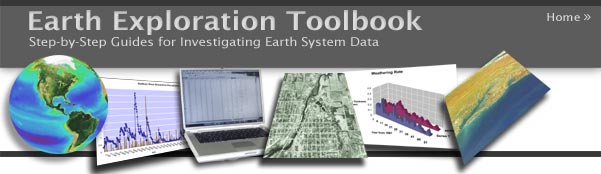
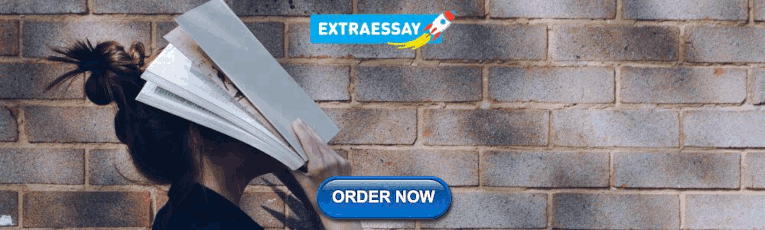
Case Study: Predicting the Next Big Earthquake
Recent earthquake activity.
USGS Recent Worldwide Earthquake Activity To explore individual earthquakes in more depth, click on the UTC Date-Time field. Show me how Hide Details for accessing USGS Recent Worldwide Earthquake Activity Scroll the list to look over earthquakes that have occurred in the last seven days. To explore individual earthquakes in more depth, follow the COMMENTS links. Scroll to the bottom of the list to view recent Earthquakes plotted on a world map. What is the magnitude of the most recent recorded earthquake? How many earthquakes were recorded for the last seven days? Of those earthquakes, how many were of a magnitude 7.0 or greater? IRIS Seismic Monitor Click on the map to zoom to specific regions. Click on individual earthquakes to see lists of others nearby. Show me how Hide Details for accessing the IRIS Seismic Monitor Click on the map to zoom to specific regions. Click on individual earthquakes to see lists of others nearby. Where are earthquakes concentrated?
Where does Earth Quake?

Predicting the Next Big One!
« Previous Page Next Page »
- Investigating Earthquakes with ArcExplorer GIS
- Teaching Notes
- Step-by-Step Instructions
- Tool and Data
- Going Further
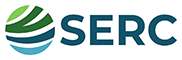
- About this Site
- Accessibility
Citing and Terms of Use
Material on this page is offered under a Creative Commons license unless otherwise noted below.
Show terms of use for text on this page »
Show terms of use for media on this page »
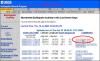
- None found in this page
- Last Modified: February 27, 2024
- Short URL: https://serc.carleton.edu/18034 What's This?
Morning Rundown: Arizona Republicans distance themselves from abortion ban, cancer institute retracts studies, ex-principal charged over 6-year-old who shot teacher
Earthquake shakes U.S. East Coast
An earthquake struck the East Coast of the United States on Friday morning, according to the U.S. Geological Survey, causing buildings to shake and rattling nerves from Maryland to Maine.
The USGS measured the quake as a 4.8 temblor with its epicenter near Lebanon, New Jersey. It struck a little before 10:30 a.m. ET. An aftershock of magnitude-4.0 hit right around 6 p.m. ET.
The morning earthquake was the strongest recorded in the Northeast in more than a decade, according to USGS records .
There were no immediate reports of major destruction or any fatalities. Local and regional officials from cities in the earthquake zone said inspections had been launched to ensure that buildings, bridges and other infrastructure were not damaged.
Follow here for live updates on the earthquake.
James Pittinger, mayor of Lebanon, New Jersey, called the earthquake “the craziest thing I’ve ever experienced.” In an interview with MSNBC , he said he had not received reports of any significant damage so far, but added that the shaking caused his dog to run for cover and objects to fall off his shelves.
While a 4.8-magnitude temblor is not considered a major earthquake, even minor shaking can cause damage on the East Coast, which does not take similar precautions as other earthquake hot spots around the world.
New York Gov. Kathy Hochul said the quake was felt across the state.
“My team is assessing impacts and any damage that may have occurred, and we will update the public throughout the day,” she wrote on X .
New York City Mayor Eric Adams said in an afternoon news briefing that no major injuries or impacts to infrastructure were reported, and that people in the city should “go about their normal day.”
Ground stops were temporarily issued at Newark Liberty International Airport in New Jersey and John F. Kennedy International Airport in New York City, according to the Federal Aviation Administration's website. Flight disruptions at the Newark airport continued into the afternoon .
The Port Authority Transit Corp., which operates a rapid transit route between Pennsylvania and New Jersey, suspended service in the aftermath of the quake.
“Crews will inspect the integrity of the line out of an abundance of caution,” PATCO said in an update on X . “Once inspection is complete, service will resume. No timeframe. Updates to follow.”
New York’s Metropolitan Transportation Authority said that there had been no impact to its service but that teams will be inspecting train lines. New Jersey Transit alerted riders of 20-minute delays due to bridge inspections following the earthquake.
While earthquakes in the northeast U.S. are rare, Buffalo, New York, was struck by a 3.8-magnitude quake in February 2023 — the strongest recorded in the area in 40 years.
A 4.1-magnitude earthquake struck the tri-state area in 2017, centered near Little Creek, Delaware, according to the U.S. Geological Survey . And before that, a 5.8-magnitude quake shook central Virginia in 2011, and was felt across much of the East Coast, forcing hundreds of thousands people to evacuate buildings in New York, Washington and other cities.
New Jersey Gov. Phil Murphy said in a post on X that the state has activated its emergency operations center and asked the public not to call 911 unless they are experiencing an emergency.
Frederik J. Simons, a professor of geosciences at Princeton University, told NBC News that the earthquake occurred on a shallow fault system in New Jersey and lasted about 35 seconds.
“The shallower or the closer it is, the more we feel it as humans,” he said.
The quake originated at a depth of less than 3 miles, according to the USGS .
Earthquakes on the East Coast can be felt at a great distance and can cause more pronounced shaking in comparison to those on the West Coast because rocks in the region are often older, harder and more dense.
“These are competent rocks that transmit energy well,” Simons said.
The earthquake ruptured within a fault zone known as the Ramapo system, Simons said. It’s a zone in relatively ancient rock that contains old faults and cracks from ancient tectonic processes. These old faults slowly accumulate stress and occasionally something slips, Simons said.
“There are cracks in it and now and then a little motion accumulates, the stress keeps growing, at very slow rates,” he said. “It’s like an old house creaking and groaning.”
Simons said this was one of the largest earthquakes in New Jersey in recent history. The last notable one was a magnitude-3.1 temblor in Freehold Township in September 2020.
“I’m on campus at Princeton University for the biggest one I’ve felt in a lifetime,” he said. “This shaking was violent, strong and long.”
Some videos captured the moment of the earthquake, including one from a coffee shop in New Jersey.
The East Coast quake struck two days after a powerful 7.4-magnitude temblor shook the island of Taiwan, killing at least 12 people and injuring more than 1,000 others. The two incidents are not thought to be related, said Dara Goldberg, a USGS geophysicist.
“We’re much too far of a distance for the stress on the fault of Taiwan to affect New York,” she said.

Denise Chow is a reporter for NBC News Science focused on general science and climate change.
Evan Bush is a science reporter for NBC News. He can be reached at [email protected].
Advertisement
Post-earthquake damage classification and assessment: case study of the residential buildings after the M w = 5 earthquake in Mila city, Northeast Algeria on August 7, 2020
- Original Article
- Published: 21 November 2022
- Volume 21 , pages 849–891, ( 2023 )
Cite this article
- Hamidatou Mouloud 1 ,
- Amar Chaker 2 ,
- Hallal Nassim 1 ,
- Saad Lebdioui 3 ,
- Hugo Rodrigues 4 &
- Matthew R. Agius 5
4092 Accesses
12 Citations
2 Altmetric
Explore all metrics
On August 7th, 2020, a magnitude Mw = 5.0 earthquake shook 5 km north of Mila city center, northeast of Algeria, causing substantial damage directly to structures, and indirectly from induced impacts of landslides and rock falls, ultimately disrupt to everyday civilian life. Given the recent significant seismic occurrences in the region, a detailed and comprehensive examination and assessment of post-earthquake damage is critical to Algeria. This is primarily because masonry, concrete, and colonial-era structures are sensitive to horizontal motions caused by seismic waves, and because masonry and concrete structures constitute a substantial portion of today’s Algeria's build environment. We present a post-earthquake investigation of the Mila earthquake, starting from the earthquake source, and a catalogue of buildings type, damage categorization, and failure patterns of residential structures in Mila's historic old town, where colonial-era brick buildings prevail. We find that structures that represent notable architectural achievements were severely damaged as a result of the earthquake. Data acquired during the immediate post-earthquake analysis was also evaluated and discussed. The graphical representations of the damages are detailed and complemented by photos. This seismic event has shown the fragility of Algeria's building stock, which must be addressed properly in future years. This study reports on an overall estimate of residential buildings in Mila's lower city, as well as an evaluation of the seismic vulnerability of three neighborhood towns (El-Kherba, Grareme-Gouga, and Azzeba). A generic database for graphical surveys and geometric research was developed and implemented making it possible to evaluate the shear strength on-site. The broad observations, collated data, and consequences were then loaded into the 3Muri structural verification program. Nonlinear static analysis was conducted to analyze probable failure paths and compare the real damage to the software results.
Similar content being viewed by others
Geological Structural Analysis Applied to Archaeoseismology
Seismic response of rc buildings during the mw 6.0 august 24, 2016 central italy earthquake: the amatrice case study.
A. Masi, L. Chiauzzi, … G. M. Verderame
Damage classification and derivation of damage probability matrices from L’Aquila (2009) post-earthquake survey data
A. Rosti, M. Rota & A. Penna
Avoid common mistakes on your manuscript.
1 Introduction
A seismic event is a rare natural occurrence that may induce enormous costs and consequences for structures, the environment, human life, and society. On August 7, 2020, at 06:15:37 UTC, an earthquake of a moderate magnitude Mw = 5.0 (Boulahia, 2022 ) and intensity VI on the European Macroseismic scale EMS-98 struck the Mila metropolitan region (Eurocode 8 2004a , b ). It was followed at 08:12:43 UTC by the strongest aftershock earthquake, with a magnitude Mw = 4.8 (Boulahia, 2022 ), and intensity of V. The primary earthquake destroyed a substantial number of structures in three areas (El-Kherba, Grareme-Gouga, and Azzeba), including both residential and public buildings. The vast majority of structures built following Algeria's earthquake code (RPA, Règulement Para-sismique Algerien 2003 ) were either undamaged or only minimally impacted by other correlated hazards, like landslides. However, most of the city (upper and lower areas) was severely affected since structures were constructed without earthquake design concerns and on unstable soils, besides, an important part of the construction was built without official approval. The destruction of buildings is considerable; over 1040 structures were affected, many of which were seriously destroyed (Fig. 1 ). At the end of the year, another moderate earthquake ( Mw = 5.2) struck Northeast Algeria, with Skikda as the epicenter, about 60 km from Mila. The seismic event triggered minor secondary impacts to already damaged constructions.
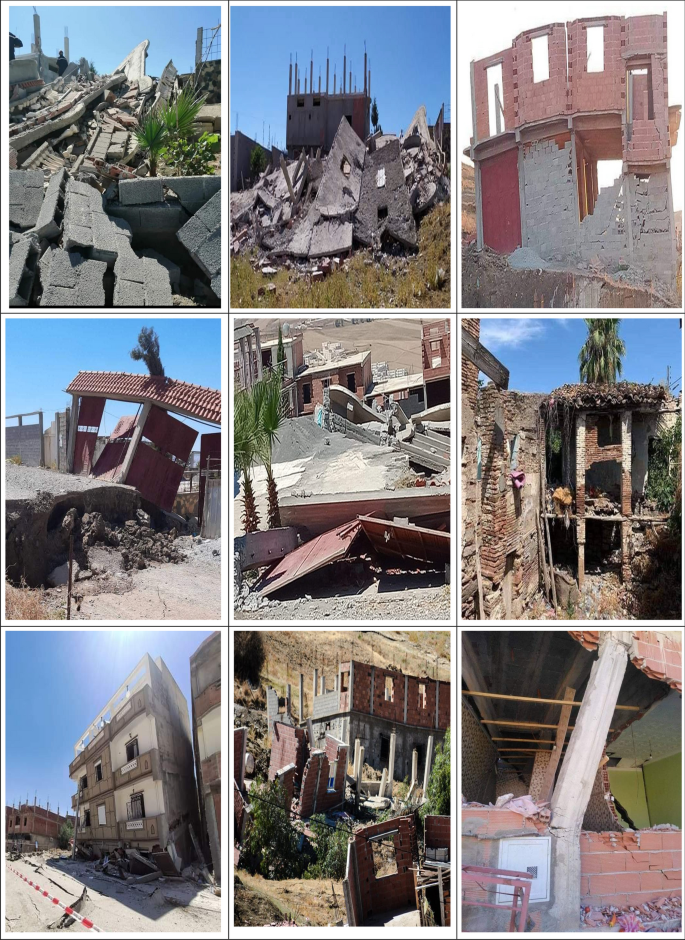
Illustration of typical structure damage following the Mila earthquake
Seismic vulnerability assessment for past earthquake in Northeast Algeria has been relatively newly initiated, starting on 1992 by Farsi and Belazougui ( 1992 ). The current work consists of regional and local studies, mainly based on seismic vulnerability assessments by (Bechtoula and Ousalem 2005 ; Laouami et al. 2006 ; Harbi et al. 2007a , 2007b ; Belazougui 2008 ; Hellel et al. 2010 ; Meslem et al. 2012 ; Mehani et al. 2013 ; Boukri et al. 2013 ; Remki and Benouar 2014 ; Hamidatou et al. 2017 ; Chimouni et al. 2018 ; Allali et al. 2018 ; Hichem et al. 2019 ; Akkouche 2019 ; Amari et al. 2020 ). We know that the first major hazard that threatens the Mila region is landslide, in this case several studies have assessed landslides’ risk as well as earthquake-triggered landslides’ risk in the region (Marmi et al. 2008 ; Atmania et al. 2010 ; Guemache et al. 2010 ; Semmane et al. 2012 ; Merghadi et al. 2018 ; Benfedda et al. 2021 ; Tebbouche et al. 2022 ; Smail et al. 2022 ; Bounemeur et al. 2022 ; Medhat et al. 2022 ).
Algeria has a vast number of modern constructions made of reinforced concrete (RC), masonry, and colonial 19th and 20th-century style buildings. Assuming that most of the ostensibly "strategic" projects of cultural and historical value are constructed of masonry, this suggests that old masonry constructions must be evaluated and repaired according to the highest standards (Atalić et al. 2019 ; Ortega et al. 2019 ; Rodríguez et al. 2019 ; Stepinac et al. 2020 ; ARES 2021 ). Pushover analysis, also known as nonlinear static evaluation, is crucial and is suggested as a reference technique in Eurocode 8–3 for such cases. Even though the engineering community has made significant advances in comprehending seismic occurrences and their consequences, there are still many unknowns and uncertainties. Earthquake engineering requires a better description of seismic motions and new robust tools for analyzing buildings and assessing seismic hazards and risks. In the previous several decades, structural standards have been carefully researched, developed, and improved to the point that they now allow for designing new types of structures while preventing major damage to human life. It is also necessary to have the ability to evaluate the response of structures to earthquake ground shaking. The seismic risk of existing masonry constructions is difficult to assess and necessitates specialized technical knowledge (Lourenco and Karanikoloudis 2019 ). Post-earthquake condition evaluation may be described as the process of determining the safety and usability of a structure following a seismic event. Many factors influence structural seismic performance, including the number of floors, roof shape, age of construction, building materials, the geometry of the structure, stiffness, strength, ductility properties, soil conditions, and seismic event characteristics. Information from multiple disciplines, such as tectonics, geophysics, seismology, geology, and civil engineering including geotechnical and structural engineering, mathematics, or applied statistics, is required.
Afterward the earthquake, the first to respond to a catastrophe were civil engineers who led and coordinated the entire organization of building assessment and construction damage analysis. Several similar post-earthquake assessment procedures are used internationally (Yavari et al. 2010 ; Marshall et al. 2013 ; Didier et al. 2017 ). In the first week after Mila earthquake, a large number of constructions were examined, with a rapid post-earthquake assessment. The most endangered buildings in the city’s center were the ones under cultural heritage protection. The aim of a rapid assessment of structures is to determine the degree of damage to buildings concerning the protection of life and property, that is, to determine if the structures are usable, temporarily unusable or unusable. Emerging technological advances allow the usage of artificial intelligence in the post-earthquake assessment process in the form of machine learning methods for more efficient and precise results (Bialas et al. 2016 ; Zhang et al. 2018 ; Kim et al. 2020 ; Natio et al. 2020 ; Stepinac and Gasparuvic 2020 ).
Mila is a rural area separated into three zones: Zone A, Zone B, and Zone C (Fig. 2 ). Mila's most ancient architectural districts are found in Zone A, which is defined by packed blocks of structure masonry, brick, or a mix of materials. The majority of structures are made out of solid longitudinal and transverse walls, brick ceiling vaults, or timber ceiling beams with reinforced concrete roofs. Some schools, commercial structures, residential and government structures, cultural institutions, and monuments are located in Zone A, and are either components of a historical city structure or stand as lone landmark buildings. The earthquake-caused landslides severely damaged 10% of the structures in Zone A. Zone B is made up of a wide range of urban designs and includes a high number of structures. The landslide zone triggered by the seismic event is Zone C, and some buildings in this area have been significantly damaged. The seismic event occurred throughout the COVID-19 pandemic lockdown and caused a critical interruption to the social restrictions adapted at that time. The research focuses on the post-earthquake investigation, damage categorization, and failure patterns of masonry residential projects in the Mila region. We present primary data acquired by onsite inspections and provide an updated and broader insight to the seismic hazard of the Mila region. We also run 3D modelling to better understand the response of building in similar conditions.
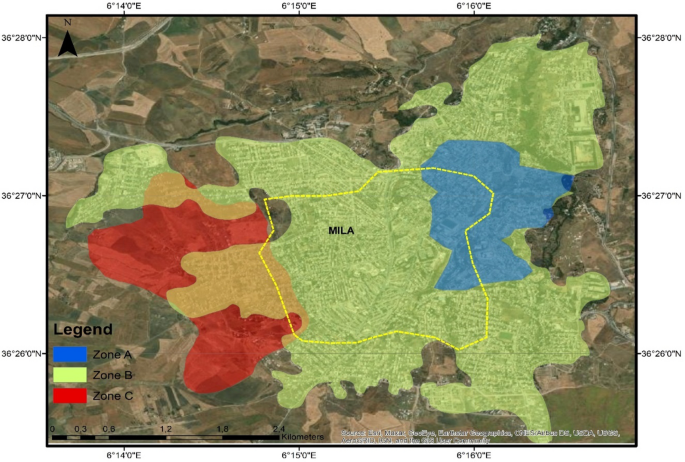
Protected areas A, B, and C, as well as the region of the analysis within the Mila (yellow dashed line)
In fact, the main shock triggered many large landslides, of which, the traces are traceable and the impact on individual houses is significant. The most important one was observed in the El Kherba region (Zone C in Fig. 2 ), a catastrophic one which caused damage to residential structures. A substantial number of structures and infrastructures in the landslide's neighbourhood has been severely damaged. According to this study in post-earthquake categorization, about 10% of structures were damaged in zone A, 15% in zone B, and 61% in zone C. Exhibiting the important effect of the major earthquake-triggered landslide in zone C.
According to Benfedda et al. ( 2021 ), six landslide zones have been identified using InSAR analysis of two Sentinel-1A images, taken before and after the August 7 event main shock. The landslides were located along a 22 km long and 6.5 km wide corridor oriented NE-SW. Furthermore, Medhat et al. ( 2022 ) detected tow landslide zone in far-separated regions, Kherba city and Grarem Gouga city using the 2D decomposition MT-InSAR approach to detect the deformation velocity before the landslide activity, retrieving displacement velocity rates up to ~ 50 mm/year. Two regions were located at 12 km apart, indicating slow motion rather than fast movement along the damaged area. In addition, Halla et al. ( 2022 under publication) three large landslides have been observed within 13 km radius. The three landslides are located South, Southwest and Southeast. The most important one is that of the El-Kherba district: the Western extension of Mila city. The second landslide is located just near the epicenter within a radius of 5 km, East of Grrarem-Gouga village. The last landslide is the least important. It is located in the Azzeba village, situated Southeast of Mila city.
A thorough and more thorough post-earthquake damage assessment is required in light of the recent devastating earthquakes in Algeria. This is particularly crucial for Algeria since the bulk of the country's buildings are made of masonry, which makes them extremely sensitive to earthquake-induced horizontal movements. An exhaustive assessment of a residential structure in Mila's lower town is given in this paper. Geometric surveys and visual inspections were both part of a comprehensive program that was developed and executed. This earthquake brought to light Mila's vulnerable building stock, which has to be mitigated as effectively as possible going forward. To minimize earthquake losses, determining the characteristics of susceptibility and evaluating the seismic performance of existing structures are crucial (Endo et al. 2017 ; Casapulla et al. 2018 ; Ortega et al. 2018 ; Valente and Milani 2019 ; Hichem et al. 2019 ; Grillanda et al. 2020 ).
2 Historical seismicity and the seismic sequence in Mila
2.1 seismicity in algeria's northeast.
Many seismic events have shaken Algeria's Northeast in the past. The strongest earthquakes were the tsunamigenic earthquakes that struck the city of Jijel (previously Djidjelli) on 21 and 22 August 1856, with an intensity of X on the EMS scale, and affected Djidjelli and the nearby region (Harbi et al. 2011 ). According to ancient sources, the earthquake generated a tsunami and caused extensive damage in the city, with more than 30 people killed and many collapsed structures. Figure 3 is a map of major earthquakes in northern Algeria with their surface magnitudes Ms . Only a few significant earthquakes have happened in Algeria's northeastern region in more than a century. As a result, public awareness and readiness were at an all-time low. Though authorities and scientists have warned for years about the repercussions of a catastrophic earthquake and about the importance of planning for a swift response after an earthquake (vulnerability assessment, rescue and care of people, damage assessments, etc.…), preparedness actions have been limited (Atalić et al. 2019 ; Stepinac et al. 2020 ).
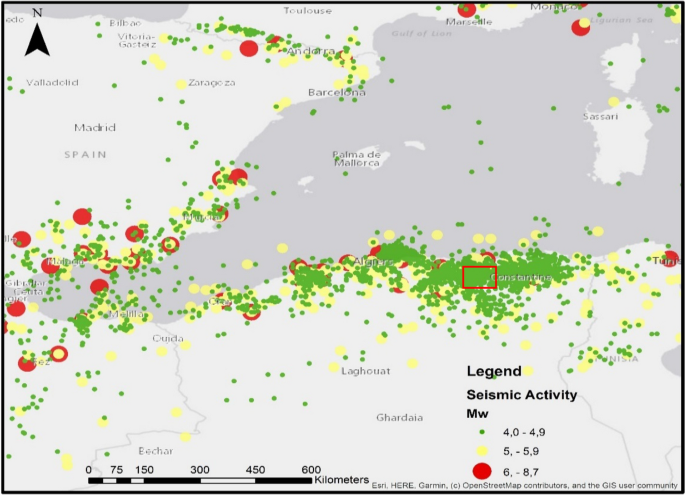
Strong earthquakes in Algeria's Northeast and surrounding regions in the previous century from 1900 to 2021, the red square indicates the Mila region (Hamidatou et al. 2017 , 2019 , 2021 )
Mila is located in the NW of the Constantine basin, which, with its tectonic configuration, is the key cause of earthquakes in the zone (Durand 1969 ; Raoult 1974 ; Vila 1980 ; Coiffait 1992 ). The 1985 Constantine earthquake (Ms = 6.0, Ousadou et al. 2012 ), the worst seismic event registered in the area, triggered substantial damage in the city and was felt in the Mila area. According to historical records, the earthquake produced extensive damage to constructions (108 structures were damaged) and caused two fatalities and injured 10 inhabitants (Bounif et al. 1987 ). The 1985 earthquake was a watershed moment in Northeast Algeria’s development and city planning. However, since strong seismic events occur across relatively extended time periods, the lessons of the past are easily forgotten. Even though 50 percent of the North Algerian region is vulnerable to severe seismic shaking, and 70% of the population lives in this region, the public’s risk awareness is low.
2.2 The seismic sequence in Mila on July–August 2020
In the period including July and August 2020, the Mila region witnessed a seismic sequence that was marked mainly by the appearance of three important shocks. Recently, Benfedda et al. ( 2021 ) studied the main events of the July–August 2020 Mila seismic sequence, including, respectively, the events on July 17 at 08:12 UTC (Mw 4.6), August 7 at 06:15 UTC (Mw 4.8) and at 11:13 UTC (Mw 4.4). More recently, Boulahia ( 2022 ) examined the first shock of July 17, 2020 at 08:12 UTC of Mw 4.8, located 1 km North of Sidi Merouane (near Grarem-Gouga area), a second shock on the August 7, 2020, at 06:15:37 UTC, where he stated a Mw magnitude of 5.0, and a third shock at 11:13:27 UTC of a Mw 4.5 (Boulahia 2022 ). The main shock (the second one) had an intensity of VI according to the EMS-98 scale, it also triggered a spectacular landslide in the El Kherba region. This landslide caused significant damage to individual buildings (Fig. 4 ).
