N1–N9) subtypes and are believed to be the natural reservoirs of influenza viruses 69 . Some viruses, presumably from waterfowl, have adapted to other birds (Galliformes, such as chicken, quail and turkeys) and mammals (such as humans, swine, equine, dogs, mink and marine mammals) 70 . Such inter-species transmission and adaptation often involve viral genetic adaptations to the new host and can be attributed to viral RNA polymerases, which are error prone and have higher mutations rates than cellular DNA polymerases. The ensuing intra-host viral genetic diversity (sometimes referred to as viral quasi-species) results in a ‘swarm’ of viral mutants that facilitate adaptation to new selection pressures. Multiple genetic variants are transmitted between animals, and low-fitness variants can be maintained during transmission, presumably helped by the fitter variants within a host 71 . Maintenance of such genetic diversity in the virus population within one host would be potentially advantageous in adaptation following inter-species transmission events. In addition, the segmented RNA genome of the influenza virus enables viral genetic reassortment, providing an additional mechanism for genetic adaptation.
Adaptation to transmission in new hosts is usually multifactorial and could involve amino acid substitutions in the viral HA to optimize receptor binding 72 , to optimize HA and NA balance between their receptor binding and their receptor destroying activities, respectively 73 , and to enable HA to become activated at different pH values 74 . Additionally, changes in the viral RNA polymerase protein subunits can affect its activity in different hosts and affect the temperature sensitivity of the virus 75 , 76 (the temperature of the human upper respiratory tract is ~33 °C, which is lower than that of birds and swine); changes in NP can affect susceptibility to the host antiviral protein interferon-induced GTP-binding protein Mx1 (ref. 77 ), and changes in M1 and M2 proteins could alter viral morphology and facilitate respiratory transmission in new hosts 78 . Such functional changes that affect transmission could also be acquired through viral genetic reassortment, as occurred with the emergence of the human pandemics of 1957, 1968 and 2009 (ref. 79 ).
Genetic adaptations can occur at the interface between wild aquatic birds and domestic ducks or geese, between aquatic and terrestrial poultry (as occurred with the emergence of avian influenza A viruses H5N1, H7N9 and H10N8) or within swine (as occurred with the emergence of the North American triple reassortant influenza A viruses and of the 2009 pandemic influenza A H1N1 virus). Of the influenza A H3N8 and H7N7 viruses endemic in equine for many decades, the H7N7 viruses seem to have become extinct, whereas the H3N8 viruses have persisted and have been transmitted to dogs in the United States 50 , increasing the host range of H3N8 viruses. In another example, avian influenza A H3N2 viruses have adapted to dogs in Asia 51 . Recently, species of bat from Guatemala and Peru have been found to carry influenza A-like viruses with new subtypes of HA and NA (namely, H17, H18, N10 and N11), and further influenza virus diversity can be detected in bats 6 , 80 . Although bat influenza A-like H17N10 and H18N11 viruses are unable to reassort with conventional influenza A viruses, they can replicate in mammalian species other than bats, raising the possibility that they could become adapted to other mammals 81 .
Innate immune responses
Host innate immunity represents a crucial barrier that viruses need to overcome to replicate and propagate in new hosts, and influenza viruses dedicate several viral proteins to overcome these responses. For example, the NS1 protein of the virus is an RNA-binding protein that prevents the activation of cytoplasmic viral RNA sensors, such as retinoic acid-inducible gene I protein (RIG-I; also known as DDX58) 82 . RIG-I recognizes the influenza viral RNAs on the basis of the presence of a terminal 5′-triphosphate moiety and an adjacent double-stranded RNA structure formed by the 5′ and 3′ ends of the viral RNAs that is required for viral RNA replication and transcription 83 . NS1 binds to the host factors E3 ubiquitin-protein ligase TRIM21 and E3 ubiquitin-protein ligase RNF135 (also known as Riplet), which are required for RIG-I activation after viral RNA recognition, preventing a signalling cascade that otherwise leads to induction of interferon and interferon-inducible antiviral genes with antiviral activity, such as MX1 , EIF2AK2 (more commonly known as PKR ), OAS1 , IFITM family members and IFIT family members, among others 84 . Moreover, influenza A virus NS1 can also prevent host mRNA synthesis, processing and trafficking events, inhibiting host responses to viral infection 85 , 86 , 87 . Interferon signalling events mediated by the Janus kinase (JAK)–signal transducer and activator of transcription (STAT) pathway as well as the antiviral functions of several interferon-stimulated genes might also be targeted by NS1 to prevent the host antiviral response 88 , 89 , 90 .
In addition to NS1, several other influenza virus proteins can dampen the interferon-mediated antiviral response. PB1–F2, a viral nonstructural protein generated from an alternative open reading frame present in the PB1 RNA, suppresses the activation of mitochondrial antiviral-signalling protein (MAVS), an adaptor molecule located downstream of RIG-I and required for interferon induction 91 . PB2, a component of the viral polymerase, also seems to target MAVS activation 92 . PA-x, a recently discovered viral protein resulting from ribosomal frameshift of the viral RNA polymerase PA mRNA, suppresses host gene expression by virtue of its RNA endonuclease activity 93 . The multiple ways in which influenza virus counteracts the interferon-mediated antiviral response illustrate the importance of this host pathway.
Both type I interferons (namely, interferon-α (IFNα) and IFNβ) and type III interferons (namely, IFNλ) can inhibit viral replication in epithelial cells 94 . Tissue-resident macrophages and dendritic cells can also be infected by the virus, but viral production seems to be limited in these innate immune cells 95 . Nevertheless, exposure of these cells to viral infection results in their activation and the secretion of pro-inflammatory cytokines, leading to the recruitment of additional innate cells, such as natural killer cells and pro-inflammatory monocytes, to the lungs, where they can control viral infection by killing infected cells. Although these host responses to infection are crucial for final viral clearance and induction of adaptive immune responses, an exacerbated response can lead to immunopathology and severe disease. For instance, high levels of neutrophil extracellular traps (chromatin-based structures released from neutrophils) can lead to lung damage and severe influenza virus infection 96 . Therapies aimed to reduce this so-called cytokine storm might be beneficial for patients with severe influenza 97 .
Adaptive immune responses
T cell immunity.
Although mouse models are not considered optimal for analysing the pathogenesis of viral pneumonia, much of the research into how influenza virus-specific T cells work has used such systems. However, as the technology for monitoring T cell-mediated immunity in humans has improved, these basic insights have been validated 98 , 99 , 100 , 101 , 102 . In influenza virus infection, CD4 + T cell help is required for class-switching of antibodies and for optimal CD8 + T cell memory responses 103 , 104 . CD4 + T cell help is also required for peptide-based (and other) vaccines to promote optimal humoral and cellular immunity 105 , 106 . It is less clear whether CD4 + T cells are important effectors of direct influenza virus clearance that work via IFNγ production 99 , 104 , as occurs in infections with large DNA viruses such as herpesviruses.
With respect to CD8 + T cells, cytotoxic T lymphocyte (CTL) effectors are required for optimal influenza virus clearance 107 , 108 . In their absence, antibody-mediated mechanisms might also work, though less rapidly. Prominent antigenic components are the NP, matrix protein M1 and the viral polymerases 109 . Of particular interest is the fact that infected cells display viral peptides 8–12 amino acids in length that bind major histocompatibility complex (MHC; encoded by the HLA genes) class I glycoproteins to form the antigenic complex (epitope) recognized by T cell receptors on influenza virus-specific CTLs. These peptides are generally derived from components inside the virus that are less subject to antibody-selected antigenic drift and, therefore, are more conserved across distinct influenza virus strains and subtypes. Strong evidence supports the idea that established CTL memory directed towards conserved viral peptides presented by widely prevalent human MHC glycoproteins (for example, that are encoded by HLA-A2 ) can provide a measure of protection against a novel influenza A virus that crosses over from an avian reservoir to humans 101 (for example, the recent H7N9 cases 101 ) (Fig. 6 ). Furthermore, the increased influenza virus susceptibility of some indigenous populations (in Australia and Alaska) correlates with the relatively low frequency of such ‘protective’ HLA types within these populations 100 .
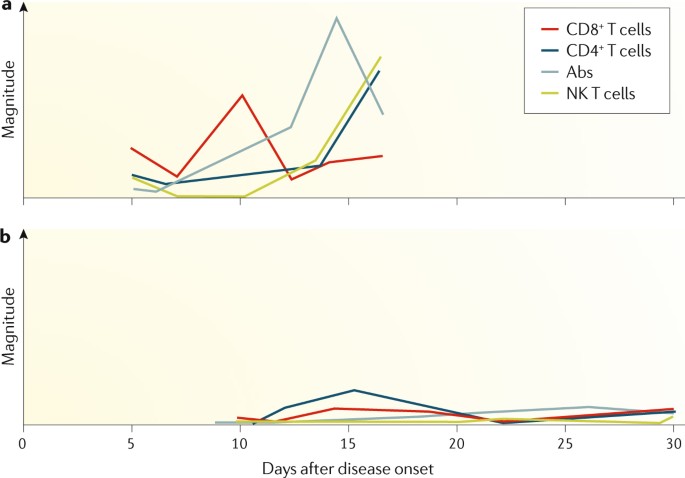
a | Patients who recovered early from severe influenza A H7N9 disease had rapid and prominent CD8 + cytotoxic T lymphocyte (CTL) recall responses. These early interferon-γ (IFNγ)-producing CTLs were most likely derived from pre-existing memory pools established after seasonal human influenza A virus infection, which then cross-reacted with H7N9. CTL responses were followed by antibody (Abs) responses 2–3 days later and by CD4 + T cells and natural killer (NK) cell responses 101 . b | By contrast, individuals who succumbed to severe influenza disease had minimal cellular or antibody immunity.
Given the unpredictability of influenza A virus pandemics and the inevitable lag phase in the production of vaccines (which are typically inactivated influenza virus vaccines), there is, of course, broad interest in possible strategies for cross-protective immunization. Live attenuated vaccines certainly have the potential to induce CD8 + T cell responses, but evidence to date suggests that they do not promote strong CTL memory 110 . Strategies aimed at maintaining large numbers of influenza virus-specific CTLs in the normal lung may both be impractical and have physiological consequences; instead, effector CTLs will likely need to be recalled from the recirculating ‘resting’ CTL memory pool. This approach can give more rapid virus clearance than is the case in a CTL-naive individual, resulting in milder disease outcomes.
Major questions are what constitutes optimal CTL memory, and how is this established. At a basic level, epigenetic profiles of the various CTL differentiation states (naive, effector and memory) are being defined to better understand the processes involved 111 . Also, as naive CTL sets are lost with age, priming influenza virus-specific CTLs early is important 112 . As for many other questions with CTLs, analysis of influenza virus infections will continue to inform the overall understanding of protective viral immunity.
B cell immunity
B cell responses are an integral part of the immune response to both influenza virus infection and influenza virus vaccines. Antibodies contribute substantially to the protection from infection, as shown by early experiments in which sera from an influenza virus-immune ferret could protect a naive ferret from influenza disease after challenge 113 . B cell responses mainly target viral HA and NA and, to a lesser degree, other viral proteins, including the NP and the matrix proteins 114 . Of note, discrete antigenic sites on the head domain of HA seem to be the preferential target of the antibody response 115 ; these sites are immunodominant over most other B cell epitopes of the virus 116 , 117 . However, the molecular and immunological reasons for antigenic immunodominance remain obscure. Antibodies that target the head domain of HA are typically neutralizing (via blockade of receptor binding) and show inhibitory activity in the haemagglutination inhibition assay. Importantly, the haemagglutination inhibition titre correlates (imperfectly) with protection and is a surrogate marker for vaccine efficacy that is globally accepted by regulatory agencies 118 .
Antibody responses to influenza virus infection in naive humans are typically robust and long lasting. For example, antibodies raised to strains encountered in childhood can usually be detected at reasonable levels in elderly individuals and might protect from virus variants with similar antigenicity encountered later in life 119 , 120 , 121 , 122 . However, antigenic drift particularly affects the HA head domain, which has high plasticity 123 , 124 . Over the course of a lifetime, individuals might be exposed to or infected by influenza viruses several times. Interestingly, the first infection in life and its immune imprinting might skew antibody responses to subsequent exposures. This phenomenon was originally described as the original antigenic sin 125 , but different aspects of it have been called antigenic seniority, antigenic imprinting and back-boosting 126 . Typically, exposure to a new virus variant (or subtype) might also induce antibodies to viruses encountered earlier in life, depending on the relatedness of the two antigens. An antibody response against a novel virus variant in adults might, therefore, be heavily influenced by the individual’s exposure history 117 , 127 , 128 , which is an issue for vaccine development.
In addition to neutralizing strain-specific antibodies, viral infection can also induce low-level humoral immune responses against conserved epitopes of viral proteins 126 . Among these epitopes are the membrane proximal stalk domain of HA 129 , 130 , the extracellular domain of the M2 protein 131 and conserved epitopes of NA 132 , 133 , 134 , 135 . The broad antibody responses against these epitopes might contribute to protection, and they often work through mechanisms that rely on crystallizable fragment (Fc)–Fc receptor (FcR) interactions (effector functions) or — in the case of anti-NA antibodies — NA inhibition 136 , 137 , 138 . However, the antibody responses induced by such conserved epitopes through infection or immunization (with current vaccines) are weak and reduce their potential contributions to protection in the general population.
As the mucosal surfaces of the respiratory tract are the main entry pathway for influenza viruses in humans, secreted antibodies play a major part in the prevention of infection. Key experiments in the guinea pig model of influenza have shown that only mucosal immune responses (including immunoglobulin A (IgA)) but not systemic immune responses can efficiently inhibit virus transmission 139 , 140 , which is important considering that influenza virus vaccines should prevent both disease in the host and transmission.