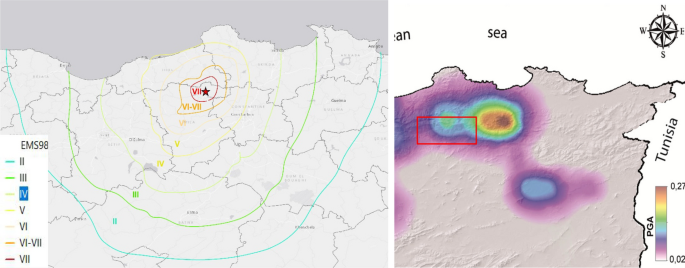
Preliminary Earthquake Intensity Map (left) from the earthquake of August 7, 2020, at 6:15:37 (UTC) compared with predicted peak ground accelerations (right) for a return period of 475 years (Hamidatou et al. 2021 ), the red square indicates the Mila region
Benfedda et al. ( 2021 ) performed a waveform inversion of the accelerograms to calculate the seismic moment, moment magnitude, and focal mechanisms of the three main seismic events:
The July 17th, 2020, Mo = 1.019E + 16 Nm, Mw = 4.6, h = 5 km.
The August 7th main shock, Mo = 1.794E + 16 Nm, Mw = 4.8, h = 8 km.
The August 7th aftershock, Mo = 4.653E + 15 Nm, Mw = 4.4, h = 12 km.
They determined focal mechanisms generated a pure strike-slip solution for the three events with nodal plans oriented NE-SW and NE-SW and a pressure axis oriented N-S.
In addition Boulahia ( 2022 ) used an Empirical Green’s Function (EGF) method to derive the Relative Source Time Functions (RSTF’s) and high-resolution relocation to active structures and analyzed the spatiotemporal behavior and mechanics of the sequence. They managed to separate the initial seismic cloud into two densely concentrated spatial clusters of strongly correlated events, and were able to detect components of directivity toward the southeast for the shock (Mw 4.8) and directivity toward the northeast for the mainshock (Mw 5.0).
The July 17th, 2020, Mo = 2.14 × 1016 N.m, Mw = 4.8.
The August 7th main shock, Mo = 3.14 × 1016 N.m, Mw = 5.0.
The August 7th aftershock, Mo = 0.67 × 1016 Nm, Mw = 4.5.
They relocate over 981 events from the sequence, with structures matching moment tensor solutions and focal mechanisms indicating predominantly right- and left-lateral strike-slip ruptures. The results reveal orthogonal conjugate structures—one trending ~ N65W and dipping 80°SW, and one trending ~ N28E, 70°NW-dipping fault plane. The earthquakes evolved in two phases, with a spatiotemporal migration of epicenters from the NW–SE fault plane to the NE-SW fault at a rate consistent with pore fluid diffusion (Boulahia 2022 ).
Furthermore, Benfedda et al. ( 2021 ) recorded the peak ground accelerations (PGAs) of the three major events in Mila by the local network. The Beni-Haroun station recorded the maximum accelerations for all events. The Beni-Haroun huge dam reservoir is just a few meters away from the BHAR station, which is situated in a free field and on hard rock on the NW side. The high values of ground motion of these relatively small earthquakes are explained by the near field and shallow depth of the seismic events. Hard rock often records such high acceleration values quite near to the hypocenters (Laouami and Slimani 2018 ).
The earthquake's epicenter was located at latitude 6.28 N and longitude 36.54E. The epicenter was located around 13 km Southwest of Mila center, in the Hamala area, at a depth of 7 km. The strongest aftershock occurred at 8:12:43 UTC, with an Mw magnitude of 4.8 (Boulahia 2022 ) and EMS-98 intensity V. The epicenter of this aftershock was about 10 km Southwest of Mila's center, at a depth of 10 km. On the same day, five aftershocks with Mw magnitudes larger than 3.0 and EMS-98 intensity V were observed. Following that, the city of Mila and its surroundings have been hit by a series of mild and medium aftershock earthquakes (Fig. 5 ).
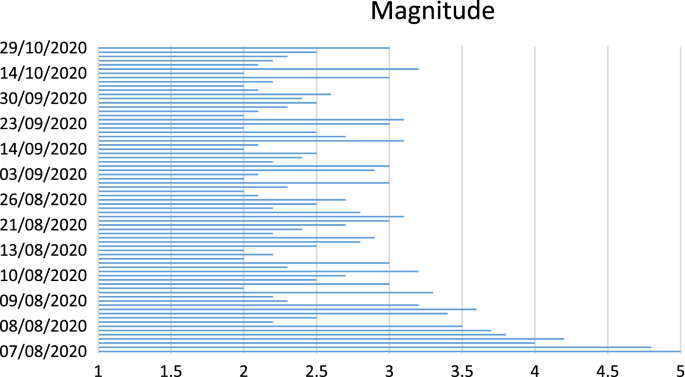
Earthquakes with a magnitude more than 2.0 ( Mw ) occurred in Mila between August 7 and October 30, 2020
Even though the seismic event was of moderate magnitude, it caused a large amount of measurable damage. Seismic risk assessment in urban areas and building vulnerability are usually misread, therefore the majority of community media (and a section of the technical community) have consistently reported on the disparity between the magnitude of the earthquake and the degree of damage. Assuming that a thorough and complete seismic risk assessment for the city of Mila has not been conducted, such reporting is not unusual. Given that the PGA for the historic core of Mila, which was the subject of prior studies, was about 0.19 g, and that most of the structures are quite vulnerable, widespread damage should have been predicted (Hamidatou and Sbrtai 2016 , 2017 ; Hamidatou et al. 2019 ; 2021 ). The primary earthquake destroyed a substantial number of old urban structures, including residential buildings, administrative offices, colleges, and public institutions.
3 Procedure for immediate response and post-earthquake evaluation
The seismic event in Mila occurred during the strong restrictions imposed following the first wave of the COVID-19 pandemic, which imposed extra constraints on rescuer and researcher operations. Immediately following the earthquake, a multidisciplinary post-seismic disaster management team visited the Mila City Crisis Management Agency to examine the damaged state of the constructions and the possibility of their continued use in a timely manner. The Civil Protection Center was activated, and researchers, specialists, and experts from research centers and universities, as well as the CRAAG, were hired to organize work related to field condition assessment, installation of the seismic network and Global Positioning Systems (GPS), geological and geophysical field missions, rapid engineering assessment training, and the organization and development of an information system for dealing with disasters. All activities were conducted in partnership with the Ministry of the Interior's Civil Protection Directorate and the City of Mila's Civil Protection Authority.
Based on Italian results, a prototype technique for post-earthquake damage and useability study was proposed (Baggio et al. 2007 ) and EMS-98 (EMS 1998 ), because knowledge of seismic occurrences in northern Algeria is low, vigilance for such events and immediate actions was also poor. Until now, data on the number of structures, floor layouts, cross-sections, building materials, or function at the time of the earthquake were not available. The post-earthquake damage analysis includes a quick visual evaluation of each structural system, an indication of the degree of damage, and the categorization of the structure into each of six sections:
CN1 (dark red color): Not feasible due to external hazards—The construction is dangerous due to the likelihood of major portions of a nearby structure collapsing. It is advised not to remain in such facilities given the considerable number of aftershocks.
CN2 (red color): Unusable owing to damage—The structure is dangerous due to substantial structural damage, collapse, and failure. The structure has reached the limit of its loadbearing capability and ductility and cannot be utilized in any way. That does not always mean that the building must be destroyed.
PCN1 (dark yellow color): Possibly unusable—Full assessment required—The structure has a fair extent of the damage but no risk of collapse. The loadbearing capacity has been partly reduced. A shorter visit to the structure is conceivable, and a structural engineer should provide suggestions for future repair work.
PCN2 (yellow color): Temporarily unusable- Emergency rehabilitation measures required—The structure has some damage with no probability of collapse, but cannot be utilized owing to the possibility of failure of some structural components. The structural engineer is aware of emergency response methods and must give instructions to users. The structure, or a portion of it, is inoperable until the safeguards are put in place. Provisional usability may apply to elements of the structure (components) only.
CU1 (dark green color): Usable without limitations—There is no damage or only minor damage that does not jeopardize the structure's load-bearing ability and usage.
CU2 (green color): Consider Protective Measures.—Except for some elements where there is an immediate risk to a portion of the structure, the structure can be used. The building evaluator can grant authorization for sthe risk to be removed and advise the occupants to impose temporary residential limitations on specific portions of the structure. The structure can be used freely after the risk has been removed.
After Day 1, Class CU2 was used in the procedure because non-structural components of the structures were damaged and may endanger passers-by and members of the public. It was essential to eliminate these components as quickly and efficiently as possible. The structure was safe to use once the non-structural damaged components were removed.
The building typologies, structural damage, and failure patterns caused by the earthquake or landslide in the Mila city center area are depicted and discussed in the following sections. The brick structures that make up a considerable proportion of the city center and the surrounding area are given special attention. This study focuses on the typical damage and disaster to residential structures. Significant advancement has recently been achieved in understanding the seismic behavior of masonry buildings and analysis during seismic occurrences (Binda et al. 2000 ; Ortega et al. 2017 ; Vlachakis et al. 2020 ). It is hoped that the current data will positively contribute to further development in this field.
4 Typology of constructions in Mila
Mila's buildings typically consist of roughly 91,000 residential constructions and 5,000 non-residential constructions, according to the Algeria Population and Housing Census 2008, provided by the National Office of Statistics of Algeria. More than 20% of the construction stock is over 40 years old. The use of traditional materials and building techniques, like masonry and timber, is a defining feature of such older Mila structures. Most of the people in Mila province, particularly in the cities, work and reside in colonial constructions, especially in Mila's city core.
Throughout the city's and the surrounding areas’ history, numerous types of structures have been constructed depending on the advancement of construction technology, understanding of soil qualities, and urban planning, including urban protection measures and the demand for building areas. Knowing when a set of buildings were built provides a reasonable estimate of their seismic strength following the 1985 Constantine earthquake. Most of the structures in Mila's old city center are concrete and masonry constructions with timber floors and roofs. A single-story typical height varied from 2.5 to 3.7 m.
They followed RPA norms from 1996 to 2003, and after 2003, they followed RPA regulations (RPA 2003 ). As a result, many structures were built without any proper lateral force resisting system before any seismic regulations were implemented. In terms of floor systems, timber in older houses and reinforced concrete in later constructions are the most common options. Timber floors are more flexible than rigid concrete slabs. Because the connections between walls and floors are typically weak, this makes walls more susceptible to potential out-of-plane failure mechanisms.
New structures with four or more stories have been built in the city throughout the previous two decades. They were designed to withstand earthquakes. A mix of historic individual structures and new apartment towers distinguishes the urban area. Most single-family houses in Mila's immediate vicinity are one- or two-story brick structures. Contained masonry with reinforced concrete floor constructions is more recent than unreinforced stone masonry constructions with wood or reinforced concrete floors. About 17 percent of dwelling units in Mila were built before the first seismic code was enacted (1982), Fifteen percent of housing units were designed following the first seismic code (1962–1996), and 29% were built using the enhanced seismic code (Table 1 ). The RPA 2003 code was used to design housing units built after 2003.
Mila's historical city compound is a conservation area under the Act on Culture and Heritage Conservation and Maintenance. Zone A and Zone B are the two zones that make up the region (Fig. 6 ). The oldest and most architecturally key areas of Mila are in Zone A, which is the subject of this study. Both locations, however, have architectural and historic landmarks and are characterized by densely packed blocks of constructions using stone brick or a mix of materials. Many schools, businesses, residential and government structures, social organizations, and mosques are in Zone A and are conserved as part of a historical city structure or as separate historical sites, although they are not the subject of this research. Zone B contains the remaining parts of Mila's historic urban complex. It contains a wide range of urban forms as well as a considerable number of historically significant structures.
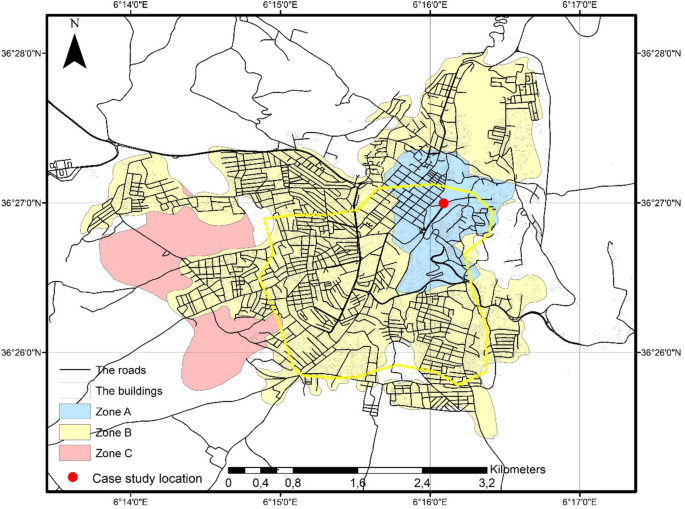
Heritage-protected zones in Mila (Zone A, Zone B, and Zone C). The yellow dashed line indicates the study's observation area
Once most of the buildings are constructed as part of bigger blocks, their sides are frequently the width of a leaf. The same building strategy was utilized even when the structures were built inside the blocks, i.e., as freestanding units, and these freestanding structures were severely damaged.
The inadequate connections between walls and floors are also observed, and since the floor structure is primarily wooden, the structures lack the so-called box-type behaviour. Timber flooring prevails despite the presence of multiple composite wooden concrete compound buildings. Because group of unreinforced masonry (URM) buildings are prone to damage from seismic excitations (Palazai et al. 2022 ), the damage was observed in a large number of structures following the Mila earthquake. The typical roof structure is a king or queen post truss; however, there are many distinct types of integrated timber roof constructions (Fig. 7 ). Due to rehabilitation work, a concrete slab beneath the roof systems can be found in a limited number of structures. Even though tie rods have been used for many years over the world, they were not widely used in Mila. Timber-reinforced masonry was used in very few examples in the ancient city core. After 1980, numerous new concrete constructions were put within the old downtown's existing building blocks, a trend that still exists today. These structures were either unaffected by the earthquake or suffered only minor non-structural damage. Newer buildings, on the other hand, tended to have more stories and less interstory height than older buildings, which clearly influenced the seismic response of neighboring structures (Fig. 8 ).
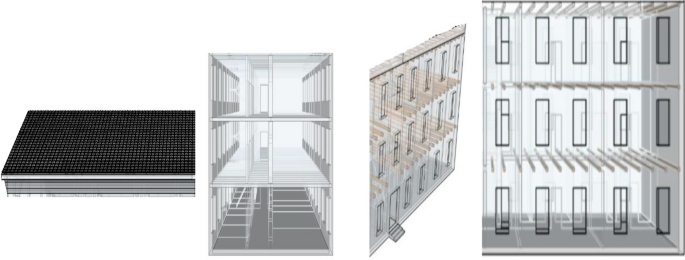
Typical residential structure (schematic representation)
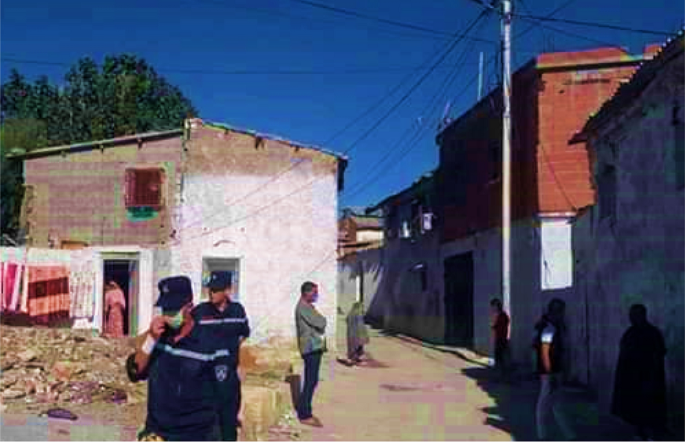
Insertion of new concrete constructions "within" existing blocks during the building process and at the end
The lack of maintenance was identified as a key factor in the condition of structures following the earthquake. Because many structures were poorly or never maintained, the masonry strength deteriorated with time, the connection between the masonry and the walls was degraded, and the seismic performance of such buildings worsened. Water infiltration damaged the characteristics of both masonry and wood elements in numerous cases.
5 Case study: Sidi Ghanem Mosque
We studied the Sidi Ghanem Mosque, a building located in historical Mila city (Figs. 9 and 10 ). The Sidi Ghanem Mosque is the oldest mosque in Africa. Mila was once a Roman settlement. The Umayyad Arab forces arrived in 675 CE, about 59 AH. Under the direction of Abu al-Muhajir Dinar, they seized the city. That same year, it appears, the order was made to clear a site adjacent to a Christian Basilica and to build a mosque. The basilica had an abundance of building materials, stones, and columns that could be reused. The mosque, on the other hand, did not mirror the familiar Roman basilica or the Roman city street architecture, but it had an important meaning. Its 62 m-high minaret, for example, was constructed with 365 steps, representing the number of days in the year. The mosque of Abu al-Muhajir in Mila does not have a marked orientation. Here's a blueprint for the structure. It began as a mosque, but was later turned into a workshop and, finally, a hospital.
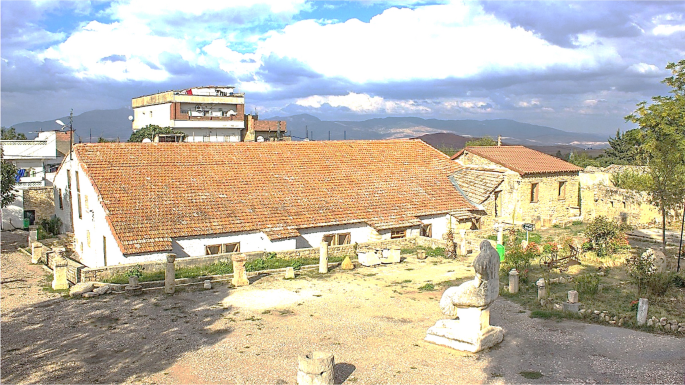
North façade view of the Sidi Ghanem Mosque
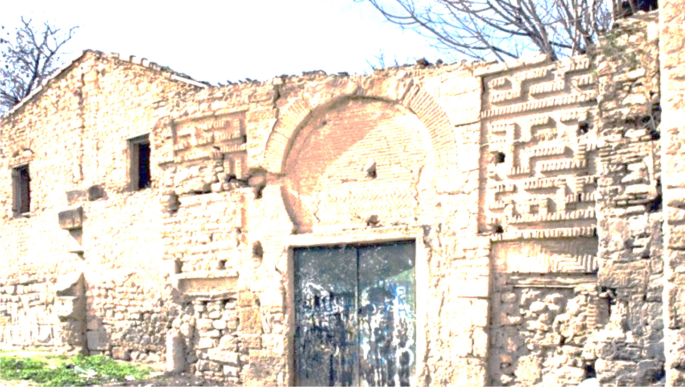
South façade view of the Sidi Ghanem Mosque
The Roman basilica was demolished to make way for the mosque to be rebuilt. The main construction is made of reclaimed materials from the old town. The reuse of Roman columns and marquees allowed the mosque to have more robust construction. The other materials used were full-size bricks made on-site. There are visible elements of a second-story building that the French-built to house the soldiers.
The state of disrepair of the Sidi Ghanem Mosque was worsened by the Mila earthquake. The study of the monument’s restoration, which began in 2019, focused in its first phase on "the state of the sites and emergency measures," in light of which emergency work was undertaken.
According to the Algerian seismic hazard map (Hamidatou and Sbartai 2017 ; Hamidatou et al. 2019 , 2021 ), for a return time of 475 years, the peak ground acceleration at the building site is 0.197 g. The building serves as an educational-cultural institution. Prior to the earthquake, the structure's state in terms of vertical loads was adequate and well maintained.
6 Residential building damage and common failure patterns
The earthquake severely damaged key architectural achievements and shattered Mila's historically identifiable city center. The vast majority of structures were constructed after the first mandated earthquake rules (i.e., during the 1980s) withstood the earthquake unscathed or with only minor damage. However, many structures in the historic district (Upper and Lower Town) were severely damaged. It is estimated that about 10% of the entire building stock was damaged in Zone A and more than 15% in Zone B—a total of 540 damaged buildings. However, in Zone C, 61% of the buildings were damaged, with a total of 743 building, i.e., more than the total of the damaged structures in Zones A and B gathered, exhibiting the substantial effect of the major earthquake-triggered landslide in zone C. (Table 2 ).
According to the previously mentioned post-earthquake categorization (red or N, yellow or PN, green or U), of 136 damaged structures in zone A, 97 were green-tagged, 35 were yellow-tagged, and 4 were red-tagged, whereas in zone B, of 404 damage structure, 342 were green-tagged, 55 were yellow-tagged, and 7 were red-tagged. However, in Zone C, among the 743 damaged buildings, 515 were green-tagged, 137 were yellow-tagged, and 91 were red-tagged. In Fig. 11 , the residential structures with usability tags are depicted on a map of the historical city center, whereas Fig. 12 depicts a typical building block. In this block, 17 buildings were green-tagged (13 percent of all damaged buildings), 20 (17 percent) were yellow-tagged, and 3 (4 percent) were red-tagged.
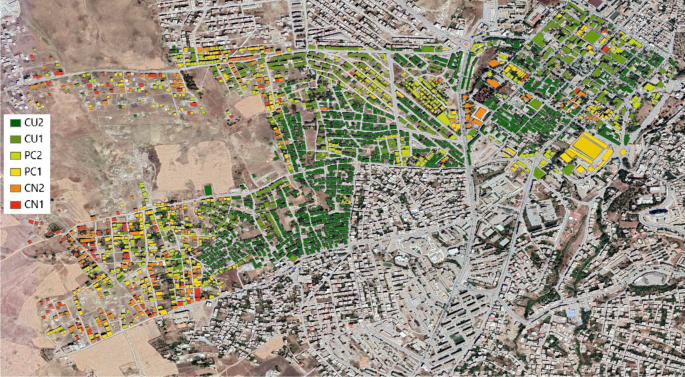
A detail of Mila city's building usability rating
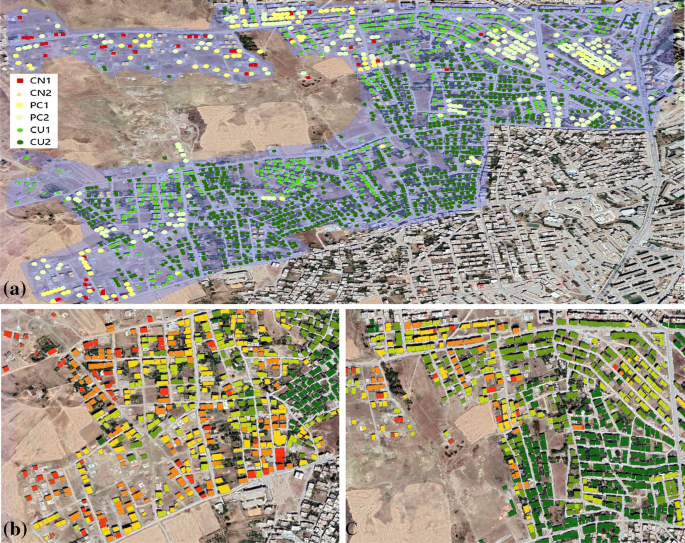
Post-earthquake damage assessment for one typical block of buildings
The historic downtown is made up of blocks of aggregated buildings, a damage map for each one has been prepared (Fig. 13 ). The goal was to gather information on the risk of individual blocks as well as a prospective seismic vulnerability assessment for certain sections of Mila. Although it was assumed that blocks further away from the epicenter would be less damaged, the map in Fig. 13 reveals a significant spread and random outcomes.
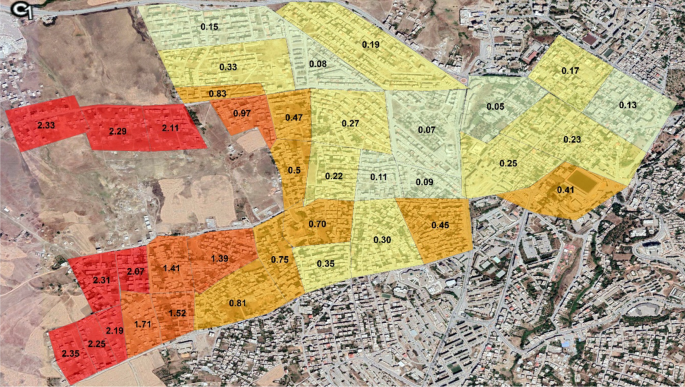
Average damage index for each block of buildings
According to our post-earthquake assessment analysis, each individual building in the block was assigned a number on the map. CU1 buildings were assigned an index of 0.5, CU2 buildings were assigned an index of 1, PCN1 and PCN2 buildings were assigned an index of 3, and CN1 and CN2 buildings were assigned an index of 5.
Ni: Number of structures in each calculation mesh assigned to a damage category.
Based on the information received; these figures are enough for a basic indicator of damage or average index can be computed for the entire block. For the block depicted in Fig. 12 , the damage index is computed as a weighted average depending on the number of individual structures, as \(\left[ {{12} \times 0,{5}\left( {{\text{CU1}}} \right) + {25} \times {1}\left( {{\text{CU2}}} \right) + {4}0 \times {3}\left( {{\text{PCN1}} + {\text{PCN2}}} \right) + {9} \times {5}\left( {{\text{CN1 }} + {\text{ CN2}}} \right)} \right]/{86} = {196}/{86} = {2},{28}\) .
It should be emphasized that the damage information should be viewed with caution because the criteria of various engineers were often subjective and deviated from the evaluation guidelines.
The choice to consider PN1 and PN2 designations, as well as N1 and N2 with the same damage index is also justified. Although the results may not provide an entirely accurate image of the damage in the city, they do provide a reasonable indication of which areas are most vulnerable to earthquakes. The immediate post-earthquake evaluation revealed unique structural damage typologies. Non-structural components such as chimneys and ornamental elements on facades were found to be losing their structural resistance and stability on every structure in the city center. These factors resulted in further damage to the building exterior or structural damage to nearby structures, as well as water intrusion inside the premises. Secondly, the loss of structural strength and stability of structural components jeopardized the structural integrity of entire structures. Gable walls, masonry columns, portions of walls between or under windows, vaults, ceilings, and stairs are the most frequently encountered damaged elements. Some of these features also harmed roof systems, which frequently became unstable because of the collapse of individual load-bearing walls underneath them.
Individual buildings, particularly those within the blocks of buildings, suffered significant damage and had uncertain structural resistance and stability. Table 3 shows the prevalence of observed masonry structure damages and load-deformation in Mila. The structural damage observed is discussed in the following section with graphical interpretations and images of the affected buildings. There are examples of in-plane, out-of-plane, and mixed damage or failure mechanisms.
6.1 Structural damage
Masonry is a well-known heterogeneous, composite material with low tensile strength and significant self-weight, making it difficult to sustain earthquake-induced stresses. Learning from past occurrences can help us design better new structures and make post-earthquake evaluations easier. The Mila earthquake caused damage to clay brick masonry constructions similar to those observed in Japan and Italy (Penna et al. 2014 ; Binda et al. 2000 ; De Luca et al. 2018 ) and Turkey after recent earthquakes (Karimzadeh et al. 2018 ; 2020 ). Most of the residential structures in Mila's old town were constructed with URM. To prevent masonry failure, proper detailing and strengthening should be employed. However, this was not always the case in older structures. Even though “zero mechanism” was frequently mentioned in many post-earthquake evaluations in African nations (i.e., the disintegration of the material) (Indirli et al. 2013 ), due to the construction type, i.e., solid brick structure, this mechanism was not detected during the Mila earthquake. The partial collapse or overturning of gable walls was one of the most typical failure types in Mila's residential structures. Gable walls are often just 9 cm thick. In addition to the attic gable walls, the parapet walls were frequently damaged, but to a lesser extent. The preceding part depicted the roof type, and it was observed that the connections between the timber framework and the walls were frequently insufficient or non-existent. Roof rafters and ridge beams frequently collide with gable walls, causing substantial damage and failure due to their weight and magnified accelerations at the gable's height. The same thing happens with the mezzanine wood structure (which rests on the longitudinal walls) and sidewalls—the wall and horizontal parts are not or are very poorly attached. Figure 14 depicts damage examples from the most prevalent gable wall collapses.
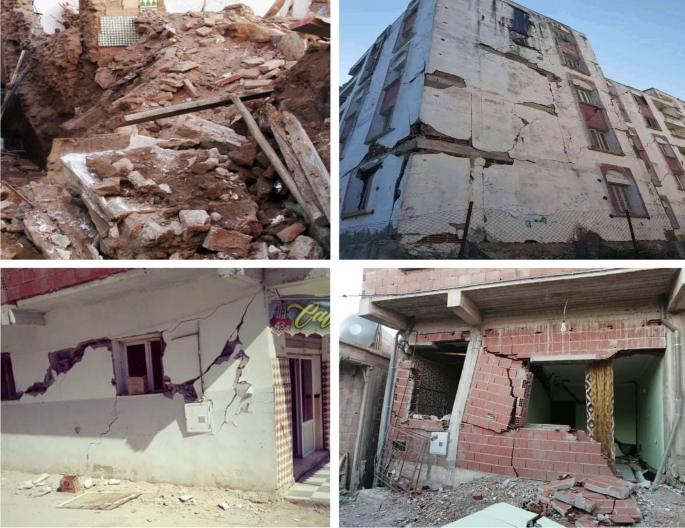
In-plane and out-of plane damage
Many buildings have an architectural design that includes protrusions for entrances or other floor plan irregularities. Cracks frequently emerge at the junction of orthogonal load-bearing walls in these areas; torsion ensues, which can compromise the building's overall stability. Because building entrances with stairs are widespread in these locations, the staircases and related load-bearing walls are frequently damaged. Figure 15 depicts one example.
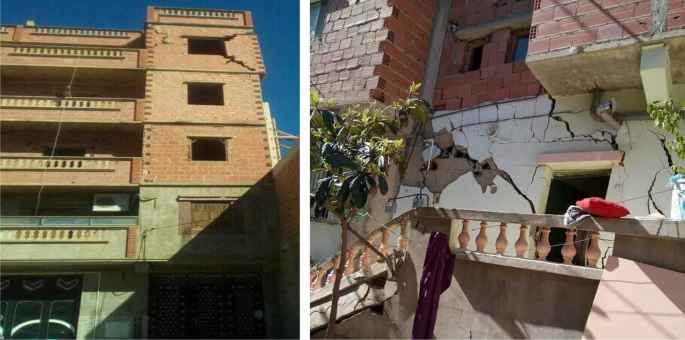
Plan irregularities caused damage to the connecting components
6.2 Non-structural damages
The Mila earthquake caused the most damage to non-structural elements. Partition walls are typically composed of 9 cm-thick masonry but can be as thin as 3.7 cm without plaster. The degree of damage to partition walls ranges from minor plaster damage to severe collapse and failure (Fig. 16 ). Non-structural walls are sometimes built on the shorter side of the brick (shiner) and are likely to be destroyed after an earthquake. Although damage to partition walls may not result in a permanent reduction in structural strength and stability, it can nonetheless represent a risk to the building's performance and peoples’ safety. Many buildings in Mila have such walls that are exceedingly high and long. This makes them comparatively thin, and their collapse can endanger people. The massive damage to partition walls reflects a major economic loss as well. Certain institutions with huge floor areas have partition walls that have sustained so much damage that cost–benefit assessments have shown that repairing them is not profitable. The building approach was to stack partition walls on top of one other, transferring no load to the slab. If the first-floor wall is destroyed or lost, the weight of the second floor (and higher levels) will be transmitted to the first-floor slab.
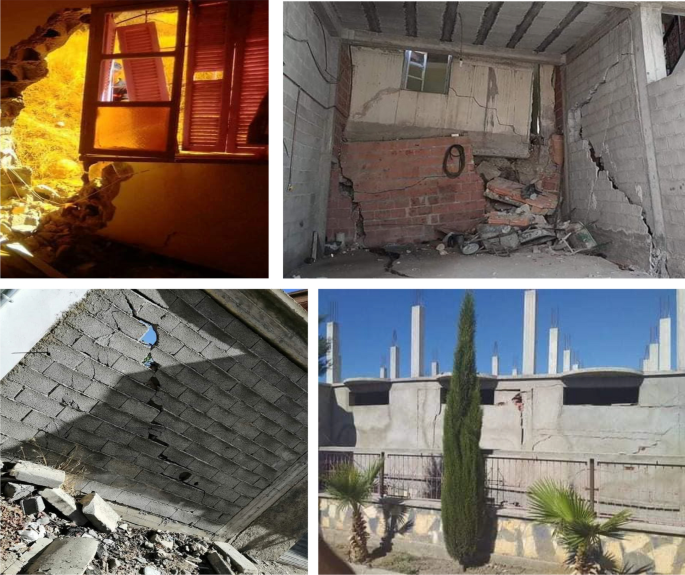
Damage to partition walls
Conversion of non-residential spaces to residential spaces is a common adjustment to building spaces (such as basements, attics, or service rooms). Partition walls were frequently eliminated during such renovations, undesired intrusions in load-bearing walls are also common. It is often assumed that if a steel beam is installed on top of the opening to replace the load-bearing wall, nothing will happen to the structure. Elements of the roof structure are frequently removed when transforming an attic into a living area since they interfere with the intended usage (Fig. 17 ). Roof structures have been extensively modified during the conversion of attics to residential use. Timber tie components were frequently cut to install a door (Fig. 17 ), creating significant alterations to the roof structure's basic structural system.
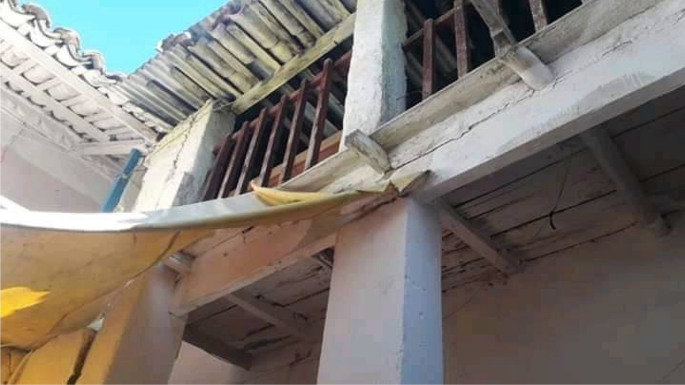
An example of an attic conversion for residential use
Stairs in residential structures are often positioned in the center of the floor layout, with various residential units on either side. Stairs are not necessarily non-structural features, but in the case of brick structures in Mila, they are not critical for seismic force resistance. Stairs can be monolithic at times, although they are usually fixed to the walls on one side and supported by steel profiles on the other. Many stairs lack a supporting beam and are not monolithic, as seen in Fig. 18 (right). Staircase walls are often thicker and part of a rigid core. The most typical staircase damage is the separation of individual stairs, the falling of plaster (sometimes as thick as 3 cm), and the separation of the stairs from the walls (Fig. 18 ).
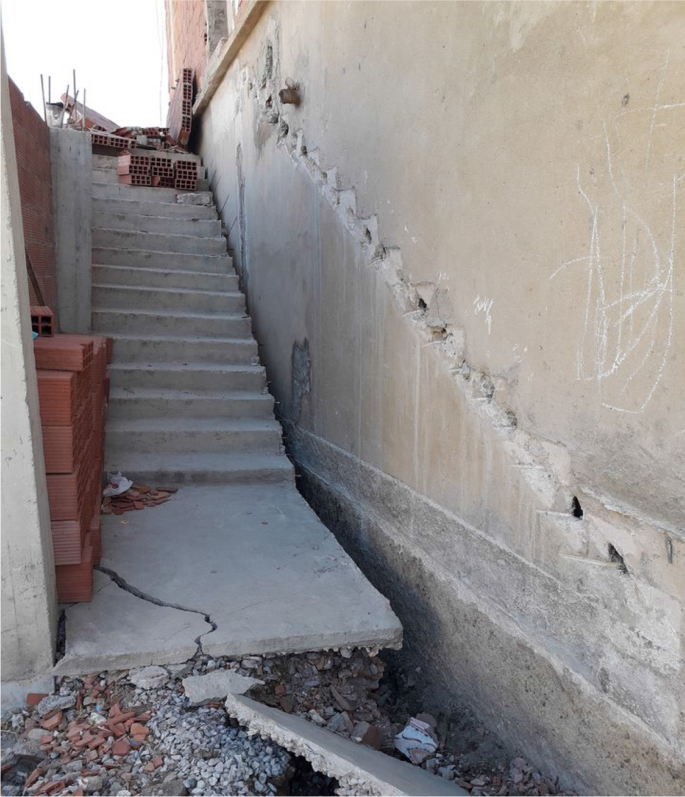
Typical stairwell damage
Many staircases were built cantilevered into the wall, resulting in substantial step separations during the earthquake. Finally, in older structures, elevator shafts served as partition walls and were frequently damaged (Fig. 19 ).
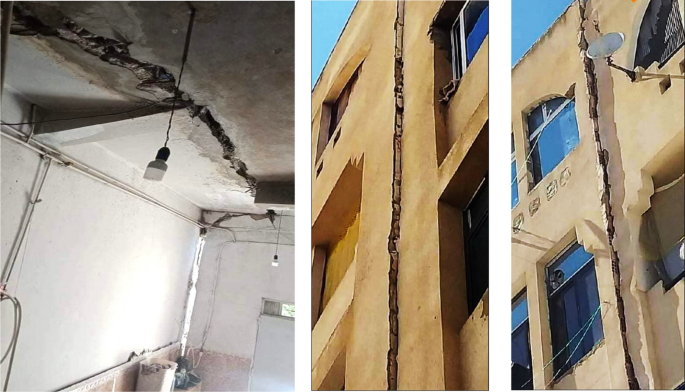
Typical elevator shaft damage
7 Methodology
7.1 assessment procedure.
A quick, preliminary assessment of the continued useability including all structures affected in the earthquake is the first step in a thorough post-seismic building evaluation (Stepinac et al. 2017 ; Uroš et al. 2020 ). The information thus collected may also be used to evaluate old infrastructure and build a modeling and simulation model. The following studies employed similar technologies (Dall’Asta et al. 2019 ; Betti et al. 2021 ). When required, detailed assessment and available Non-Destructive Testing (NDT) assessment methods are preferred (Stepinac et al. 2020 ). With consideration of the safety of civil engineers in the field, a quick initial survey is carried out as quickly and efficiently as possible following the earthquake. In Algeria, this type of evaluation entailed a quick visual inspection of each load-bearing structural component and an assessment of the level of damage, and the assignment of the building into one of six potential categories (Fig. 20 ):
CU1 Unrestricted use possible (Dark Green label),
CU2 Useful with suggestions (Green label),
PCN1 Unusable for the time being, a thorough examination is essential (Dark Yellow label),
PCN2 temporarily ineffective (Yellow label),
CN1 Due to extraneous influences, it is no longer usable (Dark Red label), and
CN2 Due to damage, it is no longer useable (Red label).
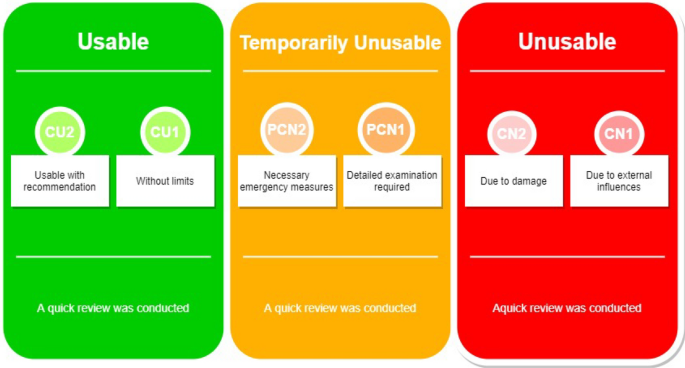
Six categories, each with a unique label
7.2 Detailed assessment results
On August 8, 2020, within the first 24 h after the earthquake, a rapid evaluation of the case study building was conducted. Following a quick thorough observation of load-bearing structural components, the building was deemed temporally uninhabitable (Dark Yellow label), with a suggestion for a further assessment (PCN1). The preliminary assessment's main findings are as follows:
On all levels, there is apparent damage in the form of cracks in the wall coverings, arches (Fig. 21 a), vaults, and ceilings (Fig. 21 b);
Plaster separation and localized damage;
Structural components (walls, columns, and arches) have minor damage;
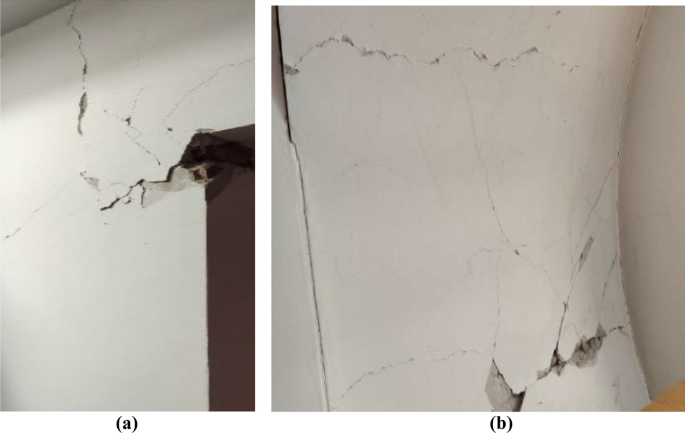
First floor cracks: wall, lintel ( a ), and ceiling ( b )
In the eastern part of the structure, diagonal cracks are apparent on load-bearing walls.
The second floor and attic had slight damage, but the eastern (Fig. 22 a, b) and central staircase wings received the most serious damage (Fig. 23 a, b). The structure should be used with caution in areas where there is a risk of plaster collapsing, according to the instructions. Furthermore, while the eastern and western stairs can be used with a limited number of people, the central staircase cannot be used until a comprehensive assessment has been conducted.
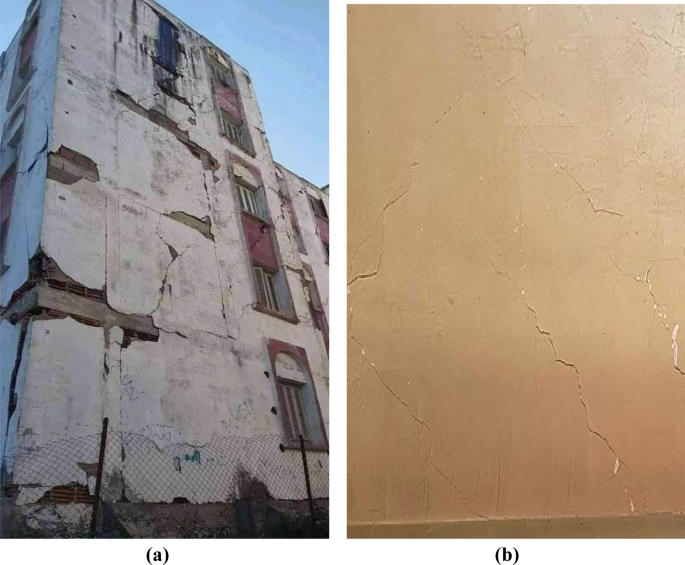
Exterior a and internal b cracks on the eastern stairwell
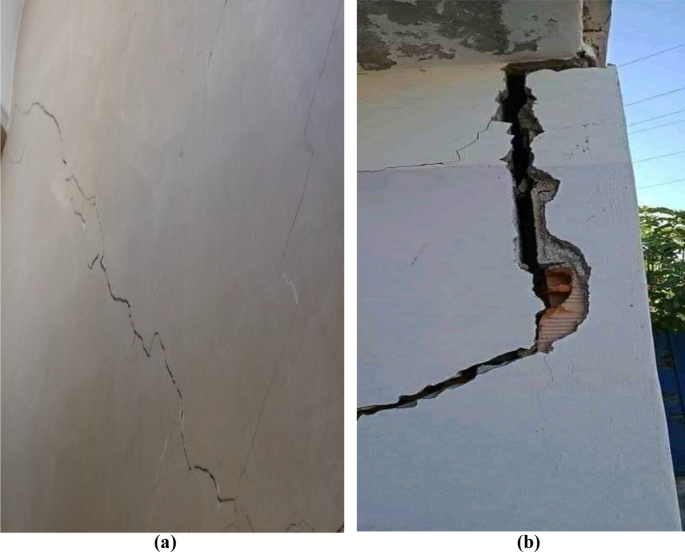
Exterior a and internal b diagonal cracks on the central staircase
The buildings under consideration are in the range of peak ground acceleration of 0.199 g, according to current structural engineering standards shown by RPA ( 2003 ), and governmental codes—which means the expected earthquake intensity is X on the EMS-98 scale for a return period of 475 years. Furthermore, a seismic hazard map for the Mila region was developed according to RPA 2003 criteria based on the latest findings from research conducted by CRAAG in collaboration with the University of Skikda (Hamidatou et al. 2017 ). The soil in the immediate area of the evaluated structure falls into the category of soil type C, according to the specified seismic hazard study (2017–2019).
The damage observations are depicted on the building’s floor plans (Figs. 24 and 25 ). The building was evaluated from the air using an unmanned aerial vehicle, and no damage to the primary load-bearing structure or the roof structure was found. Also examined were decorative crosses, figurines, and reliefs. The 3D model of the building was created using photogrammetric photographs for digital preservation reasons.
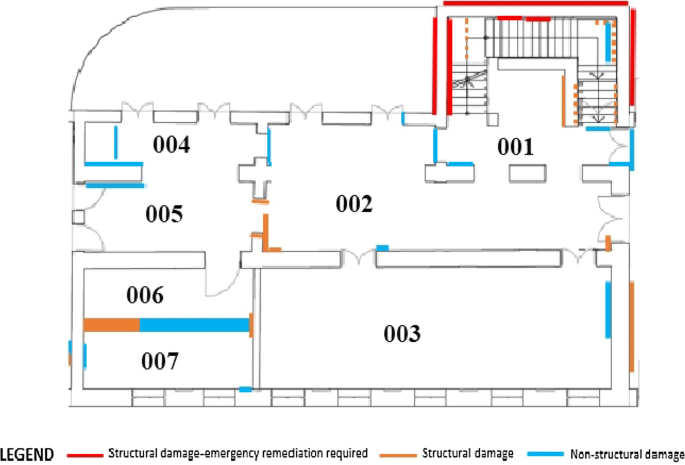
Damage pattern and shear strength-testing locations on the building's ground floor
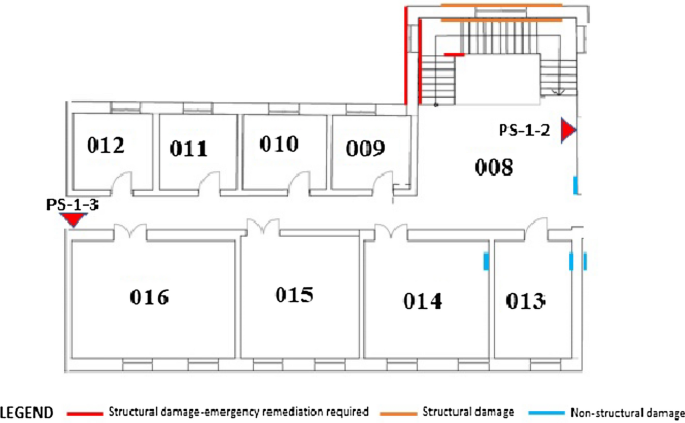
Damage pattern and shear strength-testing locations on the first level of the structure
After a detailed assessment of the structure, the following damages were discovered: cracks in wall hangings, vaults, and ceiling, and separation and local degradation of plaster on the bottom floor. Due to lateral motions during the earthquake, cracks in the barrel vaults are frequently parallel to the supporting joints. The cracks are caused by tensile strains that run perpendicular to the supporting joint. If such fissures develop deeply enough, they can induce hinge formation and, as a result, loss of stability. However, the defects found in the studied structure are superficial and mostly concentrated in the plaster. Minor local damage occurred to the structural elements (walls, columns, and arches). Diagonal fractures on load-bearing walls may be seen in the center core of the structure, where the main stairway is located, and in the eastern part of the building. These fissures can also be noticed on the building's north side. All the floors show visible damage, such as cracks and crumbling plaster on the walls. Minor local damage to the first-floor walls, as well as cracks on the partition walls and ceiling connectors, may be seen.
The east wing's ground and first floors were severely damaged. The cracks that developed spread along the entire wall of the wing's south front. It is unfavorable that the fissures are connected and proceed to the transversely interconnected walls and lintels. The little contribution of a torsional reaction of the structure, where the boundary elements are the most strained and collapse might induce such fractures. In addition, that component of the structure is connected to the adjacent structure. Although this can have a favorable impact in general, in the case of the east wing walls, such a boundary condition might produce extra forces. The walls may fail if they are not well connected to the diaphragms. The wall is not in danger of collapsing because there has been no out-of-plane displacement, but it should be strengthened as soon as possible to prevent further damage. Except for the main staircase, the entire structure is available for use. Depending on the probable future, a static and dynamic analysis of the structure's current condition must be done. Irrespective of how much the entire structure would be repaired, the primary staircase, as well as every other wall with cracks along their length, need to be renovated and repaired. Prior to that, preliminary research on the masonry's characteristics and other essential data for the design and analysis must be performed.
7.3 Numerical modeling
The 3Muri software is used to generate a 3D numerical model of the evaluated building. Because of its computational efficiency and excellent accuracy, the macro-element technique is used (Mouyiannou et al. 2014 ).
Its simulation adaptability (including properties of the different elements, realistic floor stiffnesses, strengthening, and many other features) makes it extremely helpful in a location where the great bulk of building stock is brick. This research was based on relevant case analyses in the 3Muri program (Lamego et al. 2017 ; Malcata et al. 2020 ).
The equivalent-frame technique, which employs non-linear beam elements, is used by the macro-element approach. The three categories of macro-elements are piers, spandrels, and stiff nodes (or non-linear beam elements). The piers and spandrels, which are connected by rigid nodes, are the focal points of all deformation. Figure 26 depicts an analogous frame model constructed from these aforementioned macro-elements.
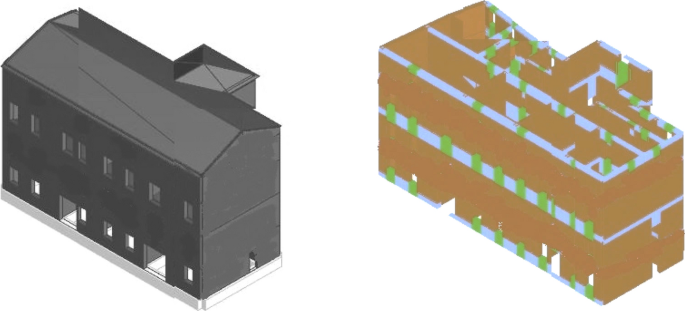
3Muri 3D model and a 3D comparable framework
Non-linear static pushover analysis (Fajfar and Fischinger 1998 ; Cerovecki et al. 2018 ) verifies the limit load ratio employed in numerical solution and provides more comprehensive information on relevant components, failure causes, and the building's overall performance. The pushover study is conducted with constant dynamic loading and monotonically increasing lateral stiffness. Two alternative horizontal load distributions along the building's elevation are investigated in the pushover research. The horizontal load is proportionate to the mass of the building in the first distribution, which also has a high degree of similarity. The horizontal loads are transferred according to the structure's mode pattern in the second distribution first vibration mode form as established by elastic analysis (Figs. 27 and 28 ). Such horizontal forces are imposed in the model at the position of the masses, i.e., at each floor level in the center of the masses. Furthermore, incidental irregularity is carefully considered to account for uncertainty in the determination of the building’s center of mass. For both the x (longitudinal) and y (transversal) directions, 3% of the structure's height perpendicular to the seismic load direction is considered on each side.