Diagnosis, prevention and screening
A diagnosis of influenza is made mostly on the basis of clinical presentation and epidemiological likelihood of infection. Initially, nonspecific symptoms predominate, including fever, chills or frank shaking, headaches, myalgia (muscle pain), malaise (discomfort) and anorexia. The onset of these symptoms is sudden, and respiratory symptoms, particularly a dry cough, sore or dry throat (possibly with hoarseness) and nasal obstruction and discharge, are usually also present. Cough is the most frequent respiratory symptom and can be accompanied by substernal discomfort or burning. Presentation in older adults and in individuals with compromised immune systems can initially be less dramatic, possibly because of a diminished cytokine response, but initial mild symptoms can progress to severe lower respiratory disease in these patients. The presentation can include fever, lassitude (lack of energy) and confusion without the characteristic respiratory complaints, which may not occur at all. Presentation in children can include febrile seizures but other prominent systemic complaints may not be present.
During seasonal influenza epidemics, diagnosis on the basis of clinical presentation has reasonable accuracy in previously healthy young and middle-aged adults, in whom the presence of fever with either cough or sore throat is associated with influenza virus infection in ~80% of patients 141 . However, as these symptoms are similar to those caused by other respiratory pathogens, which include parainfluenza virus, respiratory syncytial virus, human metapneumovirus, adenoviruses, rhinoviruses and coronaviruses, specific diagnostic tests are required to confirm infection by an influenza virus. Confirmation is important for reasons that include management of individual patients, public health responses and surveillance efforts 142 , 143 , 144 , but as patients do not always present to health-care facilities, the number of confirmed diagnoses is under-reported. The type of sample submitted for testing is usually a nasopharyngeal swab, a nasal wash or a combined throat and nasal swab; because the sensitivity correlates with viral load, samples obtained within 3 days of symptom onset are preferable.
As influenza-like illness can be caused by many viruses, the gold standard laboratory tests used for influenza are viral culture and reverse transcription PCR (RT-PCR) (Table 1 ). Virus culture is critical for detailed characterization of novel viruses, surveillance of sensitivity to antiviral drugs and monitoring of antigenic drift; it is time consuming, but the length of the test can be reduced if shell-vial co-cultures are used, which detect virus-positive cells by immunostaining before the presence of cytopathic effect 145 . By contrast, RT-PCR, which is a highly sensitive and specific molecular test, is quick, can be incorporated into multiplex assays and can be used to subtype viruses. The nucleic acid amplification tests are clearly superior to virus culture in both sensitivity and speed in the context of clinical management. For both viral culture and RT-PCR, the quality of the sample is important, as irregularities in handling can lead to degradation and false-negative results. The site sampled can also affect the sensitivity of the assay; nasopharyngeal swabs are preferred to throat swabs for detection of the virus during upper respiratory infection. As neither of these assays provides a point-of-care diagnosis, rapid antigen tests have been developed that are fast, can be run in a physician’s office and are less expensive, although low and variable sensitivity is a limitation. To overcome this limitation, a rapid molecular assay has been developed and approved by the US FDA, with results comparable to those of RT-PCR except in samples with low viral loads 146 . New point-of-care digital immunoassays also have superior sensitivity in detecting influenza A and influenza B virus infections compared with traditional rapid antigen tests 147 . Several recent point-of-care nucleic acid amplification tests with even higher sensitivities 147 are now approved in United States. Future developments might include the use of transcriptomic approaches to diagnose both the cause and the severity of respiratory illnesses, for example, the presence of secondary bacterial infections and/or inflammatory markers 148 .
After the causative agent of influenza was identified to be a virus in the 1930s, attempts were made during World War II to develop inactivated virus as a vaccine, which culminated in the licensing of the first influenza virus vaccine for the civilian population in 1945 in the United States. Unfortunately, it soon became clear that the vaccine did not protect well against new influenza virus strains. Specifically, the 1947 seasonal epidemic strain had changed sufficiently that the vaccines made with earlier circulating strains had lost their effectiveness 149 . All currently available influenza virus vaccines are injected intramuscularly, with the exception of the live attenuated influenza virus vaccines, which are administered intranasally.
Design and manufacture
Although antigenic shift and antigenic drift in influenza viruses are recognized problems, the procedure to manufacture influenza virus vaccines has remained largely unchanged for many decades. The manufacture of influenza virus vaccines involves growing the virus in embryonated chicken eggs and inactivation using formalin (or another alkylating reagent). In the 1970s, two changes were introduced. First, reassortment of up to six internal RNA segments of the high-yielding influenza A/Puerto Rico/8/1934 strain with RNA segments encoding HA and NA of the seasonal vaccine candidates improved the growth characteristics of the virus in embryonated eggs with obvious advantages for vaccine production (Fig. 7 ). Second, the whole virus is now subjected to treatment with ether or detergent to make subvirion (or split) preparations, which are much less reactogenic and are more tolerable for the patient (reviewed in ref. 150 ).
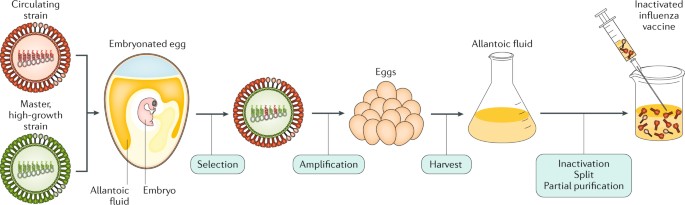
An antigenically representative circulating strain is reassorted with the high-growth influenza A/Puerto Rico/8/1934 (PR8) virus strain by co-infection in eggs, and a vaccine virus is selected with the high-growth properties of PR8 (conferred by its RNA segments encoding internal viral proteins) and the haemagglutinin (HA) and neuraminidase (NA) derived from the circulating strain. The vaccine virus is amplified in eggs, and the allantoic fluid from the infected eggs containing high titres of the virus is harvested; the virus is inactivated, treated for splitting of individual viral components and subjected to partial purification to enrich for the HA (and NA) viral components in the final injectable vaccine.
In 2003, MedImmune (Gaithersburg, Maryland, USA) introduced the first live attenuated influenza virus vaccine (LAIV) licensed in the United States. This vaccine is based on the backbones of the influenza A/Ann Arbor/6/1960 and influenza B/Ann Arbor/1/1966 strains, through the passaging of the viruses at low temperature (25 °C). Cold-adapted influenza virus vaccines based on the same principle and developed by the Institute of Experimental Medicine (IEM, Saint Petersburg, Russia) have been successfully used in Russia since 1987 (ref. 151 ). Seasonal vaccines are made by reassortment of the six internal RNA segments of the cold-adapted strain with the HA and NA RNA segments of the influenza virus strains specified by the health authorities for inclusion in the seasonal vaccines. Vaccine strain selection for both inactivated vaccines and LAIVs is based on the determination of the prevalence of recent human circulating strains by multiple laboratories engaged in a WHO-sponsored influenza surveillance programme (Box 3 ). LAIVs seem to have advantages in inducing mucosal and more broadly protective immune responses in infants and children than inactivated influenza virus preparations (reviewed in ref. 150 ). However, studies with LAIVs in the United States have shown poor efficacy against the influenza A H1N1 component 152 .
Tissue culture cell-based substrates other than embryonated eggs have the advantage of not becoming limiting in cases when vaccine production needs to increase, such as during pandemic outbreaks. Cell-grown influenza virus vaccines based on Madin–Darby canine kidney (MDCK) and Vero cells (from kidney epithelial cells of monkeys) were licensed in Europe in 2007 and 2009, respectively. However, production of the Vero-cell vaccine has been stopped, as the company that developed them ceased its vaccine production portfolio, and the FDA only approved the first MDCK-grown influenza virus vaccine in 2012 (ref. 153 ). This approval was a major accomplishment after exhaustive studies to demonstrate that the cells did not contain adventitious viruses that were due to known naturally occurring dog viruses, such as oncogenic papilloma and retroviruses.
Another breakthrough for influenza vaccines came in 2013, when the FDA licensed Flublok (Protein Sciences Corporation, Meriden, Connecticut, USA), which is a product made exclusively using recombinant DNA technologies. For the quadrivalent product, four different baculoviruses expressing the HA of two influenza A viruses and the HA of two influenza B viruses are used to infect continuous insect cell lines; the HA proteins are then extracted and purified from the infected cells — a process that completely avoids the use of influenza viruses and of embryonated eggs. Thus, there is now a faster start-up possible for vaccine manufacturing in cases of vaccine shortages or the emergence of novel pandemic strains. Also, there is no need for time-consuming adaptations of the vaccine strains to increase yields in embryonated eggs or in tissue culture, as the recombinant baculoviruses express different HAs at comparable levels in insect cells 154 .
Addition of adjuvants to inactivated influenza vaccines can improve vaccine efficacy and the breadth and duration of protection and can have dose-sparing benefits in case of years with vaccine shortages. Indeed, in November 2009, German regulatory authorities approved an MF59-adjuvanted, MDCK-grown influenza A H1N1 pandemic vaccine 155 . MF59 is a squalene-based adjuvant that increases the immunogenicity of protein-based vaccines, and MF59-adjuvanted inactivated influenza vaccines are now approved for use in people >65 years of age, in whom unadjuvanted inactivated influenza vaccines perform poorly. In 2013, the FDA approved an adjuvant influenza vaccine for the prevention of pandemic influenza A H5N1. This vaccine is for use in people >18 years of age who are at increased risk of infection with avian influenza A H5N1 viruses. The adjuvant used, AS03, similar to MF59, is a squalene-based oil-in-water emulsion that is combined with the H5N1 component before intramuscular injection. AS03-adjuvanted pandemic influenza virus vaccines have previously been used in Europe during the 2009 influenza A H1N1 pandemic, where they received close attention owing to several reported cases of vaccine-associated narcolepsy 156 , a chronic neurological disorder characterized by excessive daytime sleepiness. Contributory roles of the pandemic virus or the vaccine antigens in these adverse events could not be excluded.
To increase the poor immunogenicity of inactivated influenza vaccines in elderly individuals, an unadjuvanted high-dose vaccine has also been developed and is proven to decrease severe influenza virus infection outcomes compared with standard-dose vaccines 157 . In addition, some available influenza vaccines are now tetravalent, incorporating both influenza B virus lineages. Thus, the variety of approved influenza virus vaccines has been dramatically increased in recent decades globally. However, all these available seasonal influenza virus vaccines still necessitate annual reformulations to match antigenically circulating vaccines (Box 3 ). In addition, the short duration of immunity is another major shortcoming of the current vaccines.
Reverse genetics technologies to generate recombinant influenza viruses with defined genetic sequences from plasmid DNA were introduced in 1990 (ref. 158 ). These techniques should improve both live and inactivated influenza virus vaccine manufacturing by creating vaccine backbones with increased replication properties and stability for use in embryonated eggs or in tissue culture. Other approaches are aimed at inducing protective immune responses against the aforementioned conserved epitopes of various viral proteins. Novel and safe adjuvants based on Toll-like receptor-mediated immune stimulation and novel modes of delivery may dramatically change the vaccine field in the future. The ultimate goal of these approaches is to make an influenza virus vaccine that provides lifetime (or at least multi-year) protection, protects against all influenza antigenic variants and establishes a degree of herd immunity. Indeed, consensus is that influenza virus vaccines could and should be improved and that efforts to design a long-lasting universal influenza virus vaccine effective against different antigenic variants are worthwhile 159 , 160 .
Box 3 Seasonal influenza vaccine strain identification
Year-round surveillance for human influenza is conducted by >100 designated national influenza centres around the world. The laboratories send isolated viruses for genetic and antigenic characterization to five WHO Collaborating Centres for Reference and Research on Influenza, which are located in the United States, the United Kingdom, Australia, Japan and China. A WHO committee reviews the results of surveillance and laboratory studies twice per year and makes recommendations on the composition of the influenza vaccine on the basis of the use of antigenically matched viruses with those that are expected to be highly prevalent in the next season. Each country then makes their own decision about which viruses should be included in influenza vaccines licensed in their country.
Schedules and vaccination programmes
Vaccine recommendations vary from country to country, with some recommending vaccination in only the high-risk groups and contacts. Currently, the public health authorities in the United States and the American Academy of Pediatrics recommend yearly vaccination with seasonal influenza virus vaccine to all people >6 months of age 161 . Stronger recommendations are given to individuals in high-risk groups for severe influenza and to caregivers and contacts of those at high risk. The WHO recommends seasonal influenza vaccination to pregnant women (highest priority) and, in no particular order of priority, to children 6 months to 5 years of age, elderly individuals (>65 years old), individuals with specific chronic medical conditions and health-care workers.
In the absence of a universal vaccine that covers all possible strains and subtypes of influenza virus, many countries have implemented stockpiling vaccine programmes to store vaccines against influenza virus strains with pandemic potential for emergency first responders to alleviate the problem imposed by the rapid spread of pandemic influenza. Although preparing for potential pandemic threats is sound public health policy, it is not compelling to assume that the influenza A H5N1 viruses (or the more recently detected H7N9 virus (March 2013)) are pandemic precursor strains because they are associated with the largest numbers of human infections among animal influenza A virus strains. First, over the past 130 years, only viruses expressing an H1, H2 or H3 HA have been responsible for seasonal or pandemic strains. Avian H5N1 and H7N9 viruses have most likely circulated for at least as long without spilling over into humans and starting a new pandemic 162 . Accordingly, these ‘avian’ HA influenza viruses are unlikely to easily transmit from person to person. Second, the high case fatality rates cited by the WHO for H5N1 and H7N9 viruses in humans seem to be an artefact of the way they were calculated (that is, counting only patients who were hospitalized as having been infected by these viruses); many more people seem to have been infected by these viruses without severe outcomes 163 , 164 . Additionally, severe disease only results from exposure to large quantities of the virus, which can occur when people are in direct contact with diseased poultry. However, transmission of seasonal or pandemic influenza viruses requires only small quantities of virus (estimates vary between 2–25 virions 165 and 50–400 virions 166 ) to cause severe disease and transmission.
Efficacy and effectiveness
Despite the availability of seasonal and pandemic influenza vaccines, debate is ongoing as to the efficacy (as measured by randomized controlled trials) and effectiveness (as measured by observational studies involving vaccinated and unvaccinated individuals) of these vaccines 167 . Although different methodologies, the variability of the virulence of different seasonal strains, the fit or matching of the vaccine with the circulating strains and age differences of different cohorts can make interpretation of results between studies difficult, most studies find a positive effect of vaccination on the overall health of vaccinated individuals 168 , 169 . Nevertheless, many challenges still remain, such as the documented lack of efficacy of LAIVs in the United States in the past years 170 , the possible reduced vaccine efficacy with repeated annual immunizations 171 and the problems associated with vaccine mismatches 172 . Assays to monitor antigenicity with respect to prior viruses are performed on a regular basis, generally by government laboratories. Antigenicity of a virus is characterized by infecting naive ferrets with the cultured virus and collecting the sera 2–3 weeks later; the ability of the sera to inhibit haemagglutination compared against the current vaccine is monitored. Sera from an antigenically novel virus will show reduced haemagglutination inhibitory activity compared with previous virus strains, indicating antigenic drift and a potential vaccine mismatch.