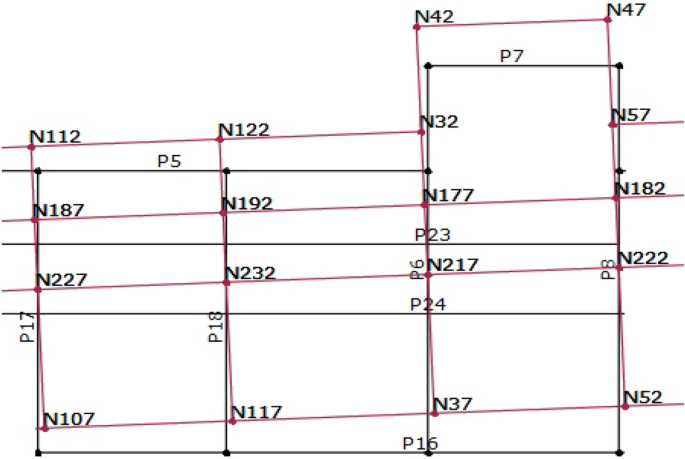
Pushover in the y-direction with a mode shape associated with a period of T = 0.26 s
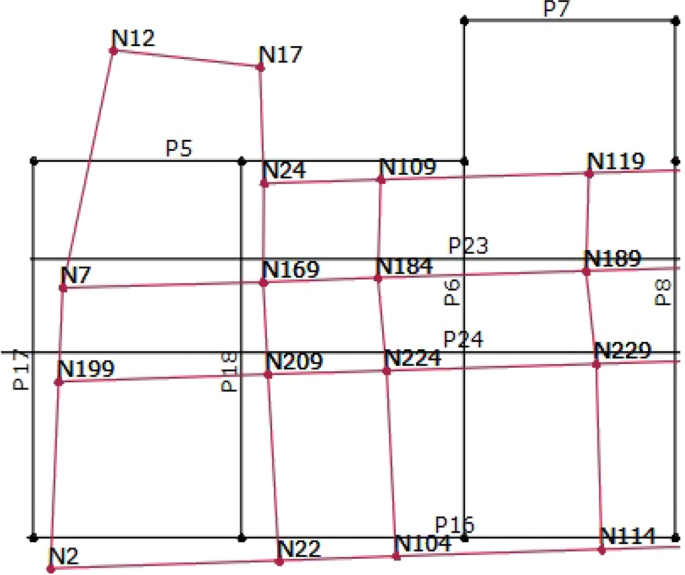
Pushover mode shape in the x-direction, associated with a period T = 0.13 s
In a 3D model, floors are considered as horizontally stiff diaphragms, which is realistic due to the true in-plane rigidity of the horizontal floor elements. In software, rigid diaphragms' in-plane rigidity is limitless, and the mass of the real slab is considered. The roof is removed from the load-bearing structure in the seismic analysis since it has no substantial effect on the structure's reaction and does not contribute to the building's overall resistance. Although it did not meet the criteria, its significance to the model in the form of force was not disregarded.
The numerical model's average values of material parameters (Table 4 ) are based on an analysis of relevant literature (Ghiassi et al. 2019 ; EC06 2021 ) as well as on-site testing. Knowledge level 2 (normal knowledge) can be characterized in terms of experimental in situ testing and extensive study of the structure. The confidence factor was set at 1.2 based on the attained knowledge level.
According to the study in (EC06 2021 ), the building is characterized as normal in height but irregular in floor design, necessitating 3D modeling. The structure is designated as a torsional stiff system. First, static analysis is carried out according to EC06 ( 2021 ) followed by dynamic analysis.
Because seismic resilience is crucial given the consequences of a catastrophe, the pedagogical facility is designated as having relevance class II. As a result, the significance factor is I = 1.2. For two limit states, three PGA values are employed.
The capacity curve represented the ratio of shear force at the structure's base to control node displacement resulting from the seismic analysis. The control node was chosen to be close to the center of mass and is positioned on the building's top story. Figure 29 displays the capacity curves generated from all investigations. Figures 30 and 31 show bi-linearized pushover curves in the x and y axes, respectively. The y-axis shows total base shear in kN, while the x-axis shows control node displacement in mm.
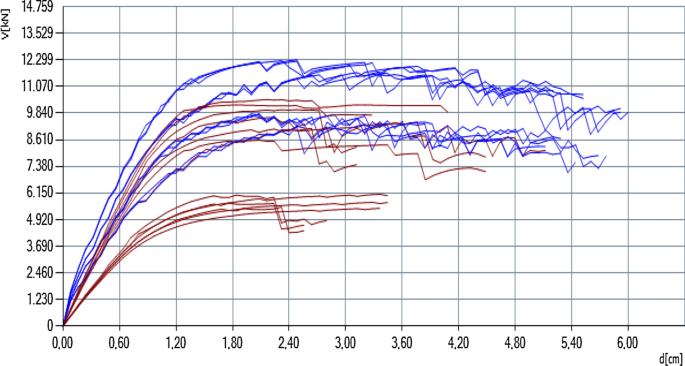
Pushover curves for the x (blue) and y (red) axes
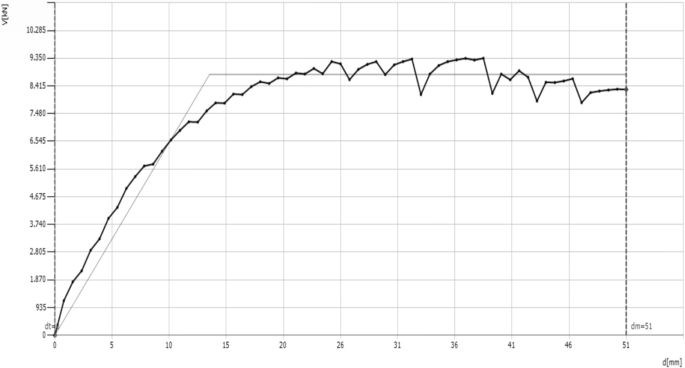
The most relevant pushover curve for the x-direction
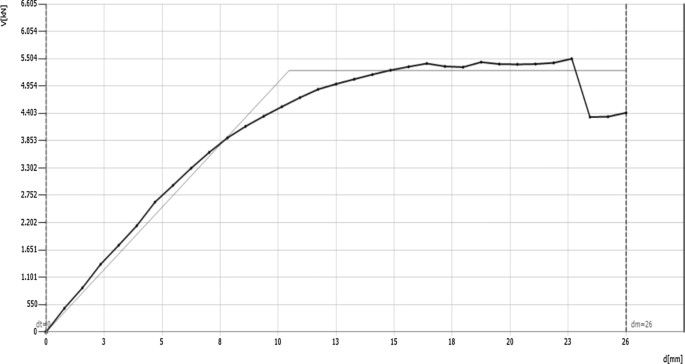
The most relevant pushover curve for the y-direction
Figures 32 and 33 show the damaged condition until the last stage of the pushover curves in the x and y-axis, with yellow indicating shear damage and red indicating bending damage.
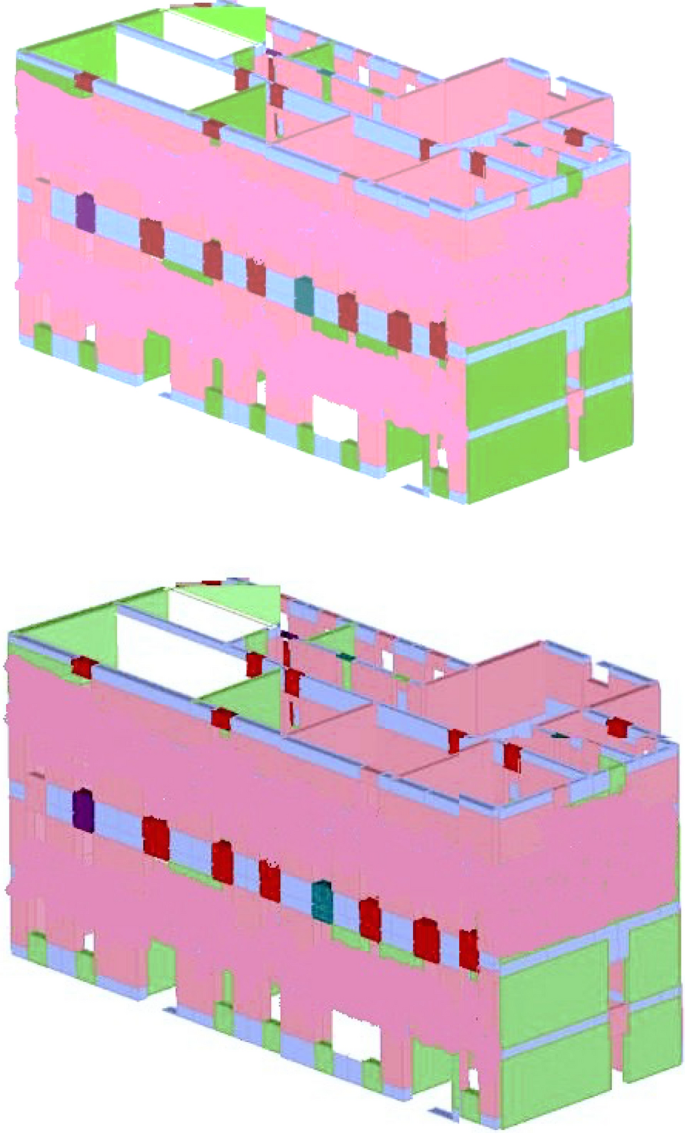
Damage at maximum displacement capacity for x-direction pushover. Yellow: shear damage, red: bending damage
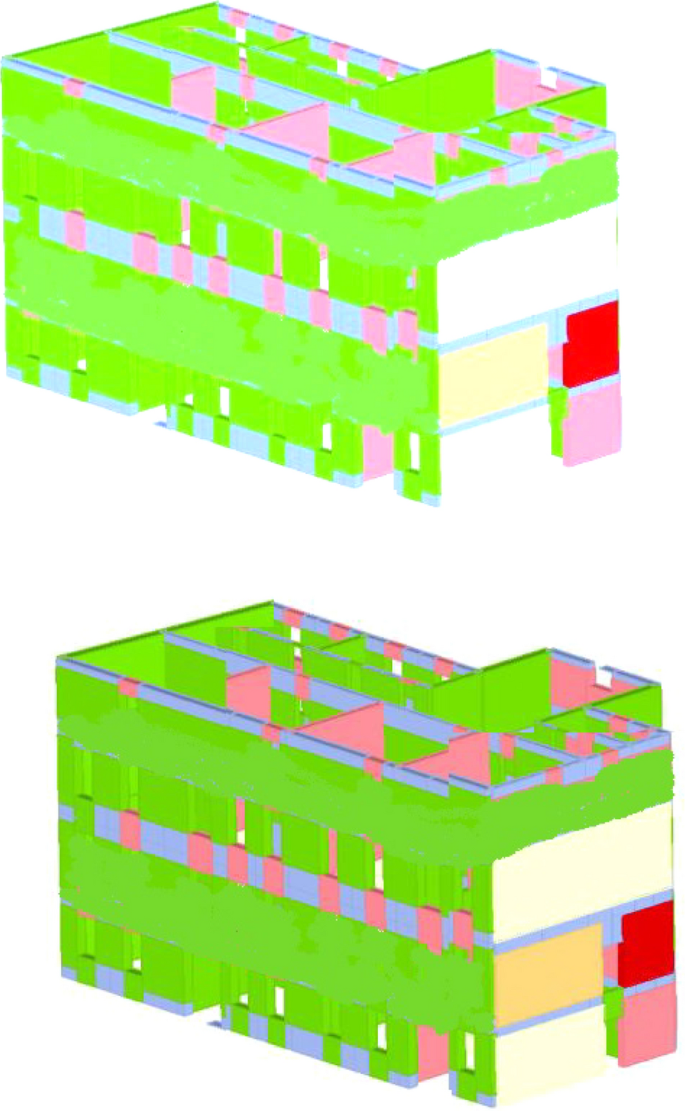
Damage at maximum displacement capacity for y-direction pushover. Yellow: shear damage, red: bending damage
The structure's capacity is assessed after the structure's response, and inspections are carried out in line with the fundamental standards pertaining to the status of extensive damage, as defined by limit states. Table 5 shows the parameters for similar SDOF systems from Figs. 32 and 33 . These characteristics are determined through bilinearization by employing the associated energy concept that is used to calculate target movement.
The ratio α of the building's limit capacity acceleration to the reference peak ground acceleration on type A ground is also presented in Table 6 . For all limit states, the parameter is provided. An issue emerges with historic masonry structures, which are frequently unable to be strengthened to the extent required to meet today's seismic-resistant construction requirements.
A study of the walls out-of-plane bending must then be performed. This explains the earthquake activity that occurs perpendicular to the walls. The investigation is carried out for the limited situation that is on the verge of collapsing. Acceleration for a 475-year return period is used. If a wall's MRd/MEd ratio is greater than or equal to 1.0, it is considered to have passed the out-of-plane bending test. In Fig. 34 , the walls that failed the examination are shown in red.
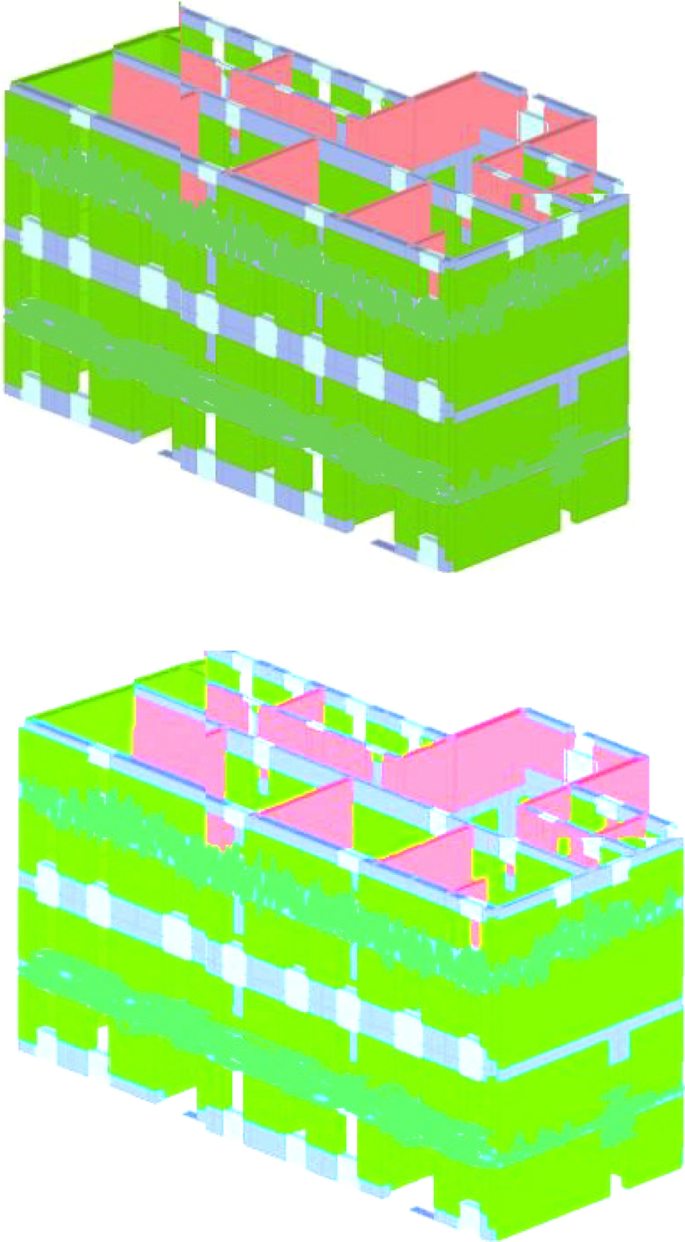
Out-of-plane bending findings. Red: bending damage
In the global study, the 3Muri software also does not take the out-of-plane loss of stability into account. It is believed that appropriate connections are created across walls and diaphragms. Out-of-plane local processes are thus avoided, allowing the global in-plane response of the structure to be studied (Lagomarsino et al. 2013 ). Therefore, the resistance to consolidation is put to the test in a specific program unit; specific elements of a single wall are tested, as well as the interaction of parts of many distinct walls that can generate various local mechanisms when combined. A local mechanism is seen in Fig. 35 , with the mechanical component.
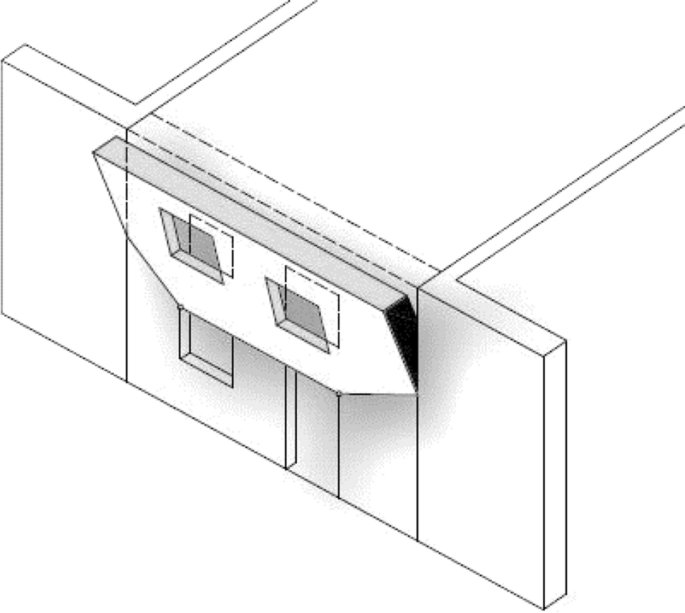
Example of a local mechanism
Local mechanisms are arbitrarily established based on the geometry of the building, common failure mechanisms, and seismic damage. Local mechanisms commonly emerge because of faulty wall and wall-to-floor structural connections. The linear kinematic analysis method is applied. There are three phases to defining a local mechanism. To begin, a kinematic block is a stiff wall element that is sensitive to movement or tilting in relation to another block or the whole of the wall. The initial conditions are then found, and the load is finally calculated. Several of the local progressive collapse of the apparent building, as well as its resistance to the types of failure shown, are listed below (Fig. 36 and Table 7 ). The ratio of the response spectrum of mechanical activation to the response spectrum of earthquake excitation is represented by the α parameter.
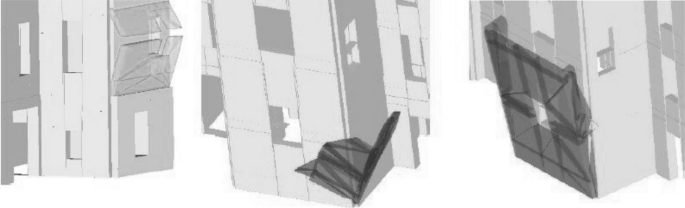
The local mechanisms LM1 (east wing), LM2 (right wing), and LM3 (left wing) are represented in the diagram (central wing)
8 Discussion and conclusions
Based on case studies from recent seismic events in Japan and Italy, (Da Porto et al. 2013 ; Lucibello et al. 2013 ; Formisano 2017 ; Formisano and Marzo 2017 ; Boschi et al. 2018 ; Chieffo and Formisano 2019 ; Malcata et al. 2020 ), the structure under study was assessed to determine its earthquake resilience. The Mila, Algeria’s recent earthquake damaged the structure. The building was not built according to seismic design principles. However, the repair and retrofit improved the structure's condition. Transverse walls are added, and old timber beams are replaced with reinforced concrete flooring. Such stiff diaphragms provide a good connection between all walls and, as a result, improved the seismic behavior of the structure. Therefore, enhancing the rigidity of conventional timber flooring in ancient brick buildings is frequently one of the first seismic retrofitting procedures. On the other hand, a recent study shows that replacing traditional hardwood floors with rigid diaphragms, such as RC flooring, improves energy efficiency. Cracks on the edges of the two materials, or, in the worst-case scenario, disintegration, and collapse of the masonry walls, might be the consequence. This strategy, however, is effective for earthquakes of expected smaller magnitudes, such as those in Mila, and serves to reinforce the existing structure against horizontal actions.
We described the damage to Mila's historic downtown following the earthquake on August 7, 2020. Despite previous calls from the scientific and technical communities, the earthquake demonstrated and verified that awareness, vulnerability mitigation, and readiness are critical to preventing catastrophic seismic consequences and enabling timely action after an earthquake. Although of low magnitude, the Mila earthquake inflicted major damage and economic loss, as well as exposed many weaknesses in the built heritage that people, decision-makers, and the professional and scientific community will have to address for many years to come. The earthquake severely destroyed older masonry structures built before seismic standards were enforced.
The information obtained during the rapid post-earthquake assessment was analyzed and discussed. Damages are graphically shown and illustrated by images. This report examines preliminary data from the database that was enhanced by field engineers. Forms for rapid post-earthquake building evaluation were developed shortly after the 1980 earthquake, and similar forms were used after subsequent earthquakes such as the Tipaza and Zemmouri earthquakes. The findings of the rapid evaluations must be calibrated and reconciled with the extensive reviews provided by the new Act for the rehabilitation of Mila city. In the impacted region, 1044 structures have been identified as severely damaged and will be submitted to further inspections.
3 Muri and an analogous frame approach were used to analyze the existing unconfined masonry structure. Because of its multiple advantages, non-linear static (pushover) seismic analysis is used instead of linear seismic analysis. For the 100, 225, and 475-year return periods, limit state checks were performed. In terms of the structure's existing wall distribution, the results are consistent with the expected behavior. The construction becomes less stiff and has higher displacements in the y direction. Furthermore, the structure's capacity is reduced in the y-direction. There is also some irregularity between the centers of stiffness and mass, which causes torsion to have a minor but unfavorable influence on the overall behavior of the structure. The middle stairwell's walls, as well as the west side of the structure’s cross walls, are crucial elements. Out-of-plane bending failure of walls was also investigated. Using linear kinematics, the causes of failure mode were also examined. The actual damage was compared to the damage calculated using the 3Muri software's non-linear static seismic analysis. From the examination of the structure's behavior during an earthquake, it is evident that strengthening is necessary to improve the structure's seismic performance. An earthquake's damage must be repaired to avoid future damage and threat to the structure's overall strength and stability.
The main results of the investigated area of the city are that the damaged structures are typically older and were built before seismic regulations were implemented. The building materials have degraded over time. Furthermore, the structures are typically under-maintained and, in many cases, designed as seismically deficient. Local (rather than global) in-plane and out-of-plane mechanisms were the most typical failure modes. Significant damage has been found in secondary and/or ornamental building components. Buildings collapsing all at once were extremely infrequent. Aside from concerns with the existing susceptible building stock built prior to seismic regulations, the amount of damage was compounded owing to unlawful conversion of ground levels for commercial activity. There appears to be a widespread lack of understanding and perception of seismic danger in the population, as well as a lack of preventative information dissemination aimed at boosting awareness.
As a result of the several devastating earthquakes that have struck Algeria, the ability to 'build back better’ is much valued. This involves using sustainable resources and producing new ideas (Funari et al. 2020 , 2021 ; Stepinac et al. 2020 ) should be implemented, and energy efficiency should be ensured (Milovanovic and Bagaric 2020 ; Valluzzi et al. 2021 ). Buildings made of masonry can be strengthened using a variety of techniques (Ortega et al. 2017 ; Kouris and Triantafillou 2018 ; Skejic et al. 2020 ). Due to their compatibility and reversibility, materials like FRP (Fiber-Reinforced Polymers) and TRM (Textile-Reinforced Mortars) can be used.
Data availability
All data generated or analyzed during this study are included in this article.
Akkouche K, Hannachi NE, Hamizi M et al. (2019) Knowledge-based system for damage assessment after earthquake: Algerian buildings case. Asian J Civ Eng 20:769–784. https://doi.org/10.1007/s42107-019-00143-z
Article Google Scholar
Allali SA, Abed M, Mebarki A (2018) Post-earthquake assessment of buildings damage using fuzzy logic. Eng Struct 166:117–127. https://doi.org/10.1016/j.engstruct.2018.03.055
Amari K, Abdessemed F, Cheikh Zouaoui M, Uva G (2020) Seismic vulnerability of masonry lighthouses: a study of the bengut lighthouse, Dellys, Boumerdès, Algeria. Buildings 10:247. https://doi.org/10.3390/buildings10120247
ARES (2021) Project first Workshop. Available online: www.grad.hr/ares (Accessed on 15 Feb 2021)
Atalić J, Šavor Novak M, Uroš M (2019) Seismic risk for Croatia: overview of research activities and present assessments with guidelines for the future. Građevinar 71(10):923–947
Google Scholar
Athmania D, Benaissa A, Hammadi A, Bouassida M (2010) Clay and marl formation susceptibility in Mila Province. Algeria Geotech Geol Eng 28(6):805–813. https://doi.org/10.1007/s10706-010-9341-5
Baggio C, Bernardini A, Colozza R, Corazza L, Bella M, Di Pasquale G, Dolce M, Goretti A, Martinelli A, Orsini G et al. (2007) Field manual for post-earthquake damage and safety assessment and short-term countermeasures (AeDES), JRC Sci, Thechnical Reports. 1–100
Bechtoula H, Ousalem H (2005) The 21 May 2003 Zemmouri (Algeria) earthquake: damages and disaster responses. J Adv Concr Technol 3(1):161–174
Belazougui M (2008) Boumerdes Algeria earthquake of May 21, 2003: damage analysis and behavior of beam-column reinforced concrete structures. In: proceedings of the 14th world conference on earthquake engineering, Beijing, Paper 14_01–1006
Benfedda A, Serkhane A, Bouhadad Y, Slimani A, Abbouda M, Bourenane H (2021) The main events of the July-August 2020 Mila (NE Algeria) seismic sequence and the triggered landslides. Arab J Geosci 14:1894. https://doi.org/10.1007/s12517-021-08301-x
Betti M, Bonora V, Galano L, Pellis E, Tucci G, Vignoli A (2021) An integrated geometric and material survey for the conservation of heritage masonry structures. Heritage 4:35
Bialas J, Oommen T, Rebbapragada U, Levin E (2016) Object-based classification of earthquake damage from high-resolution optical imagery using machine learning. J Appl Remote Sens 10(03):036025
Binda L, Saisi A, Tiraboschi C (2000) Investigation procedures for the diagnosis of historic masonries. Constr Build Mater 14(4):199–233. https://doi.org/10.1016/S0950-0618(00)00018-0
Boschi S, Borghini A, Pintucchi B, Bento R, Milani G (2018) Seismic vulnerability of historic masonry buildings: a case study in the center of Lucca. Procedia Struct Integr 11:169–176
Boukri M, Farsi MN, Mebarki A, Belazougui M (2013) Development of an integrated approach for Algerian building seismic damage assessment. Struct Eng Mech 47(4):471–493. https://doi.org/10.12989/SEM.2013.47.4.471
Boulahia O (2022) Identification, characterization and interaction of seismic sources: Implication on sequences of seismic events of the period 2010–2021 in the North-East of Algeria Doctoral thesis at Sétif University. http://dspace.univ-setif.dz:8888/jspui/handle/123456789/3956
Bounemeur N, Benzaid R, Kherrouba H et al. (2022) Landslides in Mila town (northeast Algeria): causes and consequences. Arab J Geosci 15:753. https://doi.org/10.1007/s12517-022-09959-7
Bounif MA, Haessler H, Meghraoui M (1987) The Constantine earthquake of October 27, 1985: surface ruptures and aftershock study. Earth Planet Sci Lett 85:451–460
Casapulla C, Argiento LU, Maione A (2018) Seismic safety assessment of a masonry building according to Italian Guidelines on cultural heritage: simplified mechanical-based approach and pushover analysis. Bull Earthq Eng 16:2809–2837
Cerovecki A, Kraus I, Moric D (2018) N2 building design method. Gradjevinar 70:509–518
Chieffo N, Formisano A (2019) Comparative seismic assessment methods for masonry building aggregates: a case study. Front Built Environ 5:123
Chimouni R, Harbi A, Boughacha MS, Hamidatou M, Kherchouche R, Sebaï A (2018) The 1790 Oran earthquake, a seismic event in times of conflict along the Algerian coast: a critical review from western and local source materials. Seismol Res Lett 89(6):2392–2403. https://doi.org/10.1785/0220180175
Coiffait PE (1992) A post-nappe basin in its structural framework: The example of the Constantine basin (North-East Algeria). Dessertation, University of Nancy
Da Porto F, Munari M, Prota A, Modena C (2013) Analysis and repair of clustered buildings: case study of a block in the historic city center of L’Aquila (Central Italy). Constr Build Mater 38:1221–1237
Dall’Asta A, Leoni G, Meschini A, Petrucci E, Zona A (2019) Integrated approach for seismic vulnerability analysis of historic massive defensive structures. J Cult Herit 35:86–98
De Luca FGED, Woods C, Galasso D, D’Ayala RC (2018) In filled building performance against the evidence of the 2016 EEFIT Central Italy post-earthquake reconnaissance mission: empirical fragilities and comparison with the FAST method. Bull Earthq Eng 16(7):2943–2969. https://doi.org/10.1007/s10518-017-0289-1
Didier M, Baumberger S, Tobler R, Esposito S, Ghosh S, Stojadinovic B (2017) Improving post-earthquake building safety evaluation using the 2015 Gorkha, Nepal, Earthquake rapid visual damage assessment data. Earthq Spectra 33:415–438
Durand DM (1969) Focus on the structure of the Northeast of the BERBERIE. Bull Serv Carte Geol Agerie NS 39:89–131
EMS (1998) Comision Sismologica Europea, Escala Macro Sísmica Europea EMS 98(15)
Endo Y, Pelà L, Roca P (2017) Review of different pushover analysis methods applied to masonry buildings and comparison with nonlinear dynamic analysis. J Earthq Eng 21:1234–1255
Eurocode 6 (2021) Design of masonry structures—Part 1–1: general rules for reinforced and unreinforced masonry structures. Available online: https://www.phd.eng.br/wp-content/uploads/2015/02/en.1996.1.1.2005.pdf
Eurocode 8 (2004a): Design of structures for earthquake resistance—Part 1: general rules, seismic actions and rules for buildings. EN 1998–1: European Committee for Standardization, Brussels. https://www.phd.eng.br/wp-content/uploads/2015/02/en.1998.1.2004a.pdf
Eurocode 8 (2004b) Design of structures for earthquake resistance—Part 3: assessment and retrofitting of buildings. Available online: https://www.phd.eng.br/wp-content/uploads/2014/07/en.1998.3.2005.pdf
Fajfar P, Fischinger M (1998) N2—a method for non-linear seismic analysis of regular buildings. In: proceedings of the 9th world conference in earthquake engineering, Tokyo-Kyoto, Japan, 2–9 August 1998, pp 111–116
Farsi MN, Belazougui M (1992) The Mont Chenoua (Algeria) earthquake of October 29th 1989; damage assessement and distribution", Proceedings of the 10th world conference on earthquake engineering, Madrid, Spain
Formisano A (2017) Theoretical and numerical seismic analysis of masonry building aggregates: case studies in San Pio Delle Camere (L’Aquila, Italy). J Earthq Eng 21:227–245
Formisano A, Marzo A (2017) Simplified and refined methods for seismic vulnerability assessment and retrofitting of an Italian cultural heritage masonry building. Comput Struct 180:13–26
Funari MF, Mehrotra A, Lourenço PB (2021) A tool for the rapid seismic assessment of historic masonry structures based on limit analysis optimisation and rocking dynamics. Appl Sci 11:942
Funari MF, Spadea S, Lonetti P, Fabbrocino F, Luciano R (2020) Visual programming for structural assessment of out-of-plane mechanisms in historic masonry structures. J Build Eng 31:101425
Ghiassi B, Vermelfoort AT, Lourenço PB (2019) Masonry Mechanical Properties. In: Numerical modeling of masonry and historical structures. Woodhead Publishing, pp 239–261
Grillanda N, Valente M, Milani G, Chiozzi A, Tralli A (2020) Advanced numerical strategies for seismic assessment of historical masonry aggregates. Eng Struct 212:110441
Guemache MA, Machane D, Beldjoudi H, Gharbi S, Djadia L, Benahmed S, Ymmel H (2010) On a damaging earthquake-induced landslide in the Algerian Alps: the March 20, 2006 Laâlam landslide (Babors chain, northeast Algeria), triggered by the Kherrata earthquake (Mw = 5 3). Nat Hazards 54(2):273–288. https://doi.org/10.1007/s11069-009-9467-z
Halla N, Hamidatou M, Hamai L, Atmane L, Yelles chaouche A (2022) Earthquake induced landslide in Milla region: the August 07, 2020 (El-Kherba, Grarem-Gouga and Azzeba) landslides Northeast Algeria, triggered by the Mila earthquake (Mw = 5). In process for publication, Naturals hazards journal
Hamidatou M, Mohammedi Y, Hallal N, Yelles-Chaouche A, Lebdioui S, Thallak I, Stromeyer D, Khemici O (2021) Reply to the comment on the paper “seismic hazard analysis of surface level, using topographic condition in Northeast of Algeria” by Mohamed Hamdache and José A. Pelàez. Pure Appl Geophys 178:305–312. https://doi.org/10.1007/s00024-020-02644-4
Hamidatou M, Mohammedi Y, Yelles-Chaouche A, Thallak I, Stromeyer D, Lebdioui S, Cotton F, Hallal N, Khemici O (2019) Seismic hazard analysis of surface level, using topographic condition in the Northeast of Algeria. Pure Appl Geophys 178(3):823–846. https://doi.org/10.1007/s00024-019-023104
Hamidatou M, Sbartai B (2016) Deterministic assessment of seismic risk in Constantine city, Northeast Algeria. Nat Hazards 86(2):441–464. https://doi.org/10.1007/s11069-016-2693-2
Hamidatou M, Sbartai B (2017) Probabilistic seismic hazard assessment in the Constantine region, Northeast of Algeria. Arab J Geosci 10(6):1–20. https://doi.org/10.1007/s12517017-2876-5
Harbi A, Maouche S, Ousadou F, Rouchiche Y, Yelles-Chaouche A, Merahi M, Heddar A, Nouar O, Kherroubi A, Beldjoudi H, Ayadi A, Benouar D (2007) Macroseismic study of the Zemmouri earthquake of 21 May 2003 (Mw 6.8, Algeria). Earthq Spectra 23(2):315–332
Harbi A, Maouche S, Vaccari F, Aoudia A, Oussadou F, Panza GF, Benouar D (2007b) Seismicity, seismic input and site effects in the Sahel-Algiers Region (North Algeria). Soil Dyn Earth Eng 27(5):427–447
Harbi A, Meghraoui M, Maouche S (2011) The Djidjelli (Algeria) earthquakes of 21 and 22 August 1856 (I0 VIII, IX) and related tsunami effects Revisited. J Seismol 15:105–129. https://doi.org/10.1007/s10950-010-9212-9
Hellel M, Chatelain JL, Guillier B, Machane D, Ben Salem R, Oubaiche E, Haddoum H (2010) Heavier damages without site effects and site effects with lighter damages: Boumerdes City (Algeria) after the May 2003 earthquake. Seismol Res Lett 81(1):37–43. https://doi.org/10.1785/gssrl.81.1.37
Hichem N, Ahmed M, Mohamed A (2019) Post-quake structural damage evaluation by neural networks: theory and calibration. Eur J Environ Civ Eng 23:710–727. https://doi.org/10.1080/19648189.2017.1304277
Indirli MS, Kouris LA, Formisano L, Borg RP, Mazzolani FM (2013) Seismic damage assessment of unreinforced masonry structures after the abruzzo 2009 earthquake: the case study of the historical centers of L’Aquila and castelvecchio subequo. Int J Architect Herit 7(5):536–578. https://doi.org/10.1080/15583058.2011.654050
Karimzadeh S, Askan A, Erberik MA, Yakut A (2018) Seismic damage assessment based on regional synthetic ground motion dataset: a case study for Erzincan, Turkey. Nat Hazards 92(3):1371–1397
Karimzadeh S, Kadas K, Askan A, Erberik MA, Yakut A (2020) Derivation of analytical fragility curves using SDOF models of masonry structures in Erzincan (Turkey). Earthq Struct 18(2):249–261
Kim T, Song J, Kwon OS (2020) Pre- and post-earthquake regional loss assessment using deep learning. Earthq Eng Struct Dyn 49:657–678
Kouris LAS, Triantafillou TC (2018) State-of-the-art on strengthening of masonry structures with textile reinforced mortar (TRM). Constr Build Mater 188:1221–1233
Lagomarsino S, Penna A, Galasco A, Cattari S (2013) TREMURI program: an equivalent frame model for the nonlinear seismic analysis of masonry buildings. Eng Struct 56:1787–1799
Lamego P, Lourenço PB, Sousa ML, Marques R (2017) Seismic vulnerability and risk analysis of the old building stock at urban scale: application to a neighbourhood in Lisbon. Bull Earthq Eng 15:2901–2937
Laouami N, Slimani A, Larbes S (2018) Ground motion prediction equations for Algeria and surrounding region using site classification based H/V spectral ratio. Bull Earthq Eng 16(7):2653–2684. https://doi.org/10.1007/s10518-018-0310-3
Laouami N, Slimani N, Bouhadad Y, Chatelain JL, Nour A (2006) Evidence for fault-related directionality and localized site effects from strong motion recordings of the 2003 Boumerdes (Algeria) earthquake: consequences on damage distribution and the Algerian Seismic Code. Soil Dyn Earth Eng 26:991–1003. https://doi.org/10.1016/j.soildyn.2006.03.006
Lourenco P, Karanikoloudis G (2019) Seismic behavior and assessment of masonry heritage structures. Needs in engineering judgement and education. RILEM Tech Lett 3:114–120
Lucibello G, Brandonisio G, Mele E, De Luca A (2013) Seismic damage and performance of Palazzo Centi after L’Aquila earthquake: a paradigmatic case study of effectiveness of mechanical steel ties. Eng Fail Anal 34:407–430
Malcata M, Ponte M, Tiberti S, Bento R, Milani G (2020) Failure analysis of a Portuguese cultural heritage masterpiece: Bonet building in Sintra. Eng Fail Anal 115:104636
Marmi R, Kacimi M, Boularak M (2008) Landslides in the Mila region (North-Eastern Algeria): impact on infrastructure. Revista De Geomorphologie 10:51–56
Marshall JD, Jaiswal K, Gould N, Turner F, Lizundia B, Barnes JC (2013) Post-earthquake building safety inspection: lessons from the canterbury, New Zealand, earthquakes. Earthq Spectra 29:1091–1107
Medhat NI, Yamamoto M-Y, Tolomei C, Harbi A, Maouche S (2022) Multi-temporal InSAR analysis to monitor landslides using the small baseline subset (SBAS) approach in the Mila Basin, Algeria, Terra Nova published by John Wiley & Sons Ltd. https://doi.org/10.1111/ter.12591
Mehani Y, Bechtoula H, Kibboua A, Naili M (2013) Assessment of seismic fragility curves for existing RC buildings in Algiers after the 2003 Boumerdes earthquake. Struct Eng Mech 46(6):791–808. https://doi.org/10.12989/SEM.2013.46.6.791
Merghadi A, Abderrahmane B, Tien Bui D (2018) Landslide susceptibility assessment at Mila Basin (Algeria): a comparative assessment of prediction capability of advanced machine learning methods. ISPRS Int J Geo Inf 7(7):268. https://doi.org/10.3390/ijgi7070268
Meslem A, Yamazaki F, Maruyama Y, Benouar D, Kibboua A, Mehani Y (2012) The effects of building characteristics and site conditions on the damage distribution in Boumerdès after the 2003 Algeria earthquake. Earthq Spectra 28(1):185–216. https://doi.org/10.1193/1.3675581
Milovanovic B, Bagaric M (2020) How to achieve nearly zero-energy buildings standard. Gradjevinar 72:703–720
Mouyiannou A, Rota M, Penna A, Magenes M (2014) Identification of suitable limit states from nonlinear dynamic analyses of masonry structures. J Earthq Eng 18:231–263
Naito S, Tomozawa H, Mori Y, Nagata T, Monma N, Nakamura H, Fujiwara H, Shoji G (2020) Building-damage detection method based on machine learning utilizing aerial photographs of the Kumamoto earthquake. Earthq Spectra 36:1166–1187
Ortega J, Vasconcelos G, Rodrigues H, Correia M (2018) Assessment of the influence of horizontal diaphragms on the seismic performance of vernacular buildings. Bull Earthq Eng 16:3871–3904
Ortega J, Vasconcelos G, Rodrigues H, Correia M, Ferreira TM, Vicente R (2019) Use of post-earthquake damage data to calibrate, validate and compare two seismic vulnerability assessment methods for vernacular architecture. Int J Disaster Risk Reduct 39:101242
Ortega J, Vasconcelos G, Rodrigues H, Correia M, Lourenço PB (2017) Traditional earthquake resistant techniques for vernacular architecture and local seismic cultures: a literature review. J Cult Herit 27:181–196. https://doi.org/10.1016/j.culher.02.015
Ousadou F, Dorbath L, Dorbath C, Bounif MA, Benhallou H (2012) The Constantine (Algeria) seismic sequence of 27 October 1985: a new rupture model from aftershock relocation, focal mechanisms, and stress tensors. J Seismol 17(2):207–222
Palazzi NC, Barrientos M, Sandoval C de la Llera JC (2022) Seismic Vulnerability Assessment of the Yungay's Historic Urban Center in Santiago, Chile. J Earthq Eng 1–28
Penna AP, Morandi M, Rota CF, Manzini F, Da Porto G (2014) Magenes, Performance of masonry buildings during the Emilia 2012 earthquake. Bull Earthq Eng 12(5):2255–2273. https://doi.org/10.1007/s10518-013-9496-6
Raoult JF (1974) Geology of the Center of the numidic chain (North Constantinois, Algeria). Thesis Paris Mem Soc Geol 121: 63
Remki M, Benouar D (2014) Damage potential and vulnerability functions of strategic buildings in the city of Algiers. KSCE J Civ Eng 18:1726–1734. https://doi.org/10.1007/s12205-014-0184-0
Rodríguez AS, Rodríguez BR, Rodríguez MS, Sánchez PA (2019) 9-Laser scanning and its applications to damage detection and monitoring in masonry structures. In: Ghiassi B, Lourenço PB (eds) Woodhead Publishing Series in Civil and Structural Engineering, Long-term Performance and Durability of Masonry Structures, Woodhead Publishing, pp 265–285
RPA (2003) Algerian Earthquake Rules 1999. Version 2003. National Center of Applied Research in Earthquake Engineering (CGS)
Semmane F, Abacha I, Yelles-Chaouche AK et al. (2012) The earthquake swarm of December 2007 in the Mila region of northeastern Algeria. Nat Hazards 64:1855–1871. https://doi.org/10.1007/s11069-012-0338-7
Skejic D, Lukacevic I, Curkovic I, Cudina I (2020) Application of steel in refurbishment of earthquake-prone buildings. Gradjevinar 72:955–966
Smail T, Abed M, Mebarki A, Lazecky M (2022) Earthquake-induced landslide monitoring and survey by means of InSAR. Nat Hazards Earth Syst Sci 22:1609–1625. https://doi.org/10.5194/nhess-22-1609-2022
Stepinac M, Gašparovic M (2020) A review of emerging technologies for an assessment of safety and seismic vulnerability and damage detection of existing masonry structures. Appl Sci 10:5060
Stepinac M, Kisicek T, Renic T, Hafner I, Bedon C (2020) Methods for the assessment of critical properties in existing masonry structures under seismic loads-The ARES project. Appl Sci 10(5):1576. https://doi.org/10.3390/app10051576
Stepinac M, Rajcic V, Barbalic J (2017) Inspection and condition assessment of existing timber structures. Gradjevinar 69:861–873
Tebbouche MY, Ait Benamar D, Hassan HM et al. (2022) Characterization of El Kherba landslide triggered by the August 07, 2020, Mw = 4.9 Mila earthquake (Algeria) ambient noise analysis. Environ Earth Sci 81:46. https://doi.org/10.1007/s12665-022-10172-8
Uroš M, Šavor Novak M, Atalc J, Sigmund Z, Banicek M, Demšic M, Hak S (2020) Post-earthquake damage assessment of buildings–procedure for conducting building inspections. Gradjevinar 72:1089–1115
Valente M, Milani G (2019) Damage assessment and collapse investigation of three historical masonry palaces under seismic actions. Eng Fail Anal 98:10–37
Valluzzi MR, Salvalaggio M, Croatto G, Dorigatti G, Turrini U (2021) Nested buildings: an innovative strategy for the integrated seismic and energy retrofit of existing masonry buildings with CLT panels. Sustainability 13:1188
Vila JM (1980) Geological map at 1/50.000. Dissertation, University of Paris VI
Vlachakis G, Vlachaki E, Lourenço PB (2020) Learning from failure: damage and failure of masonry structures, after the 2017 lesvos earthquake (Greece). Eng Fail Anal 117:104803. https://doi.org/10.1016/j.engfailanal
Yavari S, Chang SE, Elwood KJ (2010) Modeling post-earthquake functionality of regional health care facilities. Earthq Spectra 26:869–892
Zhang Y, Burton HV, Sun H, Shokrabadi M (2018) A machine-learning framework for assessing post-earthquake structural safety. Struct Saf 72:1–16
Download references
This paper did not receive any funding.
Author information
Authors and affiliations.
Research Center in Astronomy, Astrophysics and Geophysics, BP 63, 16340, Bouzaréah, Algiers, Algeria
Hamidatou Mouloud & Hallal Nassim
The Engineering Mechanics Institute, American Society of Civil Engineers, Reston, USA
Amar Chaker
Faculty of Technology, University of August 20, 1955-Skikda, P. O. Box 26, 21000, Skikda, Algeria
Saad Lebdioui
Civil Engineering Department, University of Aveiro, Campus Universitário de Santiago, RISCO, 3810-193, Aveiro, Portugal
Hugo Rodrigues
Dipartiment tal-Ġeoxjenza, Fakultà tax-Xjenza, L-Università ta’ Malta, Msida, Malta
Matthew R. Agius
You can also search for this author in PubMed Google Scholar
Corresponding author
Correspondence to Hamidatou Mouloud .
Ethics declarations
Conflict of interest.
The authors declare no conflict of interest. The funders had no role in the design of the study; in the collection, analyses, or interpretation of data; in the writing of the manuscript, or in the decision to publish the results.
Additional information
Publisher's note.
Springer Nature remains neutral with regard to jurisdictional claims in published maps and institutional affiliations.
Rights and permissions
Springer Nature or its licensor (e.g. a society or other partner) holds exclusive rights to this article under a publishing agreement with the author(s) or other rightsholder(s); author self-archiving of the accepted manuscript version of this article is solely governed by the terms of such publishing agreement and applicable law.
Reprints and permissions
About this article
Mouloud, H., Chaker, A., Nassim, H. et al. Post-earthquake damage classification and assessment: case study of the residential buildings after the M w = 5 earthquake in Mila city, Northeast Algeria on August 7, 2020. Bull Earthquake Eng 21 , 849–891 (2023). https://doi.org/10.1007/s10518-022-01568-9
Download citation
Received : 28 June 2022
Accepted : 09 November 2022
Published : 21 November 2022
Issue Date : January 2023
DOI : https://doi.org/10.1007/s10518-022-01568-9
Share this article
Anyone you share the following link with will be able to read this content:
Sorry, a shareable link is not currently available for this article.
Provided by the Springer Nature SharedIt content-sharing initiative
- Vulnerability
- Residential building
- Classification
- Post-earthquake
- Find a journal
- Publish with us
- Track your research
The Parkfield, California, Earthquake Experiment
September 28, 2004— m 6.0 earthquake captured.
The Parkfield Experiment is a comprehensive, long-term earthquake research project on the San Andreas fault. Led by the USGS and the State of California, the experiment's purpose is to better understand the physics of earthquakes - what actually happens on the fault and in the surrounding region before, during and after an earthquake. Ultimately, scientists hope to better understand the earthquake process and, if possible, to provide a scientific basis for earthquake prediction. Since its inception in 1985, the experiment has involved more than 100 researchers at the USGS and collaborating universities and government laboratories. Their coordinated efforts have led to a dense network of instruments poised to "capture" the anticipated earthquake and reveal the earthquake process in unprecedented detail.
Moderate-size earthquakes of about magnitude 6 have occurred on the Parkfield section of the San Andreas fault at fairly regular intervals - in 1857, 1881, 1901, 1922, 1934, and 1966. The first, in 1857, was a foreshock to the great Fort Tejon earthquake which ruptured the fault from Parkfield to the southeast for over 180 miles. Available data suggest that all six moderate-sized Parkfield earthquakes may have been "characteristic" in the sense that they all ruptured the same area on the fault. If such characteristic ruptures occur regularly, then the next quake would have been due before 1993.
These pages describe the scientific background for the experiment, including the tectonic setting at Parkfield, the historical earthquake activity on this section of the San Andreas fault, the monitoring and data collecting activities currently being carried out, and plans for future research. Data are available to view in real-time and download.
Scientific Advances
While the greatest scientific payoff is expected when the earthquake occurs, our understanding of the earthquake process has already been advanced through research results from Parkfield. Some of the highlights are described.
Real-time data from instrumentation networks running at Parkfield are available for viewing and downloading.
Parkfield Earthquake Shake Table Exhibit
The Art-Science of Earthquakes by D.V. Rogers November 23, 2009 ( video )
The exhibit was a geologically interactive, seismic machine earthwork temporarily installed in Parkfield in 2008. Rogers presented the history, conceptual premise, documentation of the work, and also put forward the idea of how early 21st century cultural practice could be used to encourage earthquake awareness and preparedness.
Pictures and interactive, 360-degree panorama .
Lessons From the Best-Recorded Quake in History
USGS Public Lecture by Andy Michael October 26, 2006 ( video )
New data from the 2004 Parkfield earthquake provide important lessons about earthquake processes, prediction, and the hazards assessments that underlie building codes and mitigation policies.
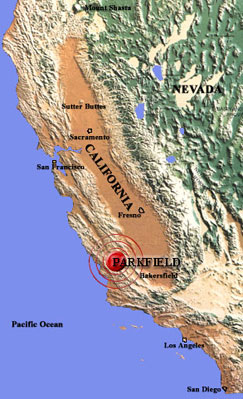
Research Scientist: John Langbein , Earthquake Science Center.
Things you buy through our links may earn Vox Media a commission.
Earthquake and Aftershock Shake New York City: Live Updates
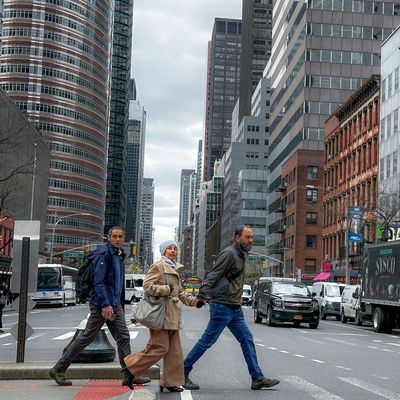
A 4.8 magnitude earthquake shook New Jersey and New York City on Friday morning, followed by a 4.0 magnitude aftershock in the evening — surprising and confusing area residents not used to seismic phenomena. Below are the latest updates and everything we know about the quake, aftershock, and aftermath.