Together with vaccines, antiviral drugs play a vital part in the prevention and treatment of influenza virus infection and disease. In a normal influenza season, antiviral drugs are primarily used for treating patients who are severely ill, particularly those who have a compromised immune system. In a pandemic setting, especially in the period before a vaccine is available, antiviral drugs are essential for both treating patients who have been infected and preventing infection in individuals who have been exposed. Two classes of drugs are currently approved for influenza, namely, the adamantanes and the NA inhibitors.
Amantadine and rimantadine are both orally administered drugs that target the M2 ion channel of influenza A viruses. However, these drugs are globally no longer recommended for clinical use because of widespread resistance among circulating influenza A viruses (see below). By contrast, NA inhibitors target the enzymatic activity of the viral NA protein. Oseltamivir is delivered orally as the prodrug oseltamivir phosphate, which is converted to its active carboxylate form in the liver; zanamivir is inhaled as a powder (limiting its use in those with underlying respiratory problems); and peramivir is administered intravenously, which is important for patients who have been hospitalized 173 . All three drugs have received approval in the United States, Europe, Canada, Australia, Japan, Taiwan and Korea and act by mimicking the binding of sialic acid in the active site of NA on influenza A and influenza B viruses 174 .
Oseltamivir and zanamivir are effective for prophylaxis and post-exposure prophylaxis in individuals 175 . However, the randomized controlled trials of these drugs were conducted in patients with uncomplicated influenza; accordingly, observational data must be used to assess effectiveness in patients who are severely ill and have been hospitalized, in whom the need is arguably greater. Despite this limitation, study results consistently report improved outcomes associated with NA inhibitor use, including reduced risk of pneumonia and hospitalization, and reduced risk of mortality in patients who have been hospitalized 176 , 177 , 178 . Another consistent finding is that better results are obtained with early administration of NA inhibitors (within 2 days of symptom onset), although later administration can still be beneficial in severe cases.
The emergence of drug-resistant viruses is a major challenge for the antiviral field. Adamantane resistance (conferred by an S31N mutation in the RNA segment encoding M2) first emerged in influenza A H3N2 viruses in 2003 and became prevalent worldwide by 2008 (ref. 179 ). The pandemic 2009 influenza A H1N1 virus, which is presently circulating as a seasonal H1N1 virus, is also resistant to the adamantanes, as its M RNA segment (Fig. 1 ) was inherited from a virus that carried the S31N mutation 180 . Thus, since 2009, only the NA inhibitors have been able to provide protection, with the currently circulating human influenza A and influenza B viruses being generally sensitive to oseltamivir and zanamivir (Table 2 ). However, emergence of oseltamivir resistance is of particular concern for influenza A H1N1 viruses because it has happened in the past; in 2007, resistant influenza A H1N1 viruses began to circulate and quickly became dominant by the 2008–2009 season 181 . The resistant phenotype is associated with an H275Y mutation in the RNA segment encoding NA, and studies have shown that, in the background of other permissive mutations, H275Y-mutant viruses do not display any fitness deficits, which explains their ability to circulate so readily 182 , 183 . Of note, these viruses remain sensitive to zanamivir. Perhaps fortuitously, the oseltamivir-resistant seasonal influenza A H1N1 virus was displaced by the introduction of the oseltamivir-sensitive pandemic influenza A H1N1 virus in 2009. Since then, surveillance efforts have monitored NA inhibitor sensitivity closely and, although oseltamivir-resistant isolates of the 2009 influenza A H1N1 virus have been detected, these are primarily (but not exclusively) from patients undergoing therapy 184 . Susceptibility to NA inhibitor is assessed by measuring NA enzyme inhibition in a functional assay using cultured virus. Alternatively, RT-PCR capable of detecting single nucleotide polymorphisms (or RT-PCR followed by sequencing) is used to detect known resistance mutations in NA 185 , but the functional assay may be required if novel mutations are present.
There is a higher prevalence of resistant virus in children and individuals who are immunocompromised, which is believed to be related to higher viral loads and prolonged virus shedding in these individuals 186 , 187 . Also, patients who have been hospitalized are more likely to be treated for longer periods, which will increase pressure on the virus and the likelihood that a resistant strain will emerge. Although this outcome may be predictable, oseltamivir resistance arose in 2007 seemingly in the absence of drug pressure; given the correct background of secondary mutations, the same outcome is a possibility for the current influenza A H1N1 virus 188 . Oseltamivir resistance among influenza A H3N2 viruses and influenza B viruses has also been reported, albeit at a much lower frequency than for influenza A H1N1 (ref. 189 ).
The elimination of adamantanes as clinical therapy combined with the concerns over increasing oseltamivir resistance illustrates the need for new influenza antiviral drugs. Several candidates are now in clinical trial, including additional NA inhibitors that can be delivered intravenously, such as intravenous formulations of zanamivir. Another NA inhibitor, laninamivir, has been approved for use in Japan; this drug is inhaled and long-acting and requires less frequent administration than oseltamivir or zanamivir 190 . Ideally, future influenza therapies will involve a cocktail of drugs with different mechanisms that should increase the barrier to resistance. Thus, drugs other than NA inhibitors are being clinically tested; within this pipeline are favipiravir (which is a polymerase inhibitor for several RNA viruses 191 ), pimodivir (which prevents the cap binding required for viral RNA transcription 192 ), baloxavir marboxil (an inhibitor of the cap-dependent endonuclease activity of the influenza viral polymerase 193 ) and nitazoxanide (an inhibitor of HA maturation 194 ). Baloxavir marboxil received approval in Japan in 2018. Favipiravir has also been approved in Japan since 2014, but only for release from government stockpile in the event of a novel strain being unresponsive to current agents. Treatment of patients who have been hospitalized with immune plasma 195 or with hyperimmune immunoglobulin (pooled from plasma of donors with high titres of anti-influenza IgG) 196 is also being clinically evaluated. In addition, several monoclonal antibodies against the conserved region of the HA stalk are also under clinical development for the treatment of severe influenza infection 197 . Many more candidates are in preclinical development and will hopefully lead to improved options for influenza therapy in the future.
Non-pharmaceutical prevention strategies
In addition to vaccines and antiviral drugs, non-pharmaceutical interventions can help to slow the spread of influenza illness. These interventions include personal measures, such as hand washing and using alcohol-based sanitizers 198 , covering coughs and/or the nose and mouth when sick and staying at home when sick. Additionally, social distancing by closures of schools and places of gathering, quarantine measures and frequent cleaning of potentially virus-contaminated surfaces, such as door knobs, can also slow the spread. Mathematical modelling studies suggest that non-pharmaceutical interventions have a substantial effect on lowering the attack rate of pandemic influenza before vaccines are available 199 .
Management of acute influenza has traditionally relied on supportive measures such as control of fever, symptomatic treatment, rehydration and treatment of complications, such as bacterial pneumonia, if they occur. Supportive care is still the mainstay of management of uncomplicated influenza. However, as described above, several antiviral agents have been developed for influenza or are in active clinical development. Furthermore, given the natural animal reservoirs of influenza (Fig. 3 ), prevention of transmission from these reservoirs to humans is key. Water treatment, indoor raising and biosecurity are the primary management goals; these strategies are followed by quarantine, stamping out and the ultimate use of animal vaccination.
Human influenza
People with clinical symptoms of influenza virus infection should remain home and avoid contact with non-infected people until symptoms alleviate. As the level of virus replication is correlated with the clinical severity of illness, strategies to limit viral replication with antiviral agents would be expected to be effective in acute influenza illness. However, adults with an intact immune system who have had previous influenza infections rapidly limit the replication of these viruses without intervention, so the opportunity to attenuate viral replication with antiviral agents is limited. Effective use of antivirals for seasonal influenza in otherwise healthy adults requires early initiation of therapy, generally within the first 48 hours of symptom onset. However, decisions regarding treatment should consider the somewhat small benefit demonstrated in clinical trials and the overall low risk of complications 177 . The benefits of later therapy in otherwise uncomplicated influenza are unclear.
Patients with underlying immune defects, those with more-severe disease or those who have a higher risk of influenza complications 200 may benefit from antiviral administration, even if initiated after 48 hours of symptom onset 201 if they are still shedding virus. Antiviral therapy is strongly considered if the illness is progressing and symptoms are worsening, particularly if the patient continues to test positive for influenza virus. Indeed, prescription of antivirals for high-risk outpatients with acute respiratory illness is beneficial 202 . Furthermore, a diagnosis of influenza in patients who have been hospitalized with community-acquired pneumonia should be confirmed to increase antiviral treatment rates in patients 203 . Studies suggest that, since the 2009 pandemic, the use of antivirals in patients who have been hospitalized with influenza has increased 204 , which is an improvement. Complications of influenza virus infection might require specific treatments, as is the case with secondary bacterial pneumonia, which should be treated with appropriate antibiotics.
Finally, consideration should also be given to the possible use of immunotherapy aimed at controlling exacerbated pro-inflammatory responses in patients with severe symptoms, especially in combination with antiviral therapy. This approach is supported by recent clinical studies suggesting beneficial effects when inhibitors of cytokine production and leukocyte recruitment were added to NA inhibitor therapy 205 .
Animal influenza
As influenza in wild and domestic waterfowl is primarily spread through contaminated water, water treatment (with, for example, chlorine and ultraviolet light), biosecurity and indoor raising of animals are the main strategies to prevent transmission. Biosecurity includes placing poultry houses and piggeries on high ground away from high-density and backyard farms, live poultry markets and bodies of water to minimize contact with waterfowl and passerine birds. Risk assessment and training of personnel to change outdoor clothing, disinfect foot baths and treat food and water are also important aspects of biosecurity.
After a novel influenza virus has breached biosecurity, a strategy that includes rapid detection and stamping out is optimal 206 . Stamping out is easier when animals display signs of severe infection, but these can go unnoticed when LPAI infection occurs. After initial emergence of HPAI H5 or H7 in poultry, quarantine, culling, decontamination and compensation of farmers with restricted zones of movement are imposed. This classic strategy was successful in the majority of Eurasian countries to which HPAI H5N1 spread 206 . However, late detection and widespread dissemination of HPAI H5N1 in China, Indonesia, Vietnam, Bangladesh and Egypt resulted in endemicity, making virus eradication difficult 206 . One difficulty in eradication is inadequate provision of compensation to farmers, who will be incentivized to hide infected animals.
Poultry vaccines use inactivated whole virus in oil emulsion, recombinant DNA or vectorized vaccines. These vaccines can be highly effective at reducing disease signs and inter-species spread, but they do not induce sterilizing immunity and have not resulted in eradication from endemic countries. Poultry vaccination is highly contentious; both the United Nations Food and Agriculture Organization (FAO) and the World Organisation for Animal Health (OIE) state that long-term vaccination without additional measures is not recommended 207 . Although poultry vaccination dramatically reduced human cases of H5N1 in Vietnam in 2006, it did not eradicate the virus and likely contributed to the antigenic diversity. On the other hand, vaccination used in combination with unvaccinated sentinel chickens and stamping out can achieve control and local eradication 208 .
Live poultry markets have been recognized as a breeding ground of influenza viruses. Changes to live poultry marketing achieved by implementing so-called clean days (that is, days when stalls are cleared of unsold poultry and disinfected) and no carry-over (that is, no infectious material remains when the next live birds arrive after disinfection) of live poultry improved hygiene and reduced virus spread 209 , 210 . A definitive solution would be the closing of all live poultry markets. Indeed, market closure contributed to the reduction of the spread of HPAI H5N1 in Hong Kong and H7N9 transmissions to humans in China 211 . Such changes without public training risk poultry smuggling from unregulated sources.
The management of influenza in pigs or horses is dependent on the use of vaccines. In pigs, influenza is a minor disease problem for which biosecurity is paramount, and vaccination uses primarily whole inactivated virus in oil emulsion. In horses, the problem with vaccination is the short-term protection offered, but antigenically matched adjuvanted inactivated vaccines and LAIVs are efficacious 212 .
Quality of life
Uncomplicated influenza in otherwise healthy adults and children is rarely fatal but can be highly disruptive and lead to substantial absences from work or school. Recovery can be prolonged, with persistent fatigue and malaise that can last for several weeks after recovery from the other symptoms. Influenza is associated with decreased job performance in adults 213 and reduced levels of independent functioning in older adults 214 . As a result, the loss of productivity by affected individuals contributes substantially to the overall economic effect of influenza. Mild abnormalities in pulmonary function are frequently experienced by healthy adults and also can take several weeks to return to normal. In a 2007 study, direct yearly medical costs associated with influenza in the United States were estimated to be approximately $10.4 billion, whereas projected lost earnings owing to illness and loss of life were estimated at $16.3 billion 215 .
One of the unique features of influenza is the somewhat high frequency with which it is associated with pulmonary complications such as pneumonia, including primary influenza virus pneumonia and secondary bacterial pneumonia. However, during an outbreak of influenza, many patients do not clearly fit into either of these presentations and may instead have mixed viral and bacterial pneumonia. Primary influenza viral pneumonia was well documented in the 1957–1958 pandemic, although many deaths of young healthy adults in the 1918–1919 pandemic were also the result of primary pneumonia 216 . Primary pneumonia occurs predominantly among patients with influenza virus infection who also have underlying cardiovascular disease, especially rheumatic heart disease with mitral stenosis, but may occur in a variety of settings. Primary viral influenza pneumonia is characterized by a rapid progression of fever, cough, dyspnoea (laboured breathing) and cyanosis (low oxygen levels resulting in bluish skin), with chest radiographs often revealing bilateral findings consistent with acute respiratory distress syndrome.