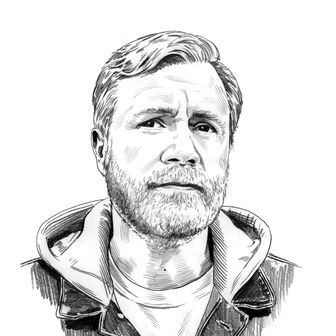
Only in New York
Our emergency alerts may lag, but no other city can out-hustle this one:
Could there be additional powerful aftershocks?
Earthquake Insights’ Dr. Judith Hubbard and Dr. Kyle Bradley explained the possible aftershocks in their detailed analysis of the East Coast quake:
As with all earthquakes, this event will trigger some aftershocks. Aftershocks are simply earthquakes that occur after other earthquakes, and are therefore thought to be related to stress changes caused by the first event. Most aftershocks will be small - many of them too small to feel. As of this post, six events smaller than magnitude 2.5 have already been detected. Based on records from past events, the USGS has developed an aftershock forecast. This forecast shows that aftershocks of at least magnitude 3 are likely; magnitude 4+ events are also possible (30% chance over the next year). It is possible, but not likely, that this earthquake could trigger an event above magnitude 5. If that happened, that event would renamed the mainshock, and the M4.8 would be described as a foreshock.
And per USGS research structural engineer Kishor S. Jaiswal, in a comment to the New York Times :
The aftershocks will likely “continue for several days and even a week,” he said Friday evening. There is also a small chance that an earthquake of similar or even larger magnitude could occur during such a sequence, he said.
A sizable evening aftershock!
The aftershock, registering a preliminary 4.0 magnitude , happened at 5:59 p.m. with an epicenter a little over 4 miles southwest of Gladstone, New Jersey. It was not the first aftershock, but the first one widely felt in the area since the more powerful first quake in the series on Friday morning.
Remember the pets
Animals don’t love earthquakes .
A few homes suffered structural damage in Newark
But there were no injuries. WABC reports :
Three multifamily homes have been evacuated by the city of Newark following Friday’s earthquake. There’s some roof damage. One house apparently shifted during the earthquake and is now leaning on the house next door. The homes are located near the intersection of 7th Avenue and 3rd Street. Shortly after the earthquake at 10:23 a.m. on Friday , the city received a 911 call to report structural damage. When firefighters arrived, they evacuated all three buildings. Ten families are displaced — a total of 25 people, including 3 children.
In case you were wondering how well New York’s older buildings would fair against a stronger quake
Aaron Short spoke with a structural engineer Troy Morgan , who says we shouldn’t bet on the safety of brick:
Which city buildings are the least equipped for an earthquake? Generally, unreinforced masonry or brick buildings because they’re heavy and they lack steel reinforcement. Things that are heavy experience more force, and if they don’t have enough steel reinforcement to resist those forces, they can be susceptible to damage. There are a lot of brick buildings in New York, New Jersey, and Connecticut, and those are buildings you’d expect to see cracking to develop or damage to their foundations. You can get shaking if there’s a large enough quake, and you can get liquefaction, where the soil temporarily liquifies, and you can get settlement that results from that.
Read the rest of the interview here .
A 1995 New York cover story , which we’ve republished for the first time today, examined how well the city would hold up against an earthquake. It begins:
More than 80 people were interviewed for this piece. They came from many different disciplines. They had wildly competitive agendas. But there was one thing they all agreed on: It’s coming. They disagreed on how big, how devastating, how soon. But this, no one denies — we’re going to see an earthquake. In New York City. And we’re not ready for it.
Read the rest of that story here .
Sorry earth science conspiracy theorists, the earthquake is probably not linked to the total eclipse
Experts, if you believe in experts, tell the New York Times there’s scant evidence the two rare events could be related:
Earthquakes happen along fault lines, or cracks between two blocks of rock on Earth’s crust. Tides stretch and squish the land on Earth just as they contribute to waves in the ocean, and those tidal forces grow as the sun, moon and Earth begin to align — a configuration that sometimes creates a solar eclipse. One theory is that this may introduce additional stress along Earth’s fault lines. “We do know that the relative position of the Earth and the moon and the sun does exert tidal forces,” said William Frank, a geophysicist at the Massachusetts Institute of Technology. “And we know that changes the stress that can be on a fault that can host an earthquake.” But the results of several studies of the relationship between earthquakes and tides are inconclusive, according to Seth Stein, a geophysicist at Northwestern University. “If there’s any effect, it would be incredibly weak,” he said.
But the Florida woman who believes in Jewish space lasers has her own theory
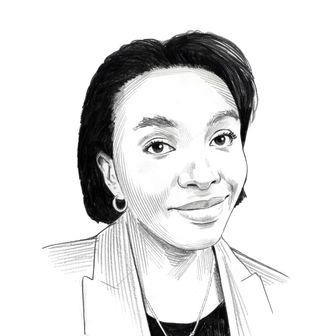
Good thing this wasn’t the big one
I just wrote about the flat-footed response from city and state officials in the immediate aftermath of the quake:
An emergency alert from the city didn’t hit phones for a full half hour after the shaking stopped. A second alert followed about 45 minutes later. An alert from the state would go out nearly a full hour after the quake had subsided, though Governor Kathy Hochul issued a statement shortly after the tremors. When asked about the gap in between when the earthquake was registered to when city residents were alerted, the city’s emergency manager Zach Iscol said the time was actually fast by their standards.
The East Coast earthquake’s music video
There was an aftershock, but it seems to have gone unfelt.
A 2.0 magnitude aftershock was detected near Bedminster, New Jersey, at 12:20 p.m., according to the USGS. While some have reported it “rocked” Donald Trump’s Bedminster golf club, there’s no evidence anybody even felt the second, much smaller tremor.
Still no reports of significant damage
Per Gothamist :
New York City Emergency Management said it hadn’t received reports of any damage to infrastructure shortly after the tremor shook the five boroughs and surrounding area. City infrastructure and services continued to operate following the rare quake. New York City’s public schools had no reports of damage as of about 11 a..m, according to school system spokesperson Nathaniel Styer . The Port Authority of New York and New Jersey reopened the Holland Tunnel following a short delay at about 11:15 a.m. In New Jersey, the state’s Board of Public Utilities said gas and electric infrastructure appeared to be undamaged. “No impacts or damage to utility infrastructure or the grid resulting from the earthquake have been reported at this time,” the board wrote on X just before noon. Inspectors, architects and construction site managers who spoke with Gothamist on Friday morning said they had not received reports of damages or disruption.
What’s it’s like to get a vasectomy during an earthquake
Wired interviews Justin Allen , who had the misfortune of being in a very delicate situation during Friday morning’s tremor:
At what stage of the procedure did this happen? We were probably almost at the midway point. Essentially, the procedure started around 10:10 [am ET] and it was 10:24 or 10:25 that the earthquake hit. Could you, uh, paint the picture for me of what it was like on that table? I’m laying there. He’s in the middle of whatever he needs to do down there and the whole building started shaking. I wasn’t sure what was happening. It definitely felt like an earthquake, but we don’t normally have those. I didn’t know if there was a train nearby or something that would cause the building to shake. And then the doctor was like, “Oh my, God. That’s an earthquake.” I thought he was messing with me. I thought it was just him trying to be funny. But as this was happening, the desk staff outside the room started screaming about an earthquake and I was like “Oh, wow this is really happening.” And the doctor puts the tools down and asks, “How long does an earthquake normally last?” and the nurse said, “I think about a minute or two.” So we stopped and waited, and he resumed as soon as it was done. So he stopped right as the shaking happened? I think so. He was toward the end of whatever step he was doing right then and there. But he did set the tools down for a moment to recalibrate.
How the earthquake looked at the U.N.
Seems like the shaking lasted about 40 seconds:
Mayor Adams says no indication of infrastructure damage or injuries
Mayor Eric Adams and other city officials held a briefing shortly after noon following the earthquake that unsettled the metro area. He said that he’s been in touch with both the White House and Governor Hochul’s office but that the quake appears to have had minimal impact on the city.
“At this point, we do not have any reports of major impacts to our infrastructure or injuries, but, of course, we’re still assessing the situation and will continue to update the public,” he said.
NYC Emergency Management Commissioner Zach Iscol said that the likelihood of aftershocks is “low” but advised residents to be attentive in the coming days. “We do remain vigilant and we ask all New Yorkers to remain vigilant as well,” he said.
Hochul: No major damage reported yet
In a briefing just before noon, Governor Kathy Hochul told reporters that the state hadn’t felt a quake of this magnitude since 2011, when the effects of a 5.8 earthquake that struck Virginia could be felt as far away as Buffalo. “This is one of the largest earthquakes on the East Coast to occur in the last century,” she said.
The governor announced that structural and engineering teams are surveying bridges and roads as well as transmission lines and dams for any impact from the earthquake, but so far nothing has been reported. Hochul also advised residents to inspect their own properties for any damage and to stay alert in case of any aftershocks.
“If there is an after effect, please stay away from buildings, especially our high-rises. If you hear a shifting or any noises, unusual noises, leave your home. Go outside. You’re safer there than in a building that could be crumbling around you,” she said.
Hochul noted that she was meeting with her senior team about the ongoing budget negotiations when the earthquake hit. “Getting a budget done that includes a once in a lifetime housing package may be the only seismic event we expected this week. So this was rather unanticipated,” she said.
Seriously though, are the End Times nigh?
Our official Rapture correspondent, Sarah Jones, asks :
We had floods earlier this week. A solar eclipse is imminent, as is a plague of locusts. Eric Adams is the mayor. It’s all very troubling! And so I return to the Scriptures for guidance, as if the earth shook the atheism right out of me. The Book of Revelation mentions earthquakes quite a lot. For example, in chapter eight: “And the angel took the censer, and filled it with fire of the altar, and cast it into the earth: and there were voices, and thunderings, and lightnings, and an earthquake.” And later, in chapter 16: “And there were voices, and thunders, and lightnings; and there was a great earthquake, such as was not since men were upon the earth, so mighty an earthquake, and so great.”
Read the rest of her response here .
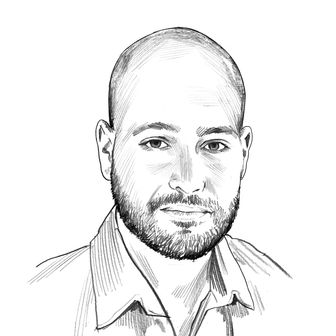
Landing at JFK shortly after the quake was a vibe
There was quite a scene as my plane touched down at JFK and every passenger’s phone sounds out loud with two separate emergency alerts at once. And it’s not even a Boeing plane!
Eric Adams drops some bad advice about standing in doorways
Here’s the outdated guidance the mayor tweeted out about aftershocks:
But the federal government specifically recommends not going near doorways:
If you are inside, stay and do not run outside and avoid doorways.
As a USGS scientist explained to the New York Times :
Experts used to advise people to stand under a doorway during an earthquake, but they don’t do that anymore, Dr. [Robert-Michael de Groot] said. “There was a time when the doorway was structurally more stable, stronger than the rest of other parts of the house,” he said. “That really isn’t the case anymore.”
What was up with that super-late emergency alert?
An emergency alert went out to phones in the NYC metro area, warning residents to remain indoors and to contact 911 if injured. However, the notification didn’t go out until around 11:02 a.m., a full 39 minutes after the quake was first felt. Ahead of the alert, a City Hall spokesman told social media for “awareness” that the city’s emergency-management department would be sending the message. The administration has previously faced criticism for what was seen as a slow response to crises such as the flooding in the city in 2023. A second anticlimactic alert went out at around 11:46 a.m., warning residents that aftershocks “may be felt” but that they can “continue usual activities.”
Airports, Amtrak slow things down in response
Arrivals into Newark, JFK, and La Guardia airports were held because of the quake, the FAA said, according to the New York Times , with 45-minute delays expected. Amtrak is limiting speed until it can finish inspecting the tracks:
Will we feel aftershocks?
It’s not yet clear whether New York City might experience aftershocks, though local officials are warning about the possibility. According to the United States Geological Survey , aftershocks, or the smaller quakes that follow the main earthquake, tend to follow shallow quakes rather than quakes that are more than 30 kilometers deep.
Here’s how you should have reacted
When you feel a strong earthquake, the current advice is to drop down to the ground, cover your heads and neck with your arms, and to take cover under a sturdy object like a table or desk and hold on tight.
An ultrarare strong quake for Jersey
The damage — and what people saw, felt, and joked about.
So far, damage appears to be minimal, with New Yorkers more frustrated with inconveniences caused by the unusual event:
So far, people seem focused on making jokes about the rare and (thankfully minor) seismic shock — and particularly jokes at the expense of the mayor:
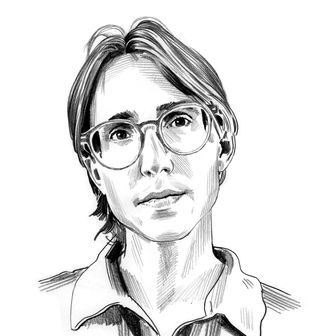
How New York authorities responded
Governor Kathy Hochul tweeted that the state will be “assessing impacts and any damage that may have occurred.” Mayor Eric Adams was being briefed on the matter, according to his press office. He will hold a press conference at 12 p.m.
The earthquake’s magnitude and scale
According to the United States Geological Surve y, the earthquake’s epicenter was near Lebanon, New Jersey, about 50 miles west of New York City. It registered at a 4.8 on the Richter scale and was felt as far away as Philadelphia and Boston. It appears to be the largest earthquake in the area since the 1800s.
This post has been updated throughout
- earthquake!
- new york city
- the city politic
- kathy hochul
- what we know
Most Viewed Stories
- A Handgun for Christmas
- The Left-Wing Authoritarians Shutting Down the Democratic Party
- Arizona’s Senate Race Is a Battle Over the Nature of Reality
- Andrew Huberman’s Mechanisms of Control
- What to Know About the Latest Student-Loan Forgiveness Plans
- Who’s the Trump VP Pick? Latest Odds for Every Shortlist Candidate.
Editor’s Picks
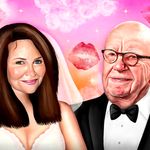
Most Popular
- A Handgun for Christmas By Lisa Miller
- The Left-Wing Authoritarians Shutting Down the Democratic Party By Jonathan Chait
- Arizona’s Senate Race Is a Battle Over the Nature of Reality By Olivia Nuzzi
- Andrew Huberman’s Mechanisms of Control By Kerry Howley
- What to Know About the Latest Student-Loan Forgiveness Plans By Lynnley Browning
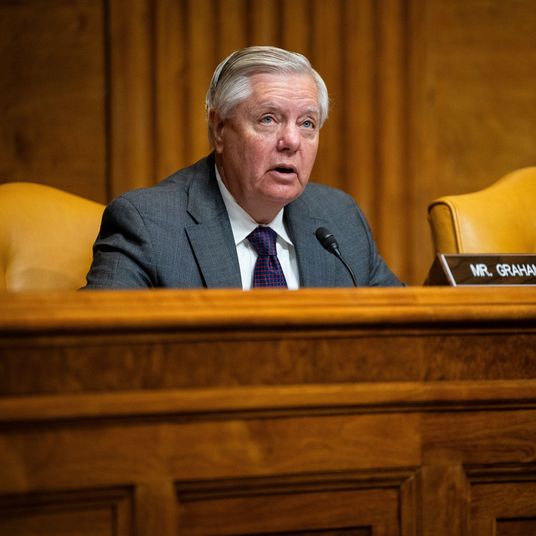
What is your email?
This email will be used to sign into all New York sites. By submitting your email, you agree to our Terms and Privacy Policy and to receive email correspondence from us.
Sign In To Continue Reading
Create your free account.
Password must be at least 8 characters and contain:
- Lower case letters (a-z)
- Upper case letters (A-Z)
- Numbers (0-9)
- Special Characters (!@#$%^&*)
As part of your account, you’ll receive occasional updates and offers from New York , which you can opt out of anytime.

Case study: The 2023 Türkiye–Syria earthquake

Case study: The effects of climate change in the Mekong Delta, Viet Nam
The 2023 türkiye– syria earthquake.
As a GCSE geographer you will learn about a range of natural hazards, their causes, impacts and how we can prepare for and respond to them. This article provides a case study of the massive and tragic earthquake that occurred in Türkiye, and the bordering country of Syria, in February 2023.
- Volume 35, 2023/ 2024
- Tectonic hazards
- Natural disasters
- (Global) Physical Geography
Michelle Minton

Earthquakes are not uncommon in this part of the world. However, the one that happened on 6 February 2023 had a magnitude of 7.8 and was the deadliest and strongest recorded in Türkiye since 1939, and was the world’s deadliest since the 2010 Haiti earthquake.
The earthquake’s epicentre was 34 km west of the city of Gaziantep in southern Türkiye, near the northern border of Syria (see back page). Türkiye is vulnerable to earthquakes as it sits on the Anatolian plate, between the large tectonic plates of Eurasia, Arabia and Africa. Within the Anatolian tectonic plate there are two major faults — the North Anatolian fault and the East Anatolian fault, and it is on the latter that the 2023 earthquake occurred.
Your organisation does not have access to this article.
Sign up today to give your students the edge they need to achieve their best grades with subject expertise
Related articles:
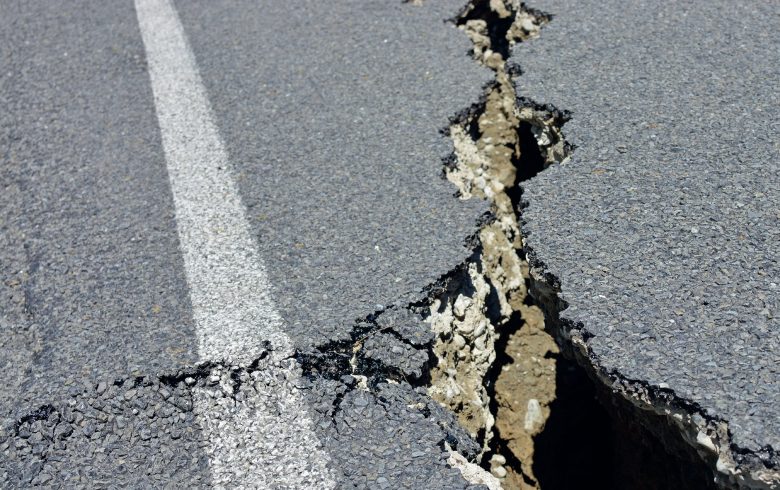
Geography online: Managing natural hazards
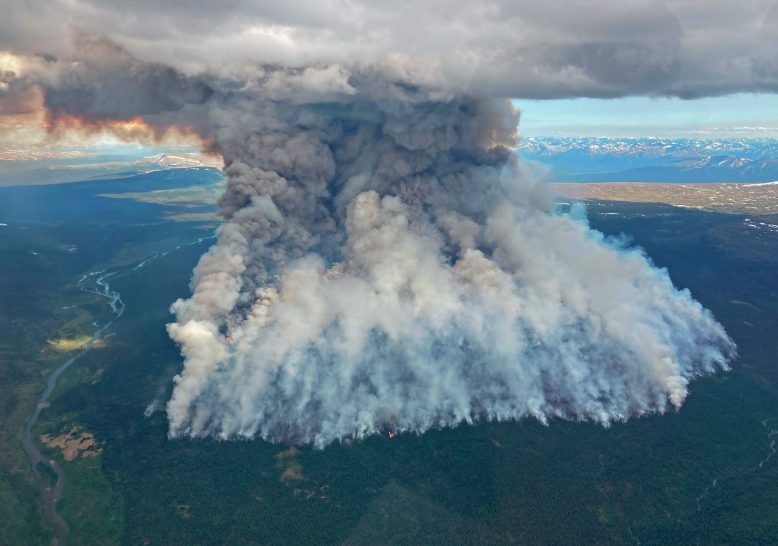
Case study: Wildfires in 2023
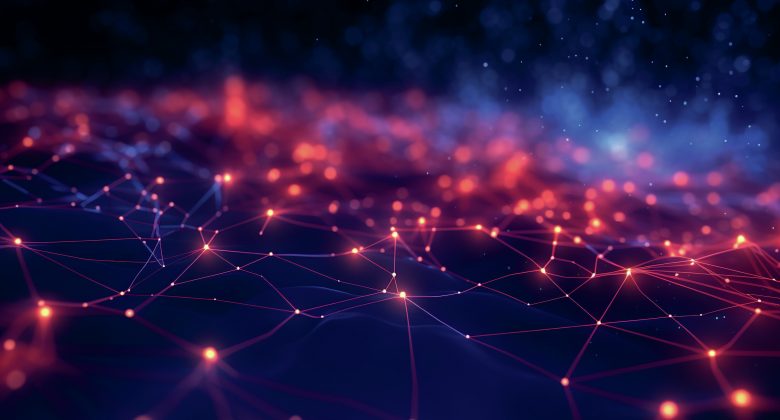
Geographical skills: Using radar diagrams to present your data
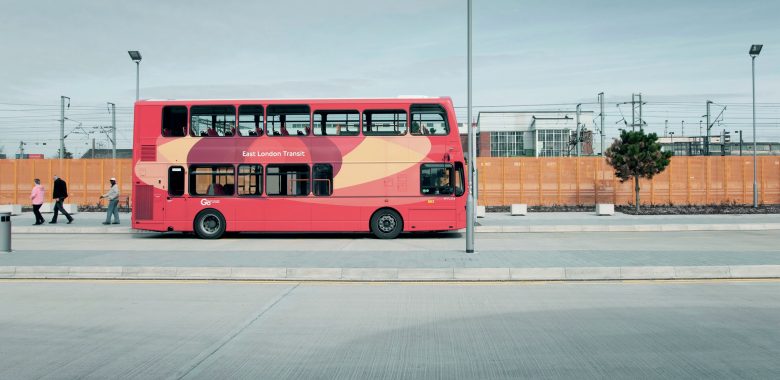
Case study: London's sustainable industrial future
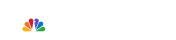
What causes an earthquake? Here's a brief explanation and how to stay safe
By dave price • published april 5, 2024 • updated on april 5, 2024 at 9:40 pm, what to know.
- Earthquakes are the result of rapid motion along a fault line. In Friday’s case, it occurred along the Ramapo Fault that runs down the center of the northern part of the state.
What will trigger an earthquake is a vertical shift in the earth — sometimes deep, sometimes shallow. That movement releases stored up energy.
- In the Northeast, where the Earth’s crust is brittle and earthquakes occur close to the surface, they are often felt far and wide. Quakes typically are quick, only lasting 10-30 seconds, like the one Friday morning.
For many across the tri-state, Friday’s earthquake may have been the first time experiencing such a wild and unpredictable occurrence.
Earthquakes in general are uncommon along the East Coast, and ones as powerful as the one that hit on Friday are exceedingly rare. There have only been three other times when magnitude near 5 or above have hit the tri-state area, and each of those was in the 18 th and 19 th century, respectively. It’s also been more than a decade since the area has even felt an earthquake as strong, when a 5.8 quake hit Virginia and the impacts were felt throughout the New York City area.
So what’s the science behind what happens to cause an earthquake? Here’s a look at the anatomy of the New Jersey quake and an explanation of the why and how of it all.
Get Tri-state area news and weather forecasts to your inbox. Sign up for NBC New York newsletters.
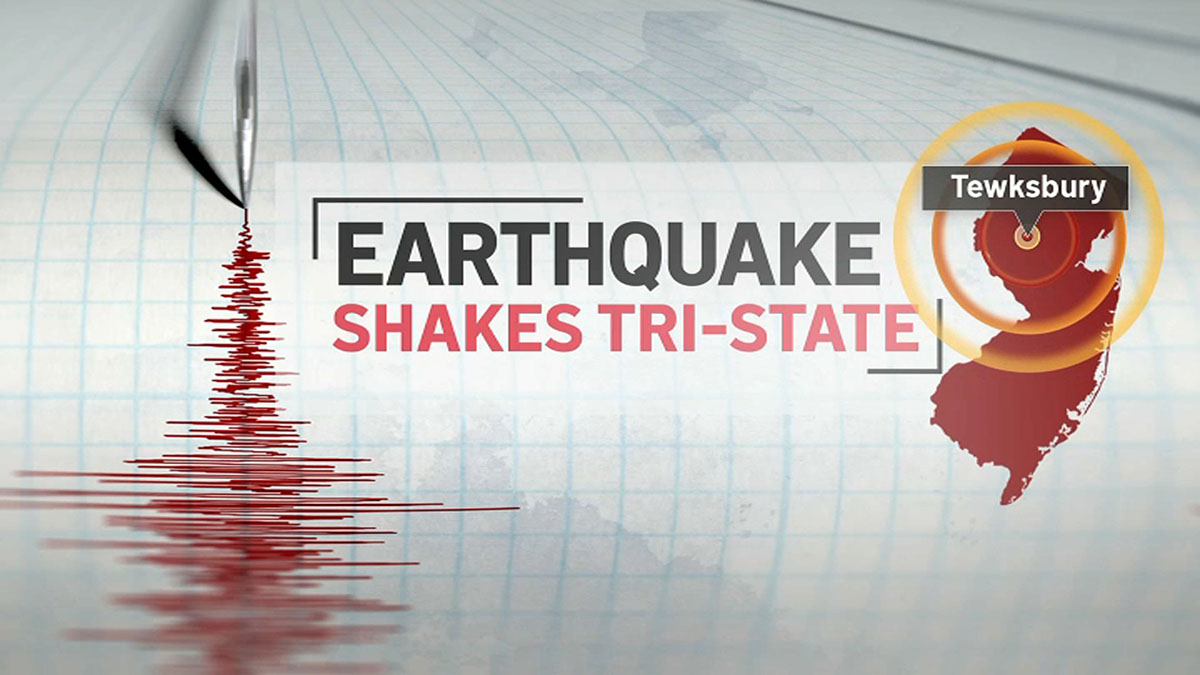
Magnitude 4.8 earthquake hits NJ, rattles entire tri-state; 4.0 aftershock felt hours later
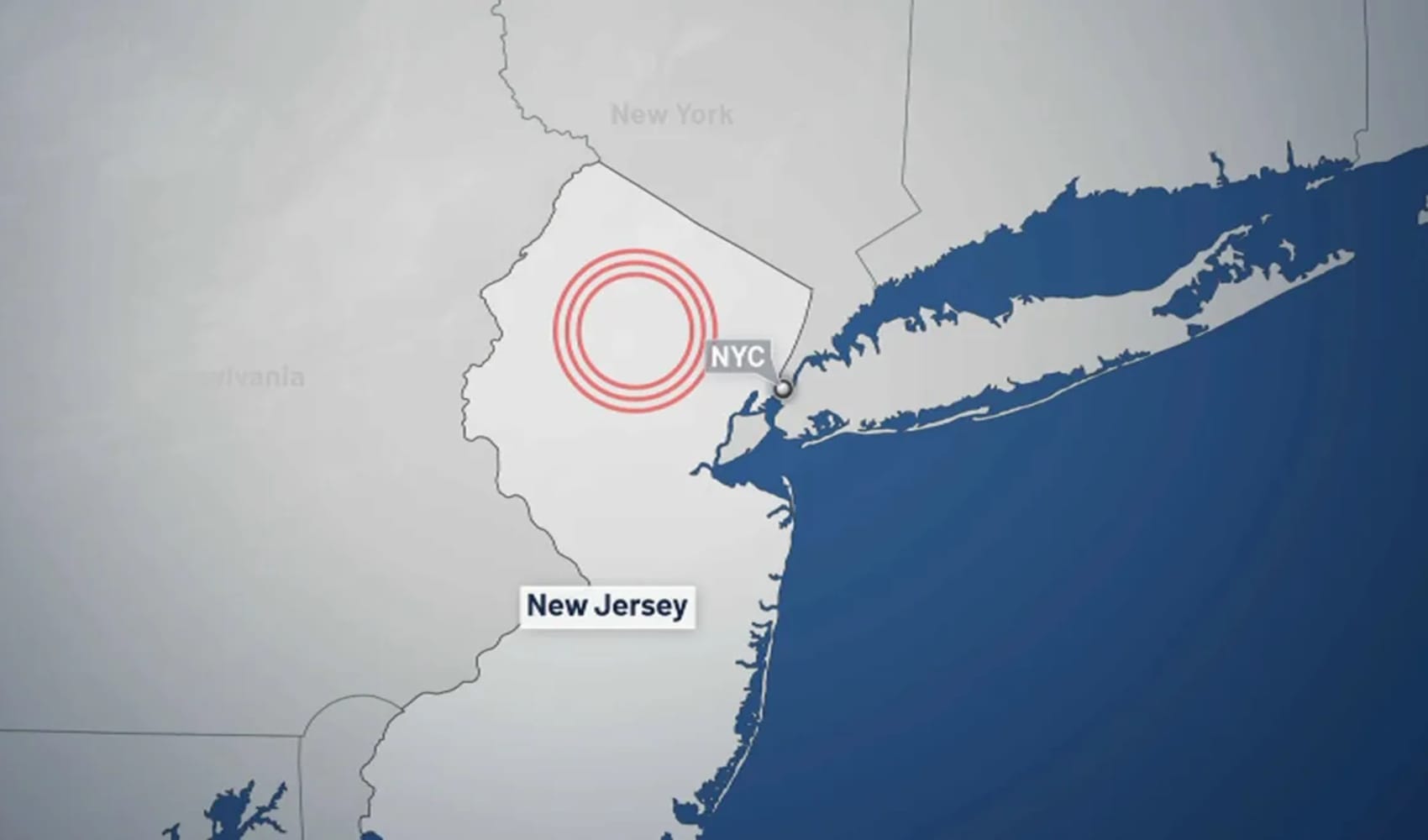
Can climate change make rare northeast earthquakes more common? Experts weigh in
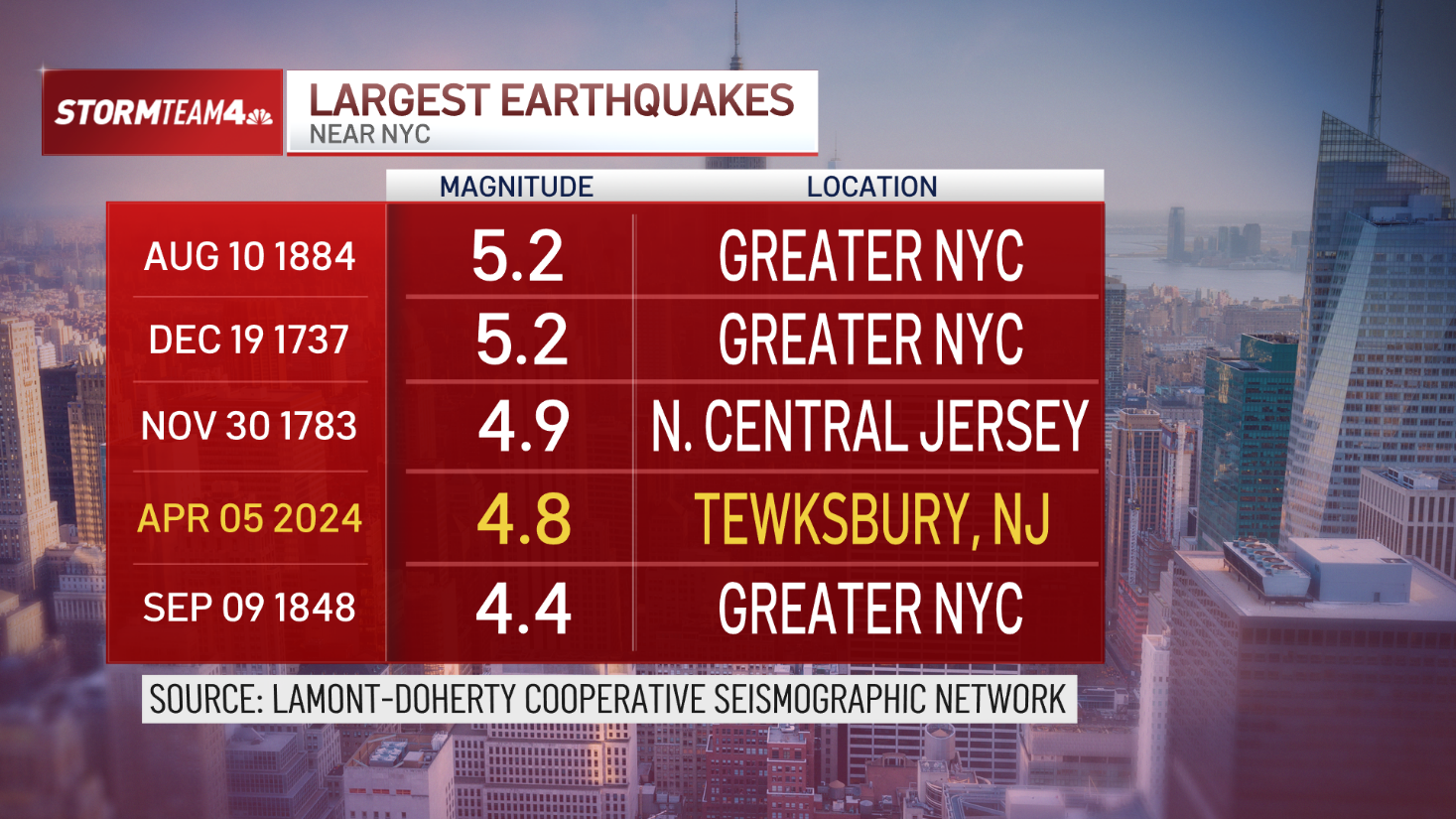
Where does today's earthquake rank in NYC area history?
What causes an earthquake.
Earthquakes are the result of rapid motion along a fault line. In Friday’s case , it occurred along the Ramapo Fault that runs down the center of the northern part of the state.
"The Ramapo fault which is the fault zone on which today's earthquake occurred, was active 200 million years ago. Plate tectonics is still responsible for the stress that builds up in the middle of plates," said Dr. James Davis, of the Lamot-Doherty Earth Observatory. "It's like if you're stretching a rubber band slowly so that you can't feel it at any given instant, but after a long time, when you let go, it will bounce back."
Millions of years of Earth's movement contribute to what was seen Friday. What Davis was referring to elastic strain, which causes seismic waves that cause the earth to shake.
In the Northeast, where the Earth’s crust is brittle and earthquakes occur close to the surface, they are often felt far and wide. Quakes typically are quick, only lasting 10-30 seconds, like the one Friday morning. But depending on the intensity and where it occurs, it can cause significant damage.
What made Friday’s earthquake exceptional is that it occurred in a densely populated corridor filled with skyscrapers and infrastructure, both above and below ground, in a region that simply doesn’t experience earthquakes of that magnitude often.
What's the difference between East and West Coast quakes?
The West Coast lies on a boundary where sections of Earth’s crust rub together, causing stress and slippage along fault lines that generate earthquakes relatively often.
East Coast quakes like Friday's are caused by compression over time of hard, brittle rock deep underground, according to Robert Thorson, an earth sciences professor at the University of Connecticut. “It’s like having a big block of ice in a vise and you are just slowly cranking up the vise,” he said. "Eventually, you’re going to get some crackling on it.”
These East Coast quakes can be harder to pinpoint. And they tend to affect a broader area. That’s because colder, harder East Coast rocks are better at spreading the rattling energy from an earthquake.
The distribution of cities across the East Coast also means that more people are around to experience the effects of a quake.
“We also have population centers over a large part of the northeast,” said Leslie Sonder, a geophysicist at Dartmouth College, “So a lot of people around here feel the earthquake.”
How do you stay safe during a quake?
Despite the infrequency of earthquakes, preparedness and communication are two key takeaways from this event. While the events can’t always be predicted, everyone can learn how to prepare for them.
USGS experts say there is a risk of aftershocks for weeks to months, which are expected after any earthquake. They recommend paying attention to emergency messaging from local officials.
To keep safe from shakes while sleeping, remove any furniture or objects that could fall and injure you or others.
If you feel shaking, drop where you are. Cover your head and neck with one arm, crawl under a table for shelter and hold on. If there’s no shelter nearby, grasp your head and neck with both hands until the shaking stops.
This article tagged under:
- Reference Manager
- Simple TEXT file
People also looked at
Original research article, case studies of multihazard damage: investigation of the interaction of hurricane maria and the january 2020 earthquake sequence in puerto rico.
- 1 Department of Civil and Environmental Engineering, University of Connecticut, Storrs, CT, United States
- 2 Department of Civil and Environmental Engineering, Rice University, Houston, TX, United States
This paper is motivated by the unique findings and observations from reconnaissance visits after the earthquake series in Puerto Rico in January 2020. It aims to discuss the potential interactions of Hurricane Maria and 2020 earthquake series and the considerations they underscore for future field reconnaissance missions. Traditionally, post-disaster damage assessment activities focus on one hazard and overlook the potentially cascading effects of multiple hazards on structures and infrastructure. This paper provides case studies showing the possible interaction of multiple hazards and their cascading effects observed in Puerto Rico. Infrastructure surveyed includes port facilities, buildings (particularly historical structures), and bridge structures. The data collected during the reconnaissance missions reveal how the impacts of Hurricane Maria, along with infrastructure aging and delayed repair and recovery activities, may have influenced the damage level and failure modes observed during the earthquake sequence a few years after. These case studies illustrate the nature of multihazard interactions and how these effects should be documented during post-disaster assessments. Beyond the insights gained from the case studies illustrated in this paper, the field survey instrument is provided as a basis for future reconnaissance studies, and the full set of reconnaissance data collected are published on the NSF funded NHERI DesignSafe cyberinfrastructure. As a result, this work not only provides data from Puerto Rico that can inform future damage and recovery modeling efforts, but also offers survey instruments and a field data collection process that is particularly tailored to cases where multihazard effects are at play.
1 Introduction
Regions across the United States and worldwide are generally at risk from more than one natural hazard which vary in spatial and temporal scale, occurrence potential, and intensity. These hazards may include earthquakes, hurricanes, floods, tsunamis, landslides, tornadoes, snowstorms, and fires, among others. Design engineers, infrastructure owners, risk managers, and other stakeholders are challenged to address the potential impacts of one or more natural hazards. Current design philosophies fail to consider the complex and intertwined effects of multiple hazards ( Roy and Matsagar, 2021 ). The development and implementation of effective design and risk mitigation strategies requires an understanding of the performance of structures and infrastructure in a multihazard environment, considering cases of concurrent, non-concurrent, or cascading hazards. Unique engineering challenges are posed by such multihazard problems ( Li et al., 2012 ). Ongoing work in the community ranges from characterizing the multihazard potential ( Gill and Malamud, 2014 ; Hayes et al., 2014 ), simulating behavior under cascading hazards ( Echevarria et al., 2015b ; Echevarria et al., 2015 ; Imani et al., 2015 ; Keller and Pessiki, 2015 ), testing structures under concurrent hazard loading ( Baheru et al., 2014 ), to modeling risk and resilience in multihazard settings ( Barbato et al., 2013 ; Kameshwar and Padgett, 2014 ; Echevarria et al., 2015a ; Jaimes et al., 2015 ; van de Lindt et al., 2015 ). Unfortunately, the lack of field data to support a variety of multihazard analyses to adequately characterize system failure modes or validate the existing models has slowed the progress in this domain ( Bruneau et al., 2017 ).
In this regard, the cascading effects of two inherently different events that impacted Puerto Rico presented a unique opportunity for researchers to collect invaluable data and provide empirical evidence for potential multihazard interactions. A team of researchers with diverse backgrounds in structural engineering, risk assessment, and risk mitigation for complex and interconnected infrastructure systems conducted reconnaissance missions to document and analyze the damages during the January 2020 earthquake series ( Miranda et al., 2020 ) with linkage to Hurricane Maria, the Category four storm that struck the island in September 2017 ( FEMA, 2018 ). The nomenclature proposed by Zaghi et al. (2016 ) to discuss multihazard design will be used in the framing of this paper. The case of two subsequent punctuated events, i.e., earthquake series and hurricane, as occurred in Puerto Rico is a classic case of non-concurrent multihazard scenarios. In such a case, degradation of structural capacity or shifts in boundary conditions from the primary event, along with the incremental or incomplete restoration processes prior to subsequent events, can have a significant impact on performance. This premise is illustrated below in Figure 1 . Here, it is shown how either a delayed repair or no repair negatively impacts system performance during a second event. For example, for a port structure, gradual decay makes the structure more vulnerable. After an event, the damaged structure could go through a faster decay than before as shown with the larger negative slope. However, this interaction between chronic stressors and multiple hazard events is not well understood, and data is scarce to fill this knowledge gap ( Zaghi et al., 2016 ).
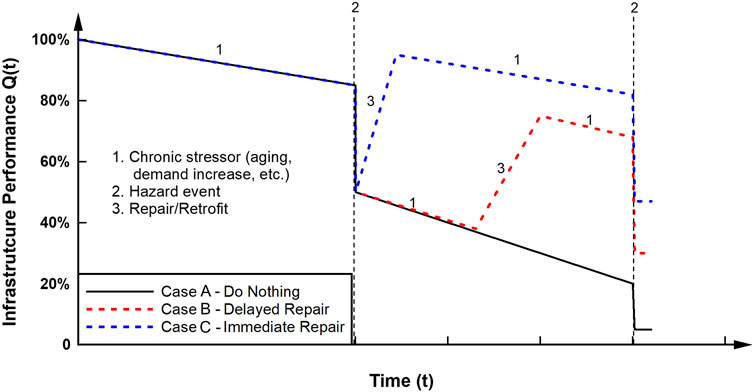
FIGURE 1 . Schematic showing change in system performance in subsequent hazards based on repair timeline.
While it is well known that earthquakes can have a different impact on structures that suffer from corrosion damage or scour ( Shiraki et al., 2007 ; Wang et al., 2014 ; Burke and Bruneau, 2016 ; Argyroudis et al., 2020 ), analyses are commonly performed on models of intact structures. This can be detrimental given that structures are rarely hit by an event in their intact form, i.e., new construction. Researchers have studied the combined effects of chronic and extreme stressors on the reliability and resilience of infrastructure systems throughout their lifetime ( Ouyang et al., 2012 ; Yang and Frangopol, 2019 ; Liu et al., 2020 ), underscoring the significance of considering the interactions of multiple hazards at different stages in the system’s service life, rather than modeling them independently. For the specific case of bridges ( Kumar and Gardoni, 2014 ; Padgett and Kameshwar, 2016 ; Vishnu and Padgett, 2020 ), several studies examined their vulnerability to impacts such as hurricanes and earthquakes, along with gradual stressors such as aging and sea-level rise, while emphasizing the pressing need for multihazard empirical data to support model development and validation. While several researchers have discussed procedures for multihazard risk assessment predicting cascading failures ( Eisenberg et al., 2020 ; Wei et al., 2022 ), the community lacks empirical data showcasing the impacts of such hazards.
During the investigation in Puerto Rico, it was found that some of the damage scenarios may be explained in a multihazard context that accounts for an interaction of impacts from the recent earthquakes and Hurricane Maria. The earthquakes exposed several unexpected and undocumented vulnerabilities of the infrastructure. As there is limited knowledge on the possible interactions of hurricanes and seismic events, the team investigated conditions that could lead to multihazard damages including: 1) structures and sites that experienced soil erosion during Hurricane Maria, 2) landslide-driven hydrologic effects of Maria that may be worsened during the earthquakes, 3) structural repairs that changed boundary conditions in subsequent events, 4) structures that showed signs of damage after the occurrence of the aftershocks, and 5) structural design concepts, such as elevated construction to avoid flooding, that makes structures more vulnerable to earthquakes.
This paper intends to present a thought-provoking series of case studies which encourage future reconnaissance missions through the lens of multihazard impacts. The main contributions of the work are: 1) presenting actual field observations and insights on multi-hazard interactions, 2) providing access to the comprehensive set of data collected, curated, and published for reuse on DesignSafe CI, and 3) sharing a field survey instrument and systematic field survey approach for future use in reconnaissance cases with potential multihazard effects. It is important to note that this paper relies heavily on observations, limited publicly available data, and the firsthand accounts of infrastructure owners or operators collected during the field visits. For that reason, significant interpretation was needed by the authors. The authors acknowledge that some of the findings presented may be viewed differently by other experts, but the aim is to share the data and information gleaned from conversations during our multiple visits and post-mission reflections. An overview of the region and susceptibility to hazards is presented first, followed by the details of four case studies of multihazard interactions. This work not only provides data from Puerto Rico that can inform future damage and recovery modeling efforts, but also presents survey instruments and a field data collection process that is particularly tailored to cases where multihazard effects are at play.
2 Overview of location and events
2.1 location of interest.
Ponce, Puerto Rico’s second largest city, is located on the island’s southern coast. During the 1800s, Ponce was an important port for shipping sugar, rum, coffee, and tobacco ( Tillman, 2009 ). As the sugar industry evaporated in the 20th century, the economy experienced a downturn that resulted in an increased reliance on tourism. In addition, the island has grappled with high unemployment and poverty rates, government disinvestment in public services, and crime. Natural hazards in this area expose vulnerabilities from colonial subjugation, economic hardship, environmental injustice, infrastructural neglect, and corruption from the island’s political class ( Lloréns, 2018 ). In the following section, we will provide an overview of the threat from natural disasters and detail the two phenomena that are the focus of this paper: Hurricane Maria and the 2020 earthquake series.
2.2 Overview of hazards
2.2.1 hurricanes.
Puerto Rico is subject to frequent and severe impacts from hurricanes, particularly between June and November ( Havidan, 1997 ; Emery et al., 2004 ; López-Marrero et al., 2019 ). Puerto Rico experiences some of the highest hurricane frequency in the North Atlantic basin. Storms typically approach from the east and south-east, and the most intense storms often form on the western coast of Africa and reach Puerto Rico near maximum intensity ( Emery et al., 2004 ). Based on data from 1900 to 2017, a total of 33 hurricanes passed over Puerto Rico or within 86 miles from the coast. Furthermore, 14 of the 33 reached wind velocities of Category three or higher ( López-Marrero et al., 2019 ).
The 2017 Atlantic hurricane season was one of the costliest and most destructive on record. For the first time, three Category four hurricanes made landfall in the US ( Blake, 2018 ). One such event was Hurricane Maria, which devastated Puerto Rico and many islands in the Caribbean. On 20 September 2017, Hurricane Maria’s center crossed the southeast coast of Puerto Rico near Yabucoa around 10:15 UTC. At that time, the maximum wind speed was 155 mph, which is just below Category five intensity. The hurricane’s center crossed the island from southeast to northwest and emerged into the Atlantic around 18:00 UTC ( Pasch et al., 2017 ). The estimated storm surge levels at the coast are shown in Figure 2 . The scale of the devastation, costliness of the damage, and delay in releasing federal aid left critical infrastructure unrepaired prior to the earthquake series.
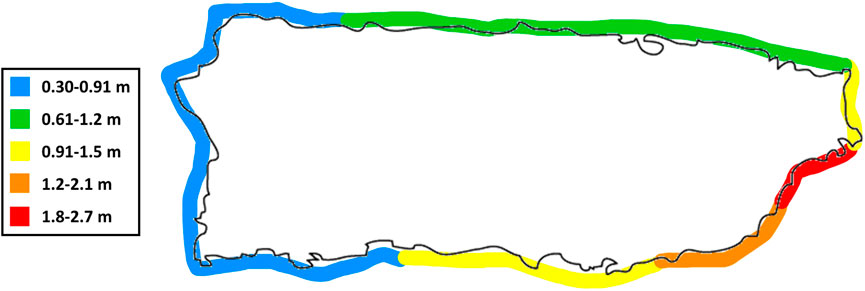
FIGURE 2 . Estimated storm surge inundation along the coasts of Puerto Rico adapted from Pasch et al. (2017) .
2.2.2 Earthquakes
The island of Puerto Rico is located between the North American and the Caribbean tectonic plates. The boundary is characterized primarily by left-lateral motion along east-west striking faults ( Jansma et al., 2000 ). The Puerto Rico-Virgin Islands microplate is one of three microplates located in this complex plate boundary zone ( Raussen and Lykke-Andersen, 2009 ), and is part of the Caribbean plate. On the northern side of the island, the North American plate is subducting obliquely beneath the Caribbean plate ( Vičič et al., 2022 ). This is bounded by the east-west striking Puerto Rico trench ( Jansma et al., 2000 ). On the southern side of the island, the Muertos trough, an east-west striking fault defines the southern limit of the Puerto Rico-Virgin Islands microplate ( Jansma et al., 2000 ). Seismicity in and around Puerto Rico averages hundreds of earthquakes per year ( Jansma et al., 2000 ).
A series of significant seismic events occurred in the southwestern region of Puerto Rico in early January 2020. The first major event (M w 5.8) occurred on January 6th at 10:32 a.m. UTC. The second significant event (M w 6.4) occurred on January 7th at 8:24 a.m. UTC. The third significant event (M w 5.6) occurred on January 7th at 11:18 a.m. UTC. These earthquakes caused significant damage to many structures near Guayanilla and Ponce, Puerto Rico. The start of the seismic events occurred on 28 December 2019, and the region continued to experience aftershocks as of March 2020 ( Miranda et al., 2020 ). A summary of all magnitude five and larger earthquakes between 29 December 2019 and 17 January 2020 are listed in Table 1 ( Van Der Elst et al., 2020 ). The PGA contours from the M w 6.4 event are shown in Figure 3 .
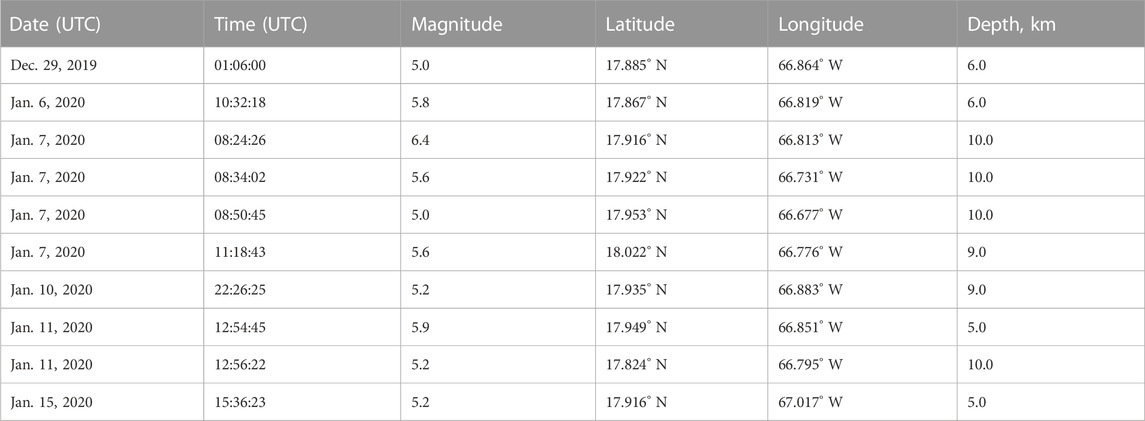
TABLE 1 . Summary of magnitude five and larger earthquakes between December 29th, 2019–17 January 2020 ( Van Der Elst et al., 2020 ).