The classic presentation of secondary bacterial pneumonia is that of typical influenza illness followed by a period of improvement, which is then followed by recurrent fever, cough, sputum production and consolidation (fluid build-up in the lungs). Indeed, bacterial co-infections were present at high frequency during the 1918 pandemic 217 , 218 . The two pathogens that are currently most commonly associated with influenza are Streptococcus pneumoniae and Staphylococcus aureus 219 . The mechanisms by which influenza infection uniquely predisposes to bacterial superinfection are being actively investigated. In addition to pneumonia, multiple other pulmonary complications of influenza are recognized, including bronchiolitis (inflammation of the bronchioles) and croup (laryngotracheobronchitis) and exacerbations of chronic bronchitis or asthma.
Non-respiratory complications have also been reported in association with acute influenza infection 220 . Myositis, with tender leg muscles and elevated serum creatine phosphokinase, has been reported primarily in children 221 . Both myocarditis and pericarditis have been rarely associated with influenza A or influenza B virus infection. Because influenza may predispose to superinfection with S. aureus , toxic-shock syndrome, a clinical illness characterized by hypotension, fever, erythroderma (reddening of the skin), desquamation (skin peeling) and multisystem involvement, has also been observed following influenza, primarily in children 222 . Guillain–Barré syndrome has been reported to occur after influenza A infection, as it has after numerous other infections, but no definite aetiological relationship has been established. In addition, cases of transverse myelitis (inflammation and myelin loss around the spinal cord) and encephalopathy have occurred rarely in association with influenza 223 . An epidemic of an atypical form of encephalitis known as encephalitis lethargica (characterized by headache, drowsiness and coma) spread around the world after the 1918 influenza pandemic and then practically disappeared in 1930, suggesting that this was a sequela caused by the pandemic 224 . However, as acute influenza is a common disease event, a temporal association with other disease entities does not always indicate a cause and effect relationship.
A better understanding of the ecological, viral and host determinants permitting inter-species transmission and adaptation of influenza A viruses to new hosts may facilitate earlier recognition and evidence-based risk assessment of influenza A viruses that may threaten either animal or human health. Better understanding will also provide the basis for implementing interventions aimed at preventing emergence at the source. For example, the understanding that domestic ducks serve as an interface between the gene pools of wild aquatic birds and terrestrial poultry supports the idea that measures to reduce exposure of domestic ducks to wild birds are warranted and the idea that separation of aquatic and terrestrial poultry through wholesale and retail live poultry marketing chains in Asia is also valid.
Despite the availability of vaccines and antiviral agents, influenza continues to be the most important cause of viral respiratory disease associated with human hospitalizations and deaths. We should not accept such a burden for, in theory, a vaccine-preventable disease. The current seasonal human influenza vaccine necessitates annual administration, is not optimal and varies in effectiveness from year to year. Pandemic years and seasonal years when the vaccine is mismatched are especially concerning. Mismatches in vaccine occur not only when the most prevalent antigenic strains are missed but also when the vaccine becomes antigenically mismatched owing to adaptations to egg culture. For example, the 2016–2017 seasonal influenza vaccine produced in eggs lacked a glycosylation site present in the influenza A H3N2 circulating strains. This change was due to egg adaptation and led to an alteration of the antigenicity of the influenza vaccine component 225 . Tissue culture to manufacture influenza virus vaccines might be less prone to HA adaptations, but it does not completely prevent them. In this respect, the aforementioned recombinant baculovirus-produced influenza vaccines have an advantage in that they do not select for HA adaptive mutations.
The management of both animal and human influenza by vaccination is less than optimal. The development of a highly efficacious universal vaccine and genetically resistant animals (that have been engineered or that have evolved to be so) are future options. Even the development of new vaccines that provide more-durable and more-effective protection against circulating viruses would represent major improvements. An exhaustive exploration in humans of adjuvants, live attenuated approaches, vaccine platforms and antigen design is required to identify the best approaches to improve immunogenicity without compromising safety. Although clinical studies to demonstrate influenza vaccine efficacy are costly and challenging, it is imperative that we apply modern science and technology to improve the existing influenza virus vaccines. In this respect, more studies are needed to improve our understanding of the correlates of immune protection in humans and to develop immunological, molecular and genetic tools for the better use of animal models of human influenza. Moreover, although experimental human challenges have been developed to quantify protective responses in patients, these are not yet optimal, as in general they require infection protocols that do not mimic natural infections 226 . The same principles apply to the development of new influenza antiviral agents. Finally, a better understanding of the risk factors, both in the host as well as in the virus, that predispose to more-severe influenza is also needed.
One hundred years after the devastating 1918 influenza pandemic, we still are not able to optimally prevent and treat influenza. However, substantial scientific advances have been made, such that our understanding of the molecular biology, immunology, host response, tropism and pathophysiology of influenza has improved, as has our understanding of the influenza virus itself. We hope that in the next decade, productive partnerships will be established between government, philanthropic agencies, academia and industry to facilitate more scientific discoveries and that these discoveries will translate to improvements in treatment and prevention of influenza. In this respect, the US National Institute of Allergy and Infectious Diseases is, for example, launching new research initiatives and strengthening existing research programmes to support inter-collaborative research to accelerate the development of improved influenza vaccines that provide durable protection from seasonal and pandemic influenza virus strains 227 — a promising step towards the generation of a universal influenza vaccine. Likely, the induction in the respiratory mucosa of effective and durable antibody responses against conserved conformational epitopes in viral HA and NA and T cell responses against conserved viral protein epitopes representing the diversity of human HLA will be required for the development of such a universal influenza virus vaccine.
Sellers, S. A., Hagan, R. S., Hayden, F. G. & Fischer, W. A. 2nd. The hidden burden of influenza: A review of the extra-pulmonary complications of influenza infection. Influenza Other Respir. Viruses 11 , 372–393 (2017).
PubMed PubMed Central Google Scholar
Kwong, J. C. et al. Acute myocardial infarction after laboratory-confirmed influenza infection. N. Engl. J. Med. 378 , 345–353 (2018).
PubMed Google Scholar
Matsuzaki, Y. et al. Clinical features of influenza C virus infection in children. J. Infect. Dis. 193 , 1229–1235 (2006).
Olsen, B. et al. Global patterns of influenza a virus in wild birds. Science 312 , 384–388 (2006).
CAS PubMed Google Scholar
Webster, R. G., Bean, W. J., Gorman, O. T., Chambers, T. M. & Kawaoka, Y. Evolution and ecology of influenza A viruses. Microbiol. Rev. 56 , 152–179 (1992).
CAS PubMed PubMed Central Google Scholar
Tong, S. et al. A distinct lineage of influenza A virus from bats. Proc. Natl Acad. Sci. USA 109 , 4269–4274 (2012).
Ma, W., García-Sastre, A. & Schwemmle, M. Expected and unexpected features of the newly discovered bat influenza A-like viruses. PLOS Pathog. 11 , e1004819 (2015).
Google Scholar
Fouchier, R. A. et al. Characterization of a novel influenza A virus hemagglutinin subtype (H16) obtained from black-headed gulls. J. Virol. 79 , 2814–2822 (2005).
Osterhaus, A. D., Rimmelzwaan, G. F., Martina, B. E., Bestebroer, T. M. & Fouchier, R. A. Influenza B virus in seals. Science 288 , 1051–1053 (2000).
Guo, Y. J., Jin, F. G., Wang, P., Wang, M. & Zhu, J. M. Isolation of influenza C virus from pigs and experimental infection of pigs with influenza C virus. J. Gen. Virol. 64 , 177–182 (1983).
Hause, B. M. et al. Isolation of a novel swine influenza virus from Oklahoma in 2011 which is distantly related to human influenza C viruses. PLOS Pathog. 9 , e1003176 (2013).
Hause, B. M. et al. Characterization of a novel influenza virus in cattle and Swine: proposal for a new genus in the Orthomyxoviridae family. MBio 5 , e00031–00014 (2014).
Smith, G. J. et al. Origins and evolutionary genomics of the 2009 swine-origin H1N1 influenza A epidemic. Nature 459 , 1122–1125 (2009).
Mena, I. et al. Origins of the 2009 H1N1 influenza pandemic in swine in Mexico. elife 5 , e.16777 (2016). This reference tracks the origins of a human pandemic influenza A virus for the first time .
Hayden, F. Developing new antiviral agents for influenza treatment: what does the future hold? Clin. Infect. Dis. 48 (Suppl. 1), S3–S13 (2009).
Hurt, A. C. et al. Antiviral resistance during the 2009 influenza A H1N1 pandemic: public health, laboratory, and clinical perspectives. Lancet Infect. Dis. 12 , 240–248 (2012).
Rambaut, A. et al. The genomic and epidemiological dynamics of human influenza A virus. Nature 453 , 615–619 (2008).
Russell, C. A. et al. The global circulation of seasonal influenza A (H3N2) viruses. Science 320 , 340–346 (2008). Together with reference 17, this seminal study proposes intriguing models of how influenza viruses are maintained in the human population and transmitted globally during seasonal epidemics .
Bahl, J. et al. Temporally structured metapopulation dynamics and persistence of influenza A H3N2 virus in humans. Proc. Natl Acad. Sci. USA 108 , 19359–19364 (2011).
Yu, H. et al. Characterization of regional influenza seasonality patterns in China and implications for vaccination strategies: spatio-temporal modeling of surveillance data. PLOS Med. 10 , e1001552 (2013).
Centers for Disease Control and Prevention. Estimated influenza illnesses and hospitalizations averted by vaccination — United States, 2014–15 influenza season. CDC https://www.cdc.gov/flu/about/disease/2014-15.htm (2015).
Cohen, S. A., Chui, K. K. & Naumova, E. N. Influenza vaccination in young children reduces influenza-associated hospitalizations in older adults, 2002–2006. J. Am. Geriatr. Soc. 59 , 327–332 (2011).
Gostic, K. M., Ambrose, M., Worobey, M. & Lloyd-Smith, J. O. Potent protection against H5N1 and H7N9 influenza via childhood hemagglutinin imprinting. Science 354 , 722–726 (2016).
Lozano, R. et al. Global and regional mortality from 235 causes of death for 20 age groups in 1990 and 2010: a systematic analysis for the Global Burden of Disease Study 2010. Lancet 380 , 2095–2128 (2012).
Iuliano, A. D. et al. Estimates of global seasonal influenza-associated respiratory mortality: a modelling study. Lancet 391 , 1285–1300 (2018).
Thompson, W. W. et al. Mortality associated with influenza and respiratory syncytial virus in the United States. JAMA 289 , 179–186 (2003). This study represents a classic analysis of hospital-acquired pneumonia and influenza deaths that establishes the impact of seasonal influenza in the United States .
Flannery, B. et al. Influenza vaccine effectiveness against pediatric deaths: 2010–2014. Pediatrics 139 , https://doi.org/10.1542/peds.2016-4244 (2017).
Thompson, W. W. et al. Influenza-associated hospitalizations in the United States. JAMA 292 , 1333–1340 (2004).
Walsh, E. E., Cox, C. & Falsey, A. R. Clinical features of influenza A virus infection in older hospitalized persons. J. Am. Geriatr. Soc. 50 , 1498–1503 (2002).
Jain, S. et al. Hospitalized patients with 2009 H1N1 influenza in the United States, April-June 2009. N. Engl. J. Med. 361 , 1935–1944 (2009).
Keren, R. et al. Neurological and neuromuscular disease as a risk factor for respiratory failure in children hospitalized with influenza infection. JAMA 294 , 2188–2194 (2005).
Neuzil, K. M., Reed, G. W., Mitchel, E. F., Simonsen, L. & Griffin, M. R. Impact of influenza on acute cardiopulmonary hospitalizations in pregnant women. Am. J. Epidemiol. 148 , 1094–1102 (1998).
Van Kerkhove, M. D. et al. Risk factors for severe outcomes following 2009 influenza A (H1N1) infection: a global pooled analysis. PLOS Med. 8 , e1001053 (2011).
Karlsson, E. A. et al. A perfect storm: Increased colonization and failure of vaccination leads to severe secondary bacterial infection in influenza virus-infected obese mice. MBio 8 , e00889-17 (2017).
Ciancanelli, M. J. et al. Life-threatening influenza and impaired interferon amplification in human IRF7 deficiency. Science 348 , 448–453 (2015).
Everitt, A. R. et al. IFITM3 restricts the morbidity and mortality associated with influenza. Nature 484 , 519–523 (2012).
Allen, E. K. et al. SNP-mediated disruption of CTCF binding at the IFITM3 promoter is associated with risk of severe influenza in humans. Nat. Med. 23 , 975–983 (2017).
van Gils, J. A. et al. Hampered foraging and migratory performance in swans infected with low-pathogenic avian influenza A virus. PLOS ONE 2 , e184 (2007).
Guan, Y. & Smith, G. J. The emergence and diversification of panzootic H5N1 influenza viruses. Virus Res. 178 , 35–43 (2013).
Ip, H. S. et al. Novel Eurasian highly pathogenic avian influenza A H5 viruses in wild birds, WA, USA, 2014. Emerg. Infect. Dis. 21 , 886–890 (2015).
Ke, C. et al. Human infection with highly pathogenic avian influenza A(H7N9) virus. China. Emerg. Infect. Dis. 23 , 1332–1340 (2017).
Lee, C. T. et al. Outbreak of influenza A(H7N2) among cats in an animal shelter with cat-to-human transmission-New York City, 2016. Clin. Infect. Dis. 65 , 1927–1929 (2017).
Fouchier, R. A. et al. Avian influenza A virus (H7N7) associated with human conjunctivitis and a fatal case of acute respiratory distress syndrome. Proc. Natl Acad. Sci. USA 101 , 1356–1361 (2004).
Perkins, L. E. & Swayne, D. E. Pathogenicity of a Hong Kong-origin H5N1 highly pathogenic avian influenza virus for emus, geese, ducks, and pigeons. Avian Dis. 46 , 53–63 (2002).
Hulse-Post, D. J. et al. Role of domestic ducks in the propagation and biological evolution of highly pathogenic H5N1 influenza viruses in Asia. Proc. Natl Acad. Sci. USA 102 , 10682–10687 (2005).
Fournie, G. et al. Interventions for avian influenza A (H5N1) risk management in live bird market networks. Proc. Natl Acad. Sci. USA 110 , 9177–9182 (2013).
Vincent, A. et al. Review of influenza A virus in swine worldwide: a call for increased surveillance and research. Zoonoses Publ. Health 61 , 4–17 (2014).
CAS Google Scholar
Neumann, G. & Kawaoka, Y. The first influenza pandemic of the new millennium. Influenza Other Respir. Viruses 5 , 157–166 (2011).
Jhung, M. A. et al. Outbreak of variant influenza A(H3N2) virus in the United States. Clin. Infect. Dis. 57 , 1703–1712 (2013).
Crawford, P. C. et al. Transmission of equine influenza virus to dogs. Science 310 , 482–485 (2005).
Li, S. et al. Avian-origin H3N2 canine influenza A viruses in Southern China. Infect. Genet. Evol. 10 , 1286–1288 (2010).
Yamada, S. et al. Haemagglutinin mutations responsible for the binding of H5N1 influenza A viruses to human-type receptors. Nature 444 , 378–382 (2006).
van Riel, D. et al. H5N1 virus attachment to lower respiratory tract. Science 312 , 399 (2006).
Hirst, G. K. Studies of antigenic differences among strains of influenza A by means of red cell agglutination. J. Exp. Med. 78 , 407–423 (1943).
Barr, I. G. et al. WHO recommendations for the viruses used in the 2013–2014 Northern Hemisphere influenza vaccine: epidemiology, antigenic and genetic characteristics of influenza A(H1N1)pdm09, A(H3N2) and B influenza viruses collected from October 2012 to January 2013. Vaccine 32 , 4713–4725 (2014).
Sandbulte, M. R. et al. Discordant antigenic drift of neuraminidase and hemagglutinin in H1N1 and H3N2 influenza viruses. Proc. Natl Acad. Sci. USA 108 , 20748–20753 (2011).
Kilbourne, E. D., Laver, W. G., Schulman, J. L. & Webster, R. G. Antiviral activity of antiserum specific for an influenza virus neuraminidase. J. Virol. 2 , 281–288 (1968).
Couzens, L. et al. An optimized enzyme-linked lectin assay to measure influenza A virus neuraminidase inhibition antibody titers in human sera. J. Virol. Methods 210 , 7–14 (2014).
Voeten, J. T. et al. Antigenic drift in the influenza A virus (H3N2) nucleoprotein and escape from recognition by cytotoxic T lymphocytes. J. Virol. 74 , 6800–6807 (2000).
Smith, D. J. et al. Mapping the antigenic and genetic evolution of influenza virus. Science 305 , 371–376 (2004). This study describes the concept of antigenic cartography and uses antigenic cartography for the visualization and quantification of antigenic drift of human H3N2 influenza A viruses over 35 years .
Koel, B. F. et al. Substitutions near the receptor binding site determine major antigenic change during influenza virus evolution. Science 342 , 976–979 (2013).
Koel, B. F. et al. Antigenic variation of clade 2.1 H5N1 virus is determined by a few amino acid substitutions immediately adjacent to the receptor binding site. MBio 5 , e01070-01014 (2014).
Lewis, N. S. et al. Antigenic and genetic evolution of equine influenza A (H3N8) virus from 1968 to 2007. J. Virol. 85 , 12742–12749 (2011).
de Jong, J. C. et al. Antigenic and genetic evolution of swine influenza A (H3N2) viruses in Europe. J. Virol. 81 , 4315–4322 (2007).
Kendal, A. P., Noble, G. R., Skehel, J. J. & Dowdle, W. R. Antigenic similarity of influenza A (H1N1) viruses from epidemics in 1977—1978 to “Scandinavian” strains isolated in epidemics of 1950–1951. Virology 89 , 632–636 (1978).
Herfst, S. et al. Airborne transmission of influenza A/H5N1 virus between ferrets. Science 336 , 1534–1541 (2012).
Imai, M. et al. Experimental adaptation of an influenza H5 HA confers respiratory droplet transmission to a reassortant H5 HA/H1N1 virus in ferrets. Nature 486 , 420–428 (2012).
Russell, C. A. et al. The potential for respiratory droplet-transmissible A/H5N1 influenza virus to evolve in a mammalian host. Science 336 , 1541–1547 (2012).
Yoon, S. W., Webby, R. J. & Webster, R. G. Evolution and ecology of influenza A viruses. Curr. Top. Microbiol. Immunol. 385 , 359–375 (2014).
Webster, R. G. & Hulse, D. J. Microbial adaptation and change: avian influenza. Rev. Sci. Tech. 23 , 453–465 (2004).
Andino, R. & Domingo, E. Viral quasispecies. Virology 479–480 , 46–51 (2015).
Tumpey, T. M. et al. A two-amino acid change in the hemagglutinin of the 1918 influenza virus abolishes transmission. Science 315 , 655–659 (2007). This study provides the first evidence that the receptor specificity of the HA of influenza A virus plays a major role in the respiratory transmission of these viruses .
Mitnaul, L. J. et al. Balanced hemagglutinin and neuraminidase activities are critical for efficient replication of influenza A virus. J. Virol. 74 , 6015–6020 (2000).
Reed, M. L. et al. The pH of activation of the hemagglutinin protein regulates H5N1 influenza virus pathogenicity and transmissibility in ducks. J. Virol. 84 , 1527–1535 (2010).
Hatta, M., Gao, P., Halfmann, P. & Kawaoka, Y. Molecular basis for high virulence of Hong Kong H5N1 influenza A viruses. Science 293 , 1840–1842 (2001).
Mehle, A. & Doudna, J. A. Adaptive strategies of the influenza virus polymerase for replication in humans. Proc. Natl Acad. Sci. USA 106 , 21312–21316 (2009).
Riegger, D. et al. The nucleoprotein of newly emerged H7N9 influenza A virus harbors a unique motif conferring resistance to antiviral human MxA. J. Virol. 89 , 2241–2252 (2015).
Campbell, P. J. et al. The M segment of the 2009 pandemic influenza virus confers increased neuraminidase activity, filamentous morphology, and efficient contact transmissibility to A/Puerto Rico/8/1934-based reassortant viruses. J. Virol. 88 , 3802–3814 (2014).
Medina, R. A. & García-Sastre, A. Influenza A viruses: new research developments. Nat. Rev. Microbiol. 9 , 590–603 (2011).
Tong, S. et al. New world bats harbor diverse influenza A viruses. PLOS Pathog. 9 , e1003657 (2013).
Moreira, E. A. et al. Synthetically derived bat influenza A-like viruses reveal a cell type- but not species-specific tropism. Proc. Natl Acad. Sci. USA 113 , 12797–12802 (2016).
Mibayashi, M. et al. Inhibition of retinoic acid-inducible gene I-mediated induction of beta interferon by the NS1 protein of influenza A virus. J. Virol. 81 , 514–524 (2007).
Pichlmair, A. et al. RIG-I-mediated antiviral responses to single-stranded RNA bearing 5′-phosphates. Science 314 , 997–1001 (2006).
Rajsbaum, R. et al. Species-specific inhibition of RIG-I ubiquitination and IFN induction by the influenza A virus NS1 protein. PLOS Pathog. 8 , e1003059 (2012).
Nemeroff, M. E., Barabino, S. M., Li, Y., Keller, W. & Krug, R. M. Influenza virus NS1 protein interacts with the cellular 30 kDa subunit of CPSF and inhibits 3′end formation of cellular pre-mRNAs. Mol. Cell 1 , 991–1000 (1998).
Satterly, N. et al. Influenza virus targets the mRNA export machinery and the nuclear pore complex. Proc. Natl Acad. Sci. USA 104 , 1853–1858 (2007).
Marazzi, I. et al. Suppression of the antiviral response by an influenza histone mimic. Nature 483 , 428–433 (2012).
Chauche, C. et al. Mammalian adaptation of an avian influenza A virus involves stepwise changes in NS1. J. Virol. e01875-17 (2017).
Li, S., Min, J. Y., Krug, R. M. & Sen, G. C. Binding of the influenza A virus NS1 protein to PKR mediates the inhibition of its activation by either PACT or double-stranded RNA. Virology 349 , 13–21 (2006).
Min, J. Y. & Krug, R. M. The primary function of RNA binding by the influenza A virus NS1 protein in infected cells: Inhibiting the 2′-5′ oligo (A) synthetase/RNase L pathway. Proc. Natl Acad. Sci. USA 103 , 7100–7105 (2006).
Conenello, G. M. & Palese, P. Influenza A virus PB1-F2: a small protein with a big punch. Cell Host Microbe 2 , 207–209 (2007).
Graef, K. M. et al. The PB2 subunit of the influenza virus RNA polymerase affects virulence by interacting with the mitochondrial antiviral signaling protein and inhibiting expression of beta interferon. J. Virol. 84 , 8433–8445 (2010).
Jagger, B. W. et al. An overlapping protein-coding region in influenza A virus segment 3 modulates the host response. Science 337 , 199–204 (2012).
Crotta, S. et al. Type I and type III interferons drive redundant amplification loops to induce a transcriptional signature in influenza-infected airway epithelia. PLOS Pathog. 9 , e1003773 (2013).
Helft, J. et al. Cross-presenting CD103+ dendritic cells are protected from influenza virus infection. J. Clin. Invest. 122 , 4037–4047 (2012).
Zhu, L. et al. High level of neutrophil extracellular traps correlates with poor prognosis of severe influenza A infection. J. Infect. Dis. 217 , 428–437 (2018).
Walsh, K. B. et al. Suppression of cytokine storm with a sphingosine analog provides protection against pathogenic influenza virus. Proc. Natl Acad. Sci. USA 108 , 12018–12023 (2011).
Sridhar, S. et al. Cellular immune correlates of protection against symptomatic pandemic influenza. Nat. Med. 19 , 1305–1312 (2013).
Wilkinson, T. M. et al. Preexisting influenza-specific CD4+ T cells correlate with disease protection against influenza challenge in humans. Nat. Med. 18 , 274–280 (2012).
Quinones-Parra, S. et al. Preexisting CD8+ T cell immunity to the H7N9 influenza A virus varies across ethnicities. Proc. Natl Acad. Sci. USA 111 , 1049–1054 (2014).
Wang, Z. et al. Recovery from severe H7N9 disease is associated with diverse response mechanisms dominated by CD8 + T cells. Nat. Commun. 6 , 6833 (2015). This manuscript provides evidence that early cross-reactive CD8 + T cell immunity plays an important part in recovery from severe avian influenza A H7N9 virus-induced disease .
van de Sandt, C. E. et al. Human influenza A virus-specific CD8+ T cell response is long-lived. J. Infect. Dis. 212 , 81–85 (2015).
Sun, J. C. & Bevan, M. J. Defective CD8 T cell memory following acute infection without CD4 T cell help. Science 300 , 339–342 (2003).
McKinstry, K. K. et al. Memory CD4+ T cells protect against influenza through multiple synergizing mechanisms. J. Clin. Invest. 122 , 2847–2856 (2012).
Deliyannis, G. et al. Intranasal lipopeptide primes lung-resident memory CD8+ T cells for long-term pulmonary protection against influenza. Eur. J. Immunol. 36 , 770–778 (2006).
Pejoski, D., Zeng, W., Rockman, S., Brown, L. E. & Jackson, D. C. A lipopeptide based on the M2 and HA proteins of influenza A viruses induces protective antibody. Immunol. Cell. Biol. 88 , 605–611 (2010).
McMichael, A. J., Gotch, F. M., Noble, G. R. & Beare, P. A. Cytotoxic T cell immunity to influenza. N. Engl. J. Med. 309 , 13–17 (1983).
Topham, D. J., Tripp, R. A. & Doherty, P. C. CD8+ T cells clear influenza virus by perforin or Fas-dependent processes. J. Immunol. 159 , 5197–5200 (1997).
Doherty, P. C., Turner, S. J., Webby, R. G. & Thomas, P. G. Influenza and the challenge for immunology. Nat. Immunol. 7 , 449–455 (2006).
Sridhar, S., Brokstad, K. A. & Cox, R. J. Influenza vaccination strategies: comparing inactivated and live attenuated influenza vaccines. Vaccines 3 , 373–389 (2015).
Russ, B. E. et al. Distinct epigenetic signatures delineate transcriptional programs during virus-specific CD8 + T cell differentiation. Immunity 41 , 853–865 (2014).
Valkenburg, S. A. et al. Early priming minimizes the age-related immune compromise of CD8 + T cell diversity and function. PLOS Pathog. 8 , e1002544 (2012).
Smith, W., Andrewes, C. H. & Laidlaw, P. P. A virus obtained from influenza patients. Lancet 222 , 66–68 (1933). This study describes the first isolation of influenza virus. Importantly, it includes proof of protection from influenza virus infection by passive transfer of antibodies in the ferret model of influenza .
Wrammert, J. et al. Rapid cloning of high-affinity human monoclonal antibodies against influenza virus. Nature 453 , 667–671 (2008).
Gerhard, W., Yewdell, J., Frankel, M. E. & Webster, R. Antigenic structure of influenza virus haemagglutinin defined by hybridoma antibodies. Nature 290 , 713–717 (1981).
Angeletti, D. et al. Defining B cell immunodominance to viruses. Nat. Immunol. 18 , 456–463 (2017).
Andrews, S. F. et al. Immune history profoundly affects broadly protective B cell responses to influenza. Sci. Transl Med. 7 , 316ra192 (2015).
Hobson, D., Curry, R. L., Beare, A. S. & Ward-Gardner, A. The role of serum haemagglutination-inhibiting antibody in protection against challenge infection with influenza A2 and B viruses. J. Hyg. 70 , 767–777 (1972).
Yu, X. et al. Neutralizing antibodies derived from the B cells of 1918 influenza pandemic survivors. Nature 455 , 532–536 (2008).
Novel Swine-Origin Influenza A Virus Investigation Team et al. Emergence of a novel swine-origin influenza A (H1N1) virus in humans. N. Engl. J. Med. 360 , 2605–2615 (2009).
Manicassamy, B. et al. Protection of mice against lethal challenge with 2009 H1N1 influenza A virus by 1918-like and classical swine H1N1 based vaccines. PLOS Pathog. 6 , e1000745 (2010).
Steens, A. et al. Age-dependent patterns of infection and severity explaining the low impact of 2009 influenza A (H1N1): evidence from serial serologic surveys in the Netherlands. Am. J. Epidemiol. 174 , 1307–1315 (2011).
Heaton, N. S., Sachs, D., Chen, C. J., Hai, R. & Palese, P. Genome-wide mutagenesis of influenza virus reveals unique plasticity of the hemagglutinin and NS1 proteins. Proc. Natl Acad. Sci. USA 110 , 20248–20253 (2013).
Doud, M. B. & Bloom, J. D. Accurate measurement of the effects of all amino-acid mutations on influenza hemagglutinin. Viruses 8 , 155 (2016).
PubMed Central Google Scholar
Monto, A. S., Malosh, R. E., Petrie, J. G. & Martin, E. T. The doctrine of original antigenic sin: separating good from evil. J. Infect. Dis. 215 , 1782–1788 (2017).
Henry, C., Palm, A. E., Krammer, F. & Wilson, P. C. From original antigenic sin to the universal influenza virus vaccine. Trends Immunol. 39 , 70–79 (2017).
Linderman, S. L. et al. Potential antigenic explanation for atypical H1N1 infections among middle-aged adults during the 2013–2014 influenza season. Proc. Natl Acad. Sci. USA 111 , 15798–15803 (2014).
Li, Y. et al. Immune history shapes specificity of pandemic H1N1 influenza antibody responses. J. Exp. Med. 210 , 1493–1500 (2013).
Krammer, F. & Palese, P. Influenza virus hemagglutinin stalk-based antibodies and vaccines. Curr. Opin. Virol. 3 , 521–530 (2013).
Ekiert, D. C. & Wilson, I. A. Broadly neutralizing antibodies against influenza virus and prospects for universal therapies. Curr. Opin. Virol. 2 , 134–141 (2012).
Neirynck, S. et al. A universal influenza A vaccine based on the extracellular domain of the M2 protein. Nat. Med. 5 , 1157–1163 (1999).
Wohlbold, T. J. et al. Broadly protective murine monoclonal antibodies against influenza B virus target highly conserved neuraminidase epitopes. Nat. Microbiol. 2 , 1415–1424 (2017).
Wan, H. et al. Molecular basis for broad neuraminidase immunity: conserved epitopes in seasonal and pandemic H1N1 as well as H5N1 influenza viruses. J. Virol. 87 , 9290–9300 (2013).
Rajendran, M. et al. Analysis of anti-influenza virus neuraminidase antibodies in children, adults, and the elderly by ELISA and enzyme inhibition: evidence for original antigenic sin. MBio 8 , https://doi.org/10.1128/mBio.02281-16 (2017).
Wohlbold, T. J. & Krammer, F. In the shadow of hemagglutinin: a growing interest in influenza viral neuraminidase and its role as a vaccine antigen. Viruses 6 , 2465–2494 (2014).
DiLillo, D. J., Palese, P., Wilson, P. C. & Ravetch, J. V. Broadly neutralizing anti-influenza antibodies require Fc receptor engagement for in vivo protection. J. Clin. Invest. 126 , 605–610 (2016). This study demonstrates that immune responses induced against the conserved influenza virus HA stalk domain include broadly protective antibodies that activate effector cells via Fc–FcR interactions.
Jegaskanda, S. et al. Cross-reactive influenza-specific antibody-dependent cellular cytotoxicity antibodies in the absence of neutralizing antibodies. J. Immunol. 190 , 1837–1848 (2013).
Jegaskanda, S., Weinfurter, J. T., Friedrich, T. C. & Kent, S. J. Antibody-dependent cellular cytotoxicity is associated with control of pandemic H1N1 influenza virus infection of macaques. J. Virol. 87 , 5512–5522 (2013).
Seibert, C. W. et al. Recombinant IgA is sufficient to prevent influenza virus transmission in guinea pigs. J. Virol. 87 , 7793–7804 (2013).
Lowen, A. C. et al. Blocking interhost transmission of influenza virus by vaccination in the guinea pig model. J. Virol. 83 , 2803–2818 (2009).
Ohmit, S. E. & Monto, A. S. Symptomatic predictors of influenza virus positivity in children during the influenza season. Clin. Infect. Dis. 43 , 564–568 (2006).
Kumar, S. & Henrickson, K. J. Update on influenza diagnostics: lessons from the novel H1N1 influenza A pandemic. Clin. Microbiol. Rev. 25 , 344–361 (2012).
Petric, M., Comanor, L. & Petti, C. A. Role of the laboratory in diagnosis of influenza during seasonal epidemics and potential pandemics. J. Infect. Dis. 194 (Suppl. 2), S98–S110, https://doi.org/10.1086/507554 (2006).
Newton, D. W., Treanor, J. J. & Menegus, M. A. Clinical and laboratory diagnosis of influenza virus infections. Am. J. Manag. Care 6 , S265–S275 (2000).
Dunn, J. J., Woolstenhulme, R. D., Langer, J. & Carroll, K. C. Sensitivity of respiratory virus culture when screening with R-mix fresh cells. J. Clin. Microbiol. 42 , 79–82 (2004).
Nie, S. et al. Evaluation of Alere i Influenza A&B for rapid detection of influenza viruses A and B. J. Clin. Microbiol. 52 , 3339–3344 (2014).
Merckx, J. et al. Diagnostic accuracy of novel and traditional rapid tests for influenza infection compared with reverse transcriptase polymerase chain reaction: A systematic review and meta-analysis. Ann. Intern. Med. 167 , 394–409 (2017).
Bhattacharya, S. et al. Transcriptomic biomarkers to discriminate bacterial from nonbacterial infection in adults hospitalized with respiratory illness. Sci. Rep. 7 , 6548 (2017).
Salk, J. E. & Suriano, P. C. Importance of antigenic composition of influenza virus vaccine in protecting against the natural disease; observations during the winter of 1947–1948. Am. J. Publ. Health Nat. Health 39 , 345–355 (1949).
Plotkin, S. A., Orenstein, W. & Offit, P. (eds), Vaccines 6 th edn (Saunders, 2012)
Rudenko, L., Yeolekar, L., Kiseleva, I. & Isakova-Sivak, I. Development and approval of live attenuated influenza vaccines based on Russian master donor viruses: Process challenges and success stories. Vaccine 34 , 5436–5441 (2016).
Caspard, H., Mallory, R. M., Yu, J. & Ambrose, C. S. Live-attenuated influenza vaccine effectiveness in children from 2009 to 2015-2016: A systematic review and meta-analysis. Open Forum Infect. Dis. 4 , ofx111 (2017).
Manini, I. et al. Egg-independent influenza vaccines and vaccine candidates. Vaccines 5 , 18 (2017).
Centers for Disease Control and Prevention. Flublok seasonal influenza (flu) vaccine. CDC https://www.cdc.gov/flu/protect/vaccine/qa_flublok-vaccine.htm (2017).
Clark, T. W. et al. Trial of 2009 influenza A (H1N1) monovalent MF59-adjuvanted vaccine. N. Engl. J. Med. 361 , 2424–2435 (2009).
Nohynek, H. et al. AS03 adjuvanted AH1N1 vaccine associated with an abrupt increase in the incidence of childhood narcolepsy in Finland. PLOS ONE 7 , e33536 (2012).
DiazGranados, C. A. et al. Efficacy of high-dose versus standard-dose influenza vaccine in older adults. N. Engl. J. Med. 371 , 635–645 (2014).
Enami, M., Luytjes, W., Krystal, M. & Palese, P. Introduction of site-specific mutations into the genome of influenza virus. Proc. Natl Acad. Sci. USA 87 , 3802–3805 (1990).
Krammer, F. & Palese, P. Advances in the development of influenza virus vaccines. Nat. Rev. Drug Discov. 14 , 167–182 (2015).
Paules, C. I., Marston, H. D., Eisinger, R. W., Baltimore, D. & Fauci, A. S. The pathway to a universal influenza vaccine. Immunity 47 , 599–603 (2017).
Committee On Infectious Diseases. Recommendations for prevention and control of influenza in children, 2017–2018. Pediatrics 140 , e20172550 (2017).
Palese, P. & Wang, T. T. H5N1 influenza viruses: facts, not fear. Proc. Natl Acad. Sci. USA 109 , 2211–2213 (2012).
Gomaa, M. R. et al. Avian influenza A(H5N1) and A(H9N2) seroprevalence and risk factors for infection among Egyptians: a prospective, controlled seroepidemiological study. J. Infect. Dis. 211 , 1399–1407 (2015).
Wang, T. T., Parides, M. K. & Palese, P. Seroevidence for H5N1 influenza infections in humans: meta-analysis. Science 335 , 1463 (2012).
Varble, A. et al. Influenza A virus transmission bottlenecks are defined by infection route and recipient host. Cell Host Microbe 16 , 691–700 (2014).
Sobel Leonard, A., Weissman, D. B., Greenbaum, B., Ghedin, E. & Koelle, K. Transmission bottleneck size estimation from pathogen deep-sequencing data, with an application to human influenza A virus. J. Virol. 91 , e00171-17 (2017).
Osterholm, M. T., Kelley, N. S., Sommer, A. & Belongia, E. A. Efficacy and effectiveness of influenza vaccines: a systematic review and meta-analysis. Lancet Infect. Dis. 12 , 36–44 (2012).
DiazGranados, C. A., Denis, M. & Plotkin, S. Seasonal influenza vaccine efficacy and its determinants in children and non-elderly adults: a systematic review with meta-analyses of controlled trials. Vaccine 31 , 49–57 (2012).
Beyer, W. E. et al. Reply: Letter to the editor, Cochrane rearranged. Vaccine 33 , 13–14 (2015).
Centers for Disease Control and Prevention. ACIP votes down use of LAIV for 2016–2017 flu season. CDC https://www.cdc.gov/media/releases/2016/s0622-laiv-flu.html (2016).
Saito, N. et al. Negative impact of prior influenza vaccination on current influenza vaccination among people infected and not infected in prior season: a test-negative case-control study in Japan. Vaccine 35 , 687–693 (2017).
Paules, C. I., Sullivan, S. G., Subbarao, K. & Fauci, A. S. Chasing seasonal influenza - the need for a universal influenza vaccine. N. Engl. J. Med. 378 , 7–9 (2018).
Hata, A., Akashi-Ueda, R., Takamatsu, K. & Matsumura, T. Safety and efficacy of peramivir for influenza treatment. Drug. Des. Dev. Ther. 8 , 2017–2038 (2014).
McKimm-Breschkin, J. L. Influenza neuraminidase inhibitors: antiviral action and mechanisms of resistance. Influenza Other Respir. Viruses 7 (Suppl. 1), 25–36 (2013).
Okoli, G. N., Otete, H. E., Beck, C. R. & Nguyen-Van-Tam, J. S. Use of neuraminidase inhibitors for rapid containment of influenza: a systematic review and meta-analysis of individual and household transmission studies. PLOS ONE 9 , e113633 (2014).
Muthuri, S. G. et al. Effectiveness of neuraminidase inhibitors in reducing mortality in patients admitted to hospital with influenza A H1N1pdm09 virus infection: a meta-analysis of individual participant data. Lancet Respir. Med. 2 , 395–404 (2014).
Dobson, J., Whitley, R. J., Pocock, S. & Monto, A. S. Oseltamivir treatment for influenza in adults: a meta-analysis of randomised controlled trials. Lancet 385 , 1729–1737 (2015).
Venkatesan, S. et al. Impact of outpatient neuraminidase inhibitor treatment in patients infected with influenza A(H1N1)pdm09 at high risk of hospitalization: an individual participant data metaanalysis. Clin. Infect. Dis. 64 , 1328–1334 (2017).
Deyde, V. M. et al. Surveillance of resistance to adamantanes among influenza A(H3N2) and A(H1N1) viruses isolated worldwide. J. Infect. Dis. 196 , 249–257 (2007).
Gubareva, L. V. et al. Comprehensive assessment of 2009 pandemic influenza A (H1N1) virus drug susceptibility in vitro. Antivir. Ther 15 , 1151–1159 (2010).
Meijer, A. et al. Oseltamivir-resistant influenza virus A (H1N1), Europe, 2007–2008 season. Emerg. Infect. Dis. 15 , 552–560 (2009).
Bloom, J. D., Gong, L. I. & Baltimore, D. Permissive secondary mutations enable the evolution of influenza oseltamivir resistance. Science 328, 1272–1275.
Abed, Y., Pizzorno, A., Bouhy, X. & Boivin, G. Role of permissive neuraminidase mutations in influenza A/Brisbane/59/2007-like (H1N1) viruses. PLOS Pathog. 7 , e1002431 (2011).
Okomo-Adhiambo, M. et al. Oseltamivir-resistant influenza A(H1N1)pdm09 viruses, United States, 2013–2014. Emerg. Infect. Dis. 21 , 136–141 (2015).
Operario, D. J., Moser, M. J. & St George, K. Highly sensitive and quantitative detection of the H274Y oseltamivir resistance mutation in seasonal A/H1N1 influenza virus. J. Clin. Microbiol. 48 , 3517–3524 (2010).
Memoli, M. J., Hrabal, R. J., Hassantoufighi, A., Eichelberger, M. C. & Taubenberger, J. K. Rapid selection of oseltamivir- and peramivir-resistant pandemic H1N1 virus during therapy in 2 immunocompromised hosts. Clin. Infect. Dis. 50 , 1252–1255 (2010).
Whitley, R. J. et al. Global assessment of resistance to neuraminidase inhibitors, 2008-2011: the Influenza Resistance Information Study (IRIS). Clin. Infect. Dis. 56 , 1197–1205 (2013).
Butler, J. et al. Estimating the fitness advantage conferred by permissive neuraminidase mutations in recent oseltamivir-resistant A(H1N1)pdm09 influenza viruses. PLOS Pathog. 10 , e1004065 (2014).
Meijer, A. et al. Global update on the susceptibility of human influenza viruses to neuraminidase inhibitors, 2012–2013. Antiviral Res. 110 , 31–41 (2014).
Yamashita, M. Laninamivir and its prodrug, CS-8958: long-acting neuraminidase inhibitors for the treatment of influenza. Antivir. Chem. Chemother. 21 , 71–84 (2010).
Furuta, Y. et al. Favipiravir (T-705), a novel viral RNA polymerase inhibitor. Antiviral Res. 100 , 446–454 (2013).
Clark, M. P. et al. Discovery of a novel, first-in-class, orally bioavailable azaindole inhibitor (VX-787) of influenza PB2. J. Med. Chem. 57 , 6668–6678 (2014).
Portsmouth, S., Kawaguchi, K., Arai, M., Tsuchiya, K. & Uehara, T. Cap-dependent endonuclease inhibitor S-033188 for the treatment of influenza: results from a phase 3, randomized, double-blind, placebo- and active-controlled study in otherwise healthy adolescents and adults with seasonal influenza. Open Forum Infect. Dis. 4 , S744 (2017). This paper reports clinical trial results of baloxavir marboxil, a new influenza drug approved in Japan in 2018 .
Haffizulla, J. et al. Effect of nitazoxanide in adults and adolescents with acute uncomplicated influenza: a double-blind, randomised, placebo-controlled, phase 2b/3 trial. Lancet Infect. Dis. 14 , 609–618 (2014).
Beigel, J. H. et al. Immune plasma for the treatment of severe influenza: an open-label, multicentre, phase 2 randomised study. Lancet Respir. Med. 5 , 500–511 (2017).
Hung, I. F. N. et al. Hyperimmune IV immunoglobulin treatment: a multicenter double-blind randomized controlled trial for patients with severe 2009 influenza A(H1N1) infection. Chest 144 , 464–473 (2013).
Koszalka, P., Tilmanis, D. & Hurt, A. C. Influenza antivirals currently in late-phase clinical trial. Influenza Other Respir. Viruses 11 , 240–246 (2017).
Torner, N. et al. Effectiveness of non-pharmaceutical measures in preventing pediatric influenza: a case-control study. BMC Publ. Health 15 , 543 (2015).
Halloran, M. E. et al. Modeling targeted layered containment of an influenza pandemic in the United States. Proc. Natl Acad. Sci. USA 105 , 4639–4644 (2008).
Fiore, A. E. et al. Antiviral agents for the treatment and chemoprophylaxis of influenza — recommendations of the Advisory Committee on Immunization Practices (ACIP). MMWR Recomm. Rep. 60 , 1–24 (2011).
Uyeki, T. Antiviral treatment for patients hospitalized with 2009 pandemic influenza A (H1N1). N. Engl. J. Med. 361 , e110 (2009).
Stewart, R. J. et al. Influenza antiviral prescribing for outpatients with an acute respiratory illness and at high risk for influenza-associated complications during 5 influenza seasons-United States, 2011–2016. Clin. Infect. Dis. 66 , 1035–1041 (2018).
Oboho, I. K. et al. Oseltamivir use among children and adults hospitalized with community-acquired pneumonia. Open Forum Infect. Dis. 4 , ofw254 (2017).
Coleman, B. L. et al. Pre-and post-pandemic trends in antiviral use in hospitalized patients with laboratory-confirmed influenza: 2004/05-2013/14, Toronto, Canada. Antiviral Res. 140 , 158–163 (2017).
Hung, I. F. N. et al. Efficacy of clarithromycin-naproxen-oseltamivir combination in the treatment of patients hospitalized for influenza A(H3N2) infection: an open-label randomized, controlled, phase IIb/III trial. Chest 151 , 1069–1080 (2017).
Sims, L. D. Intervention strategies to reduce the risk of zoonotic infection with avian influenza viruses: scientific basis, challenges and knowledge gaps. Influenza Other Respir. Viruses 7 (Suppl. 2), 15–25 (2013).
Domenech, J. et al. Experiences with vaccination in countries endemically infected with highly pathogenic avian influenza: the Food and Agriculture Organization perspective. Rev. Sci. Tech. 28 , 293–305 (2009).
Ellis, T. M. et al. Use of avian influenza vaccination in Hong Kong. Dev. Biol. 124 , 133–143 (2006).
Leung, Y. H. et al. Avian influenza and ban on overnight poultry storage in live poultry markets, Hong Kong. Emerg. Infect. Dis. 18 , 1339–1341 (2012).
Lau, E. H. et al. Effect of interventions on influenza A (H9N2) isolation in Hong Kong’s live poultry markets, 1999–2005. Emerg. Infect. Dis. 13 , 1340–1347 (2007).
Bao, C. J. et al. Live-animal markets and influenza A (H7N9) virus infection. N. Engl. J. Med. 368 , 2337–2339 (2013).
Chambers, T. M., Dubovi, E. J. & Donis, R. O. in Textbook of Influenza 2nd edn (eds Webster, R. G., Monto, A. S., Braciale, T. J. & Lamb, R. A.) (Blackwell Science, Oxford, 2013).
Nichol, K. L., D’Heilly, S. J., Greenberg, M. E. & Ehlinger, E. Burden of influenza-like illness and effectiveness of influenza vaccination among working adults aged 50–64 years. Clin. Infect. Dis. 48 , 292–298 (2009).
Gozalo, P. L., Pop-Vicas, A., Feng, Z., Gravenstein, S. & Mor, V. Effect of influenza on functional decline. J. Am. Geriatr. Soc. 60 , 1260–1267 (2012).
Molinari, N. A. et al. The annual impact of seasonal influenza in the US: measuring disease burden and costs. Vaccine 25 , 5086–5096 (2007).
Rello, J. & Pop-Vicas, A. Clinical review: primary influenza viral pneumonia. Crit. Care 13 , 235 (2009).
Morens, D. M., Taubenberger, J. K. & Fauci, A. S. Predominant role of bacterial pneumonia as a cause of death in pandemic influenza: implications for pandemic influenza preparedness. J. Infect. Dis. 198 , 962–970 (2008).
Chien, Y. W., Klugman, K. P. & Morens, D. M. Bacterial pathogens and death during the 1918 influenza pandemic. N. Engl. J. Med. 361 , 2582–2583 (2009).
Morris, D. E., Cleary, D. W. & Clarke, S. C. Secondary bacterial infections associated with influenza pandemics. Front. Microbiol. 8 , 1041 (2017).
Taubenberger, J. K. & Morens, D. M. The pathology of influenza virus infections. Annu. Rev. Pathol. 3 , 499–522 (2008).
Agyeman, P., Duppenthaler, A., Heininger, U. & Aebi, C. Influenza-associated myositis in children. Infection 32 , 199–203 (2004).
MacDonald, K. L. et al. Toxic shock syndrome. A newly recognized complication of influenza and influenzalike illness. JAMA 257 , 1053–1058 (1987).
Steininger, C. et al. Acute encephalopathy associated with influenza A virus infection. Clin. Infect. Dis. 36 , 567–574 (2003).
Dourmashkin, R. R. What caused the 1918–1930 epidemic of encephalitis lethargica? J. R. Soc. Med. 90 , 515–520 (1997).
Zost, S. J. et al. Contemporary H3N2 influenza viruses have a glycosylation site that alters binding of antibodies elicited by egg-adapted vaccine strains. Proc. Natl Acad. Sci. USA 114 , 12578–12583 (2017).
Hayden, F. G. Experimental human influenza: observations from studies of influenza antivirals. Antivir. Ther. 17 , 133–141 (2012).
Erbelding, E. J. et al. A universal influenza vaccine: the strategic plan for the National Institute of Allergy and Infectious Diseases. J. Infect. Dis. https://doi.org/10.1093/infdis/jiy103 (2018).
Freidl, G. S. et al. Influenza at the animal-human interface: a review of the literature for virological evidence of human infection with swine or avian influenza viruses other than A(H5N1). Euro Surveill. 19 https://www.eurosurveillance.org/images/dynamic/EE/V19N18/art20793.pdf (2014).
Abdelwhab el, S. M., Veits, J. & Mettenleiter, T. C. Genetic changes that accompanied shifts of low pathogenic avian influenza viruses toward higher pathogenicity in poultry. Virulence 4 , 441–452 (2013).
World Health Organization. Cumulative number of confirmed human cases for avian influenza A(H5N1) reported to WHO, 2003–2017. WHO http://www.who.int/influenza/human_animal_interface/2017_12_07_tableH5N1.pdf (2017).
Pantin-Jackwood, M. J. et al. Role of poultry in the spread of novel H7N9 influenza virus in China. J. Virol. 88 , 5381–5390 (2014).
World Health Organization. Human infection with avian influenza A(H7N9) virus – China. WHO http://www.who.int/csr/don/26-october-2017-ah7n9-china/en/ (2017).
Imai, M. et al. A highly pathogenic avian H7N9 influenza virus isolated from A human is lethal in some ferrets infected via respiratory droplets. Cell Host Microbe 22 , 615–626 (2017).
Li, C. et al. Evolution of H9N2 influenza viruses from domestic poultry in Mainland China. Virology 340 , 70–83 (2005).
Lam, T. T. et al. The genesis and source of the H7N9 influenza viruses causing human infections in China. Nature 502 , 241–244 (2013).
Palese, P., Tumpey, T. M. & García-Sastre, A. What can we learn from reconstructing the extinct 1918 pandemic influenza virus? Immunity 24 , 121–124 (2006).
Matthey, S. et al. Rapid detection of respiratory viruses by shell vial culture and direct staining by using pooled and individual monoclonal antibodies. J. Clin. Microbiol. 30 , 540–544 (1992).
Loeffelholz, M. J. et al. Comparison of the FilmArray Respiratory Panel and Prodesse real-time PCR assays for detection of respiratory pathogens. J. Clin. Microbiol. 49 , 4083–4088 (2011).
Teo, J. et al. VereFlu: an integrated multiplex RT-PCR and microarray assay for rapid detection and identification of human influenza A and B viruses using lab-on-chip technology. Arch. Virol. 156 , 1371–1378 (2011).
Kim, D. K. & Poudel, B. Tools to detect influenza virus. Yonsei Med. J. 54 , 560–566 (2013).
Hurt, A. C., Alexander, R., Hibbert, J., Deed, N. & Barr, I. G. Performance of six influenza rapid tests in detecting human influenza in clinical specimens. J. Clin. Virol. 39 , 132–135 (2007).
Download references
Acknowledgements
All authors are part of the NIAID-funded Centers of Excellence for Influenza Research and Surveillance (CEIRS) network.
Reviewer information
Nature Reviews Disease Primers thanks R.W. Compans, F. Hayden, H. Kida, H.-D. Klenk, A. Osterhaus and the other anonymous reviewer(s) for their contribution to the peer review of this work.
Author information
Authors and affiliations.
Department of Microbiology, Icahn School of Medicine at Mount Sinai, New York, NY, USA
Florian Krammer, Peter Palese, Megan L. Shaw & Adolfo García-Sastre
Duke–NUS Medical School, Singapore, Singapore
Gavin J. D. Smith
Duke Global Health Institute, Duke University, Durham, NC, USA
Department of Viroscience, Erasmus MC, Rotterdam, Netherlands
Ron A. M. Fouchier
WHO Collaborating Centre for Infectious Disease Epidemiology and Control, School of Public Health, Li Ka Shing Faculty of Medicine, The University of Hong Kong, Hong Kong Special Administrative Region, Hong Kong, China
Malik Peiris
Center of Influenza Research, Li Ka Shing Faculty of Medicine, The University of Hong Kong, Hong Kong Special Administrative Region, Hong Kong, China
Department of Microbiology and Immunology, University of Melbourne at the Peter Doherty Institute for Infection and Immunity, Melbourne, Victoria, Australia
Katherine Kedzierska & Peter C. Doherty
Department of Immunology, St Jude Children’s Research Hospital, Memphis, TN, USA
Peter C. Doherty
Division of Infectious Diseases, Department of Medicine, Icahn School of Medicine at Mount Sinai, New York, NY, USA
Peter Palese & Adolfo García-Sastre
Division of Infectious Diseases, Department of Medicine, University of Rochester School of Medicine and Dentistry, Rochester, NY, USA
John Treanor
Department of Infectious Diseases, St Jude Children’s Research Hospital, Memphis, TN, USA
Robert G. Webster
Global Health and Emerging Pathogens Institute, Icahn School of Medicine at Mount Sinai, New York, NY, USA
Adolfo García-Sastre
You can also search for this author in PubMed Google Scholar
Contributions
Introduction (A.G.-S.); Epidemiology (G.J.D.S.); Mechanisms/pathophysiology (A.G.-S., R.A.M.F., M.P., F.K., K.K. and P.C.D.); Diagnosis, screening and prevention (P.P. and M.L.S.); Management (J.T. and R.G.W.); Quality of life (J.T.); Outlook (A.G.-S.); Overview of the Primer (A.G.-S.).
Corresponding author
Correspondence to Adolfo García-Sastre .
Ethics declarations
Competing interests.
F.K., P.P., A.G.-S. and R.G.W. are inventors of influenza vaccine technologies owned by the Icahn School of Medicine at Mount Sinai, New York, USA. All other authors declare no competing interests.
Additional information
Publisher’s note.
Springer Nature remains neutral with regard to jurisdictional claims in published maps and institutional affiliations.
Rights and permissions
Reprints and permissions
About this article
Cite this article.
Krammer, F., Smith, G.J.D., Fouchier, R.A.M. et al. Influenza. Nat Rev Dis Primers 4 , 3 (2018). https://doi.org/10.1038/s41572-018-0002-y
Download citation
Published : 28 June 2018
DOI : https://doi.org/10.1038/s41572-018-0002-y
Share this article
Anyone you share the following link with will be able to read this content:
Sorry, a shareable link is not currently available for this article.
Provided by the Springer Nature SharedIt content-sharing initiative
This article is cited by
Impaired influenza a virus replication by the host restriction factor samhd1 which inhibited by pa-mediated dephosphorylation of the host transcription factor irf3.
- Zhilei Zhao
- Hongxuan He
Virology Journal (2024)
The prediction of influenza-like illness using national influenza surveillance data and Baidu query data
BMC Public Health (2024)
The role of PB1-F2 in adaptation of high pathogenicity avian influenza virus H7N7 in chickens
- Luise Hohensee
- David Scheibner
- Ulrike Blohm
Veterinary Research (2024)
Vaccine design via antigen reorientation
- Joshua J. Carter
- Peter S. Kim
Nature Chemical Biology (2024)
Highly pathogenic avian influenza virus H5N1 clade 2.3.4.4b from Peru forms a monophyletic group with Chilean isolates in South America
- Gina R. Castro-Sanguinetti
- Rosa González-Veliz
- Juan A. More-Bayona
Scientific Reports (2024)
Quick links
- Explore articles by subject
- Guide to authors
- Editorial policies
Sign up for the Nature Briefing: Microbiology newsletter — what matters in microbiology research, free to your inbox weekly.

Advertisement
Supported by
Global Health
Is Bird Flu Coming to People Next? Are We Ready?
Unlike the coronavirus, the H5N1 virus has been studied for years. Vaccines and treatments are available should they ever become necessary.
- Share full article

By Apoorva Mandavilli
Bird flu outbreaks among dairy cows in multiple states, and at least one infection in farmworker in Texas, have incited fears that the virus may be the next infectious threat to people.
The influenza virus, called H5N1, is highly pathogenic, meaning it has the ability to cause severe disease and death. But while its spread among cows was unexpected, people can catch the virus only from close contact with infected animals, not from one another, federal officials said.
“It’s really about folks who are in environments where they may be interacting with cattle that are infected with this virus,” said Dr. Demetre Daskalakis, the director of the National Center for Immunization and Respiratory Diseases at the Centers for Disease Control and Prevention.
“The risk for most everyone else is very low,” he added. “Right now, our risk assessment hasn’t changed, but if it does change, we’re going be pretty quick and pretty transparent about that.”
Avian influenza is often fatal in birds, but none of the infected cows have died so far. The only symptom in the patient in Texas was conjunctivitis, or pink eye, which was also reported in people infected during other bird flu outbreaks.
The C.D.C. and other agencies in the United States and elsewhere have tracked H5N1 for years to monitor its evolution. Federal agencies have stockpiled vaccines and drugs to be used in a possible bird flu outbreak.
“We are more ready for an influenza pandemic than probably any other outbreak that could occur, any other pathogen,” said Rick Bright, the chief executive of Bright Global Health, a consulting company that focuses on improving responses to public health emergencies.
Dr. Bright led influenza preparedness at the Biomedical Advanced Research and Development Authority, or BARDA, the federal agency that supports research on vaccines and drugs for emergencies, for several years before he served as the agency’s director from 2016 to 2020.
Here’s what you need to know about the H5N1 virus:
Is a human pandemic inevitable?
Among birds and animals, the H5N1 bird flu is already a pandemic, or a panzootic, with infections observed on every continent except Australia. To date, the virus has not evolved into a form that can spread easily from one person to another, and it may never do so.
As its name indicates, H5N1 has primarily been a problem in birds. But it has now spread to a wide range of species, from sea birds and small scavengers like foxes to large mammals, like bears and cows.
There have been sporadic infections in people since 1997, when a cluster of cases appeared in Hong Kong. But most patients worldwide have been in very close contact with infected animals, and generally they did not pass the virus to other people.
To become adept at transmission between people, H5N1 would need to pick up several additional mutations and shift its shape. The strain that was isolated from the infected farmworker in Texas carries one of those mutations, but that change has appeared before — in people, foxes and seals, among others — without further consequences.
The infections in people so far “fortunately are all still single-time cross-species transmission,” said Vincent Munster, a virologist at the National Institute of Allergy and Infectious Diseases who has studied the mutations needed for H5N1 to adapt to people.
History suggests that even if the virus changes enough to begin widespread transmission between people, it may have to give something up in return, Dr. Munster said. For example, when other flu viruses have adapted to humans , they have lost much of their virulence, causing only mild symptoms.
How will we know if the virus spreads to people?
H5N1 is an influenza virus that is followed by extensive scientific networks that track flu viruses worldwide.
“We’ve got our eye on it, and we’ve had our eye on it for years,” Dr. Daskalakis said.
These surveillance networks tracked H5N1 even before its surge in birds and animals over the past two years. Now they are on high alert. Scientists are watching for mutations that could make H5N1 more likely to infect people or resistant to the vaccines and drugs available to counter it.
The World Health Organization, the C.D.C. and other global health organizations routinely share information and genetic sequences to monitor which flu strains are spreading and where.
In the current outbreak, the Department of Agriculture has shared genetic sequences from infected cows with the C.D.C., which analyzes the sequences and ensures that the stockpiled vaccines and drugs are still effective.
Do we have a vaccine for bird flu?
BARDA has enough building blocks for vaccines — including adjuvants, substances that can enhance a vaccine’s strength — to make millions of doses in weeks. Mass production could also ramp up quickly if needed, federal officials said.
The C.D.C. already has two candidate viruses that can be used to make vaccines. As the virus changes — gaining mutations that make it resistant to the current vaccines and drugs, for example — federal researchers may create newer candidates.
Three pharmaceutical companies can be called on to make vaccines for bird flu, but those vaccines would be manufactured on the same production lines that are used to make seasonal flu vaccines. Before embarking on large-scale manufacturing, federal officials would have to consider the implications of disrupting seasonal production, said David Boucher, the infectious disease director at the federal Administration for Strategic Preparedness and Response.
The pharmaceutical companies don’t all use egg-based methods to produce vaccines, an important consideration because of the potential for bird flu outbreaks to derail the nation’s egg supply. BARDA is also looking to add mRNA to the list of technologies that can be used to make bird flu vaccines. (The Covid-19 vaccines made by Pfizer and Moderna relied on the method.)
What about treatments?
At least four antiviral medications are available to treat people who may become sick with bird flu, including the widely available generic drug oseltamivir, sometimes marketed as Tamiflu.
Unlike the vaccines, which are stockpiled by the federal government, the antiviral drugs are available commercially. Generic versions of oseltamivir are made by many manufacturers worldwide.
The federal government has a stockpile of tens of millions of doses of oseltamivir, Dr. Boucher said. The government is in close communication with manufacturers that could quickly ramp up the production of oseltamivir, as it has in the past during some bad flu seasons.
All of these preparations are in place for a worst-case scenario, but “we’re not there yet,” Dr. Boucher said. “Our job here is to prepare for the worst and get ready for it in case it does come.”
Apoorva Mandavilli is a reporter focused on science and global health. She was a part of the team that won the 2021 Pulitzer Prize for Public Service for coverage of the pandemic. More about Apoorva Mandavilli
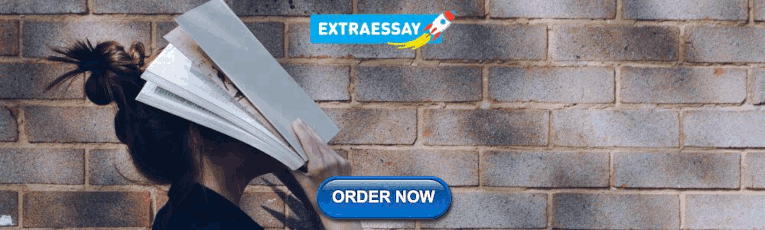
IMAGES
VIDEO
COMMENTS
INFLUENZA VACCINE DEVELOPMENT—A GLOBAL ACHIEVEMENT. The history of influenza vaccination is a success story that started almost a century ago (Figure 1).The first influenza vaccines were a monovalent inactivated influenza A vaccine produced in embryonated chicken eggs and a live-attenuated vaccine in the mid-1930s [30, 31], only a few years after the first isolations of influenza viruses ...
It can even lead to the emergence of a new influenza epidemic and a worldwide pandemic. Influenza B viruses also cause the seasonal flu epidemic in humans. There are two circulating influenza B lineages, B/Yamagata and B/Victoria, which are included in the seasonal flu vaccines [ 1 ]. Meanwhile, influenza C viruses commonly cause mild symptoms ...
Influenza in the Context of the SARS‐CoV‐2 Pandemic. There is emerging evidence that coinfection of influenza with coronavirus disease 2019 (COVID‐19) (the illness caused by SARS‐CoV‐2) is rare; however, individuals with both infections may be at higher risk of poor health outcomes. 10, 11 It is plausible that the careful use of social distancing, face covering, and handwashing used ...
Abstract. Although antiviral drugs and vaccines have reduced the economic and healthcare burdens of influenza, influenza epidemics continue to take a toll. Over the past decade, research on ...
In a recent study, a 20-valent mRNA vaccine elicited immunity against influenza virus in mice. These results, combined with the success of mRNA vaccines against Covid-19, augur well for a new type ...
Based on the cumulative data from 220 clinical trials of influenza vaccines involving 429,804 children, adults, and elderly people, we found that, in adults and elderly, all influenza vaccines, except for the trivalent inactivated intradermal vaccine, conferred protection against laboratory-confirmed influenza compared against placebo or no ...
This article lays out a strategic plan for the development of a universal influenza vaccine and reiterates the commitment from the US government for further investment in influenza vaccine research.
Recent evidence suggests that vaccination against influenza may reduce the clinical outcomes of COVID-19. This study looked at the link between influenza vaccination and the severity of COVID-19 ...
Substances. Antiviral Agents. Influenza Vaccines. Influenza is a highly contagious, deadly virus, killing nearly half a million people yearly worldwide. The classic symptoms of influenza are fever, fatigue, cough, and body aches. In the outpatient setting, diagnosis can be made by clinical presentation with optional confirmatory diagnostic ...
When urgent coronavirus disease 2019 (COVID-19) vaccine development efforts began in earnest in early 2020, researchers were by no means starting from scratch. That's in part attributable to the decades of research dedicated to creating better influenza vaccines. Indeed, many flu vaccinologists pivoted to COVID-19 two years ago, bringing to ...
The supplement articles detail ongoing research and what remains to be learned about influenza—such as how the human immune system responds to influenza infection and vaccination. Experts also discuss how such research might influence vaccine design approaches and help the public health community better prepare for the next influenza pandemic.
We identified 4979 publications, selected 681 for full review, and included 83 in the systematic review and 41 in meta-analyses. ΔVE for vaccination in both seasons compared with the current season was -9% (95% CI -16 to -1, I 2 =0%; low certainty) for influenza A(H1N1)pdm09, -18% (-26 to -11, I 2 =7%; low certainty) for influenza A(H3N2), and -7% (-14 to 0, I 2 =0%; low ...
Annual seasonal influenza epidemics of variable severity caused by influenza A and B virus infections result in substantial disease burden worldwide. Seasonal influenza virus circulation declined markedly in 2020-21 after SARS-CoV-2 emerged but increased in 2021-22. Most people with influenza have abrupt onset of respiratory symptoms and myalgia with or without fever and recover within 1 ...
Objectives To compare the efficacy of influenza vaccines of any valency for adults 60 years and older. Design and setting Systematic review with network meta-analysis (NMA) of randomised controlled trials (RCTs). MEDLINE, EMBASE, JBI Evidence-Based Practice (EBP) Database, PsycINFO, and Cochrane Evidence -Based Medicine database were searched from inception to 20 June 20, 2022.
Abstract. Methods for assessing influenza vaccine efficacy and effectiveness have evolved over six decades. Randomized trials remain the gold standard for licensure, but observational studies are needed for annual assessment of vaccine effectiveness (VE). The test-negative design (TND) has become the de facto standard for these field studies.
Vaccination is the most effective measure at preventing influenza virus infections. However, current seasonal influenza vaccines are only protective against closely matched circulating strains. Even with extensive monitoring and annual reformulation our efforts remain one step behind the rapidly evolving virus, often resulting in mismatches and low vaccine effectiveness. Fortunately, many next ...
A number of studies have suggested that influenza vaccination can provide protection against COVID-19, but the underlying mechanisms that could explain this association are still unclear. In this study, the effect of the 2021/2022 seasonal influenza vaccination on the immune response to the booster dose of anti-SARS-CoV-2 vaccination was evaluated in a cohort of healthy individuals. A total of ...
The trial was established to assess seasonal influenza vaccine efficacy in school-aged children, 6-17 years old, initially from 119 households, including 71 households in which a child received ...
The CDC's latest data show that as of late March, 74 percent of adults age 65 years and older had gotten the flu vaccine and just 42 percent had received the updated COVID vaccine. Although ...
From 2010 to 2012, vaccination led to a 74% reduction in the risk of influenza-related ICU admissions in children , and according to a 2017 study, vaccination reduced the risk of influenza-related hospitalizations in older adults by an average of 40% between 2009 and 2016 . Thus, vaccine prevention of influenza is both effective and justified.
In 2013, the same research team published a paper showing that flu infections also induce us to produce RNAi molecules. "That's why our next step is to use this same concept to generate a flu ...
TUESDAY, April 16, 2024 (HealthDay News) -- Genetics-based "one-and-done" vaccines for the flu and COVID could prove more effective and easier to craft than current jabs, researchers report.
Several research teams are working on nasal COVID-19 vaccines, and both China and India have approved the use of nasal sprays in the form of boosters. Follow me on Twitter or LinkedIn . Send me a ...
In contrast, the influenza vaccinees possessed robust immune memory for the vaccine antigens prior to vaccination, and the recall vaccination moderately boosted antibody production and Bmem responses. Antibody levels and Bmem responses waned several months after the 2nd COVID-19 vaccination, but were restored upon the 3rd vaccination.
Research Article. Evolution of H7N9 highly pathogenic avian influenza virus in the context of vaccination. ... that have sub-optimal immunity may provide a unique opportunity to witness the evolution of highly pathogenic avian influenza virus in the context of vaccination. Between January 2020 and June 2023, we isolated 16 H7N9 viruses from ...
1. Introduction. Influenza disease, usually called "the flu", is a contagious respiratory illness caused by influenza viruses. The common symptoms are fever, aches, chills, chest discomfort, cough, and headache [].The incubation period is very short, typically from 1 to 4 days [].While the majority of infected subjects recover, some develop complications, particularly at-risk groups such ...
The Good Men Project reposted an article from Futurity that reported on a study, co-authored by Jonathan Lovell in which UB researchers are developing a recombinant flu vaccine that would offer several advantages over conventional vaccines. UB Research News.
Scientific Reports (2024) Influenza is an infectious respiratory disease that, in humans, is caused by influenza A and influenza B viruses. Typically characterized by annual seasonal epidemics ...
Dr. Bright led influenza preparedness at the Biomedical Advanced Research and Development Authority, or BARDA, the federal agency that supports research on vaccines and drugs for emergencies, for ...