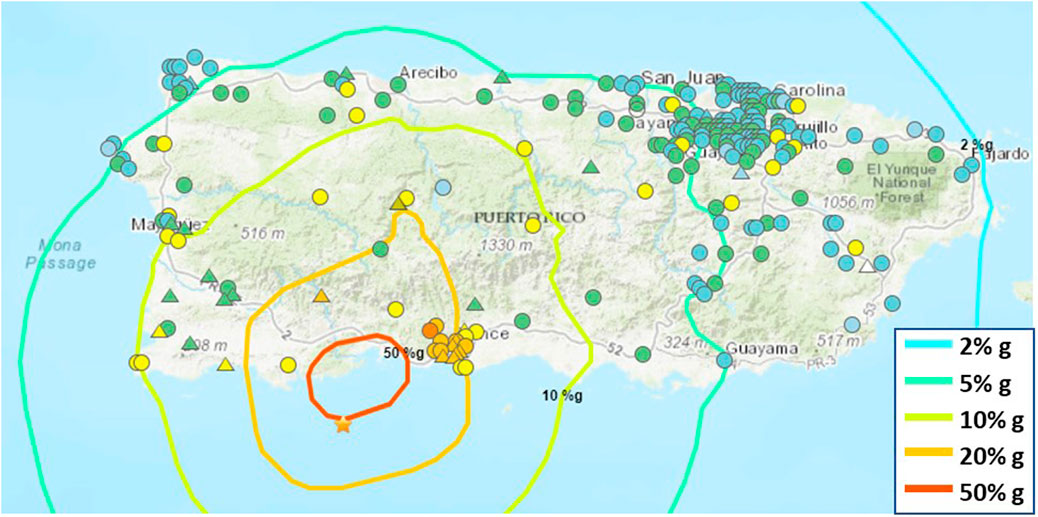
FIGURE 3 . PGA contours estimated from ShakeMap for 7 January 2020, M w 6.4 earthquake ( USGS, 2020 ).
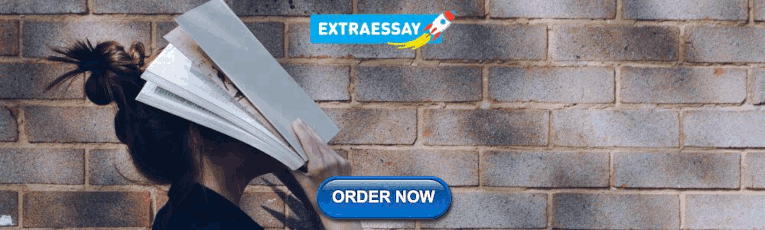
3 Reconnaissance methods
The reconnaissance missions were funded through an NSF RAPID Project. The first visits were conducted January 23rd-24th and February 24th-26th, 2020. The final visit was set to occur in March 2020 but was delayed due to COVID-19 travel restrictions. When travel bans were lifted, the final visit was conducted on April 21st-23rd, 2021. The inspections focused on structures in Southern Puerto Rico that sustained damages during the earthquake series. The damages were compared to previous seismic events to determine if the level of damage was consistent with the magnitude of the earthquake or if additional factors likely contributed to the damage. Traditional post-disaster damage assessment activities focus on one hazard and overlook the potentially destructive cascading effects of multiple hazards on structures and infrastructure. Therefore, the focus of this study was to identify the compounding impacts of Hurricane Maria and the 2020 earthquake series. Through this inspection, a comprehensive data set was collected to understand if the impacts of Hurricane Maria resulted in an increased level of damage or contributed to unexpected modes of failure during the recent earthquake series.
Multiple methods were used to ensure a comprehensive set of data was collected. The data collection methods included a damage assessment survey, multiple image types, images and videos taken with an unmanned aerial system (UAS) operated by a team member, and Lidar scan data. All data from the missions can be found on DesignSafe-CI ( Zaghi et al., 2021 ; Hain et al., 2022 ). The team made use of the RAPID Facility Field Data Collection App (Rapp) survey application to ensure a systematic approach toward data collection ( Berman et al., 2020 ). The survey included guiding questions to be answered at each site location at the start of data collection. The survey questions are included below in Table 2 . The survey is meant to be used in the numerical order shown. The damage assessment survey was completed by 2-3 of the team members to ensure it the findings were consistent.
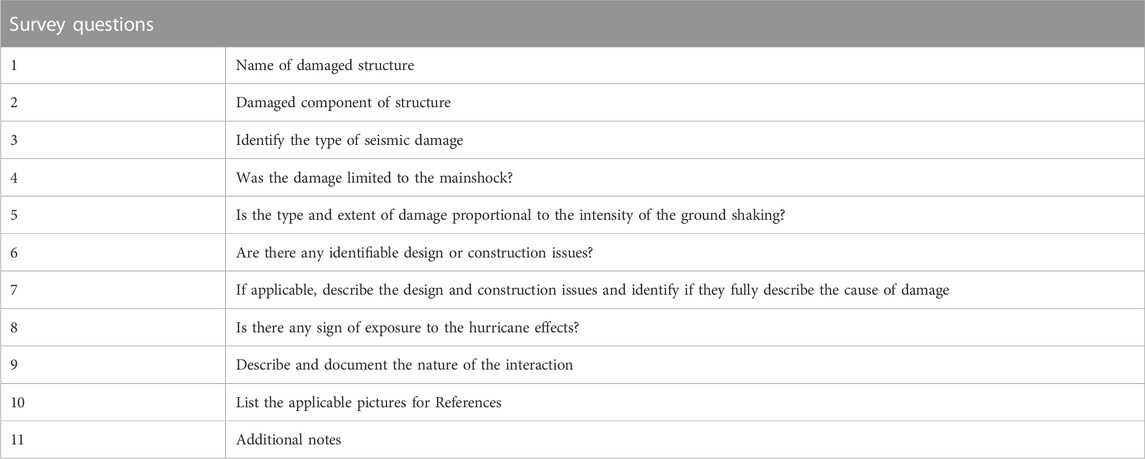
TABLE 2 . Damage assessment survey.
4 Results from multihazard case studies
4.1 case 1: historic buildings in downtown ponce, pr shock-aftershock.
One of the most prevalent examples of multihazard events is the successive nature of the initial earthquake and corresponding aftershocks. While most earthquakes are accompanied by aftershocks, typical designs only consider the maximum magnitude event. As the research team visited Puerto Rico on three occasions after the initial earthquake series, they were able to evaluate the progression of structural damages in the aftershock events.
As the economy of the Ponce region is largely focused on tourism, the team focused on historical structures. These buildings were vulnerable to seismic loads for several reasons, including their age and the brittle nature of the construction materials and structural systems. A sample of the damage progression over time is shown in Figure 4 . During the initial events, this building sustained severe damage including shear cracking at the awning and at multiple locations along the roof as shown in Figures 4A, B . At this point, the building was roped off and entry was halted for safety concerns. As the aftershocks continued, the damaged worsened, as shown in Figure 4C . One of the shear cracks along the side of the building expanded and the brick wall collapsed which exposed the structural support system of the roof. It is also important to note that the front of the structure was supported by temporary bracing that was installed after the main shock, which prevented the collapse of this portion. This shows that minimal external bracing/shoring or strengthening is an effective strategy in preventing unrecoverable damage or total collapse during aftershocks. This is particularly important for brittle structures that lack an engineered system to provide ductility and energy dissipation, and those with historic significance. To minimize damage in subsequent events it is critical to first identify the repair priorities and then allocate the resources needed to execute the repairs. When identifying repair priorities, safety, economic impact, and historical significance should be considered.
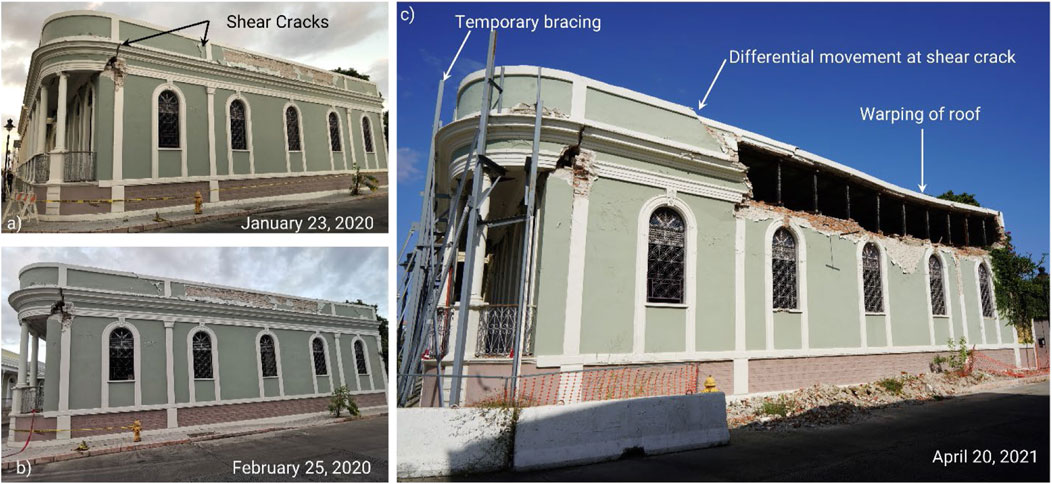
FIGURE 4 . Damage progression of sample historical building in downtown Ponce under aftershock events. Images were taken during consecutive visits on (A) 23 January 2020, (B) 25 February 2020, and (C) 20 April 2021.
A second example of a historical building that suffered damage during the initial earthquake series is shown below in Figure 5 . In Figure 5A , shear cracks on the unreinforced masonry wall around the window opening are visible. This hotel is located in downtown Ponce. It experienced temporary closures after the damage was discovered from the mainshock. The damage was repaired quickly, and the window was removed to form a more robust lateral load resisting system as shown in Figure 5B . This illustrates how the timely implementation of repairs can protect vulnerable, but significant, structures from increased damage.
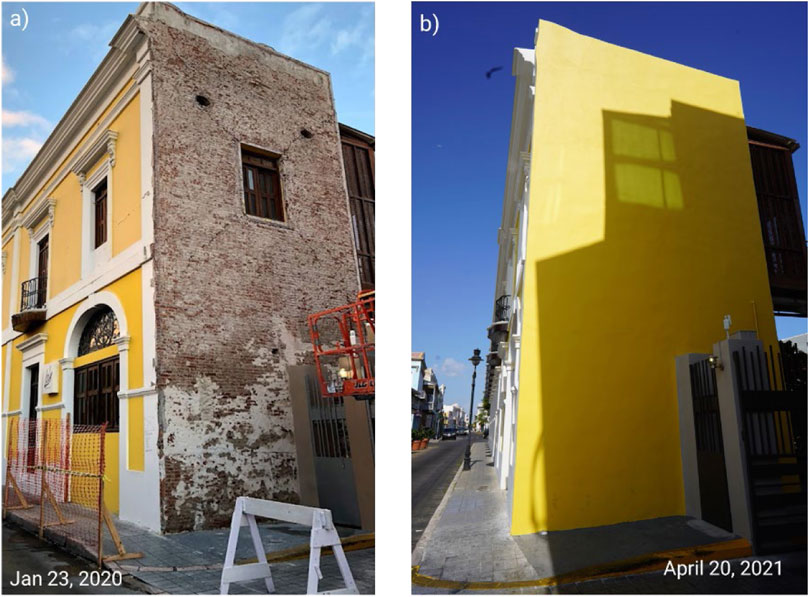
FIGURE 5 . Historical hotel in Downtown Ponce showing (A) damage after initial earthquake series and (B) view of repaired wall segment.
In the context of societal and economic impacts, damages after the hurricane and the slow recovery led to mass migration from the affected areas. In the aftermath of the earthquake series, the number of individuals leaving Puerto Rico increased ( Sanchez, 2020 ; Aranda et al., 2022 ). This included a large number of small business owners supporting the tourism industry. The research team identified that a lack of, or delayed, access to federal funding through FEMA significantly slowed the economic recovery of the region, which adds to the vulnerability. For example, the team noticed that several historic buildings in Ponce that needed restoration after the earthquakes were abandoned by the owners. At the time of the visits, the city officials were in conversation with the historic preservation community to evaluate if some of these buildings have to be demolished because of public safety concerns.
4.2 Case 2: Rafael Cordero Santiago Port of the Americas, Hurricane Maria–earthquake series
This case study details the multihazard impacts from punctuated stressors, i.e., Hurricane Maria and the earthquake series. The extent of damage on ports facilities due to hurricanes is often directly correlated to the proximity of the port to the hurricane landfall. Damages can be attributed to several characteristics of the hurricane, including storm surge, wave heights, wind speed, debris impact, and the duration of exposure. Hurricane damages can include disruptions to key features, such as the navigation channel, or structures, such as piers and wharfs, as well as destruction of ancillary structures, equipment, and cargo throughout the port ( Wakeman, 2013 ). While the port experienced notable damage from Hurricane Maria alone, this section will focus on the physical impacts from the storm that influenced its performance during the earthquake series.
This case highlights one pier that sustained severe damage during the earthquakes, shown below in Figure 6 . The level of damage sustained was greater than what is expected from earthquakes of this magnitude, suggesting additional factors contributed to the damage. Major damage types included seaward displacement of panels ( Figures 6A, C ), bowing of the berth ( Figure 7 ), and collapse of panels ( Figures 6A, B ). Upon inspection, the team determined the slab failure of the pier that occurred during the earthquake series was caused by compromised support conditions. Key personnel from the site noted washout from the pier was evident after Hurricane Maria. In addition, evidence of coastal erosion in the region was noted in Geotechnical Extreme Events Reconnaissance report following the hurricane ( Silva-Tulla et al., 2018 ). The erosion of the supporting soil fill, as shown in Figure 6B , made the unreinforced slab-on-grade vulnerable to the seismic shaking.
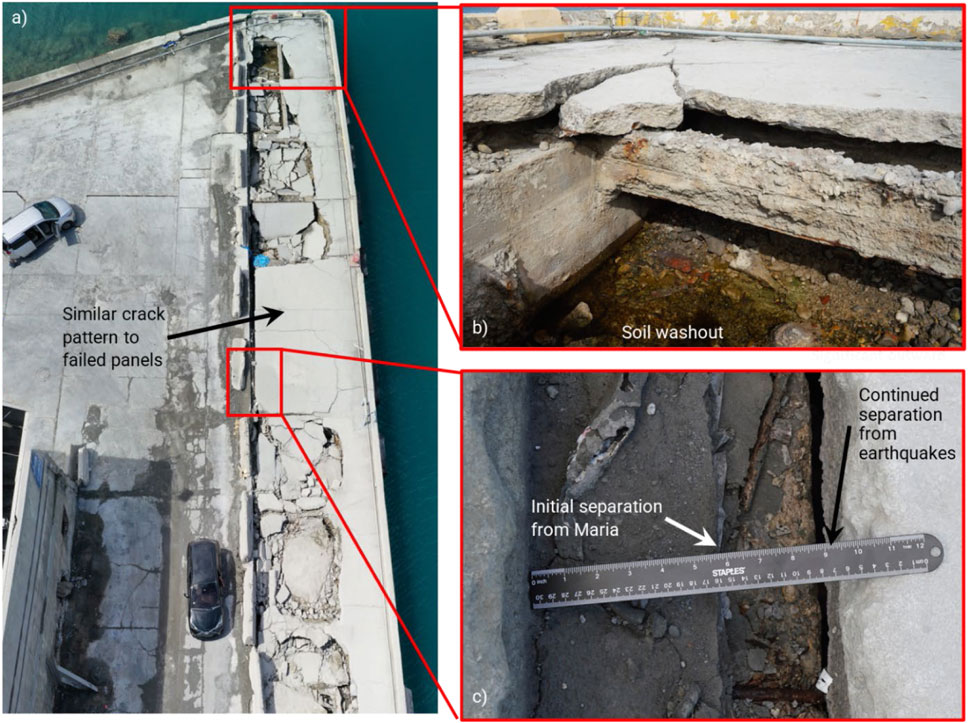
FIGURE 6 . Images of damages to Pier following January 2020 earthquake series including (A) an aerial view of the overall damage, (B) washout of soil underneath collapsed panel, and (C) representative image showing separation of main slab and pier.
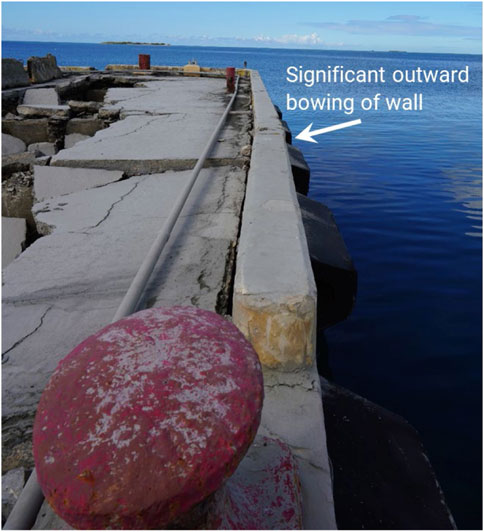
FIGURE 7 . View of bowing of berth at Pier 2.
As shown in Figure 7 , there was severe bowing of the berth at the upper region of the pier. This bowing shows an area on the edge of the pier that is experiencing larger outward displacements compared to other areas along the length, which suggests more significant pile damage at this location. It is unlikely the bowing is caused by large soil or water pressure at this location, as washout of the soil fill within the damaged regions was observed.
The port was closed for a short period of time due to damage incurred from the hurricane, yet the port was partially restored due to its critical role. While the port was allocated funds to address damages from Hurricane Maria, at the time of inspection the federal funds still had not been released. Due to this delay, the condition of many structures continued to degrade, worsening the performance in the earthquake series. It is not in the scope of this paper to discuss the reasons for delaying funds, rather we aim to highlight the ramification of such actions in terms of safety and higher overall repair costs following subsequent events. This should be a key consideration for policymakers when responding to and planning for future natural disasters.
The damages caused significant delays and postponement of the expansion plans, which were to transform the port into an integrated transport center and logistics platform for international trade in the Caribbean region. The goal of this development strategy was to convert the facility into a third-generation, multi-cargo port, in order to meet the growing needs of the container ship sector and the demands of globalization. This delay has exacerbated the economic impact of the disaster to the affected region, as the lack of capacity to manage the increased cargo volume has led to further economic losses.
4.3 Case 3: Rafael Cordero Santiago Port of the Americas, chronic stressors–earthquake series
Ports are located in highly-corrosive coastal environments. The two most commonly used structural materials, steel and reinforced concrete, are both vulnerable to corrosion deterioration ( Maniglio et al., 2021 ). As such, ports are at a higher risk of accelerated damage due to corrosion compared to other structures. Design considerations, such as increasing the thickness of the cover concrete, using corrosion resistant reinforcements such as epoxy coated or stainless-steel rebar, or including sacrificial thickness or a concrete coating for steel piles, can mitigate the adverse impacts of corrosion damage. However, implementing such strategies often results in increased costs and not all options were available during the construction of older port structures. For reinforced concrete elements, such as beams, slabs, and piles, corrosion of reinforcement is likely to occur if widespread cracking or insufficient cover is present. Corrosion of the reinforcement leads to the spalling of cover concrete, which then further exposes reinforcement. This corrosion weakens the structural elements and may lead to widespread damage under extreme loadings, such as hurricanes and earthquakes.
The majority of the earthquake damage at the ports inspected was exacerbated by underlying corrosion of the structure. This was likely worsened or initiated in Hurricane Maria due to acceleration of corrosion damage in structural components due to increased exposure to sea water. The high level of corrosion present contributed to the spalling and failure of elements in the seismic events. Piers had substantial corrosion in the superstructure. This included the beams ( Figure 8A ), support structures for slabs ( Figure 8B ) and the concrete barrier surrounding the edge of the piers ( Figure 8C ). In many cases, the corrosion damage was not observable prior to the earthquakes when the concrete spalled, but the level of corrosion in the exposed elements indicates that corrosion has been occurring for some time. This makes it challenging to identify corrosion in inspections and, therefore, unlikely to be repaired until it reaches a critical level. Understanding the progression of corrosion and how this deterioration impacts structural behavior under extreme events is critical for predicting performance of aging structures and should be considered in design.
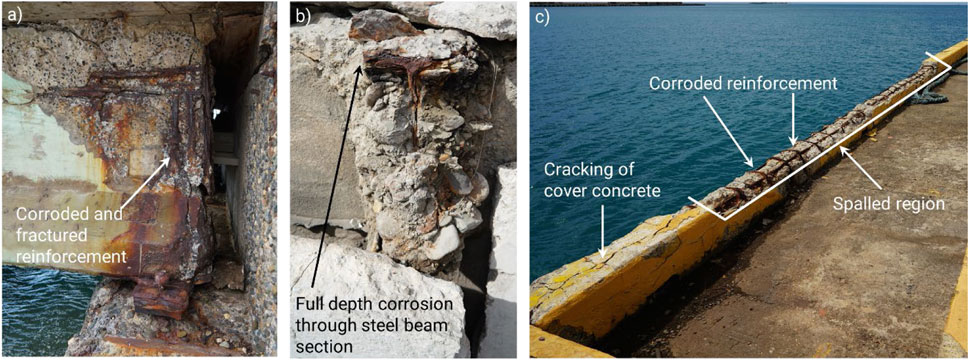
FIGURE 8 . Elements at the ports inspected had severe corrosion damage that was observable after the earthquake series. Sample views of different elements with visible deterioration from corrosion include (A) beams, (B) support structures for slabs, and (C) concrete barrier surrounding the edge of the piers.
While the economic impact of this multihazard scenario is not immediately evident, it can be viewed from the perspective of the expansion plans discussed earlier. Any expansion requires repair and replacement of the existing corrosion damaged elements. This could potentially lead to delays and increased costs due to the need to repair or remove the existing structures.
4.4 Case 4: Bridge in Guayanilla, Hurricane Maria—earthquake series
During Hurricane Maria, the Guayanilla River flooded, which resulted in washout and debris impacts. This was observed on the bridge carrying PR-127, which spans the river. The washout was most severe at the abutment, where exposed piles can be seen on the left of Figure 9A . The bridge was closed and required repair, including backfilling behind the abutment and fill work in front of the abutment ( Maria, 2017 ). This bridge was not visited in the missions in 2020, but after seeing the drone footage from after Hurricane Maria, was added to the inspection list for the 2021 mission. Following the earthquake series and as of April 2021, the bridge remained open to traffic. A panoramic view of the bridge taken with a drone is shown in Figure 9B . Upon further inspection, the research team found several examples of seismic damage, including cracking at the abutments from pounding, bent anchor bolts at the bearings, and spalling at the top of the columns, as shown in Figures 9D, E . There was also evidence of geotechnical damage, as shown in Figure 9C , but it was not clear if this was left over from hurricane Maria, or a result of the earthquakes.
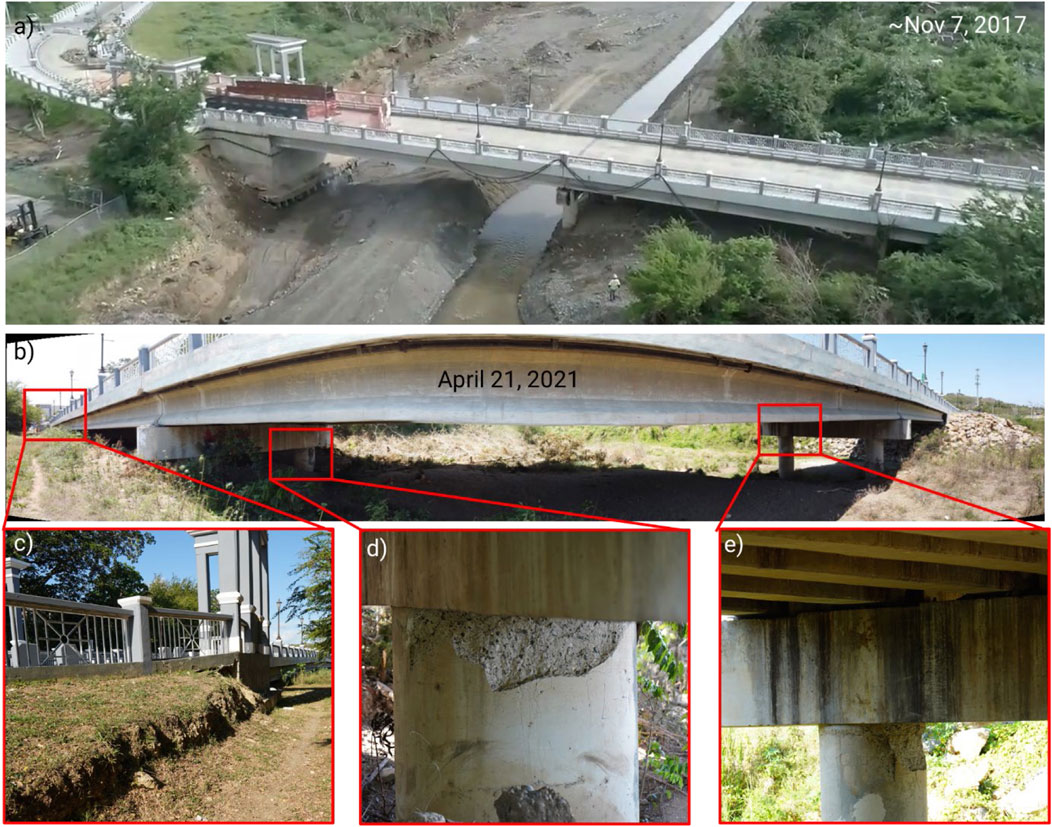
FIGURE 9 . Bridge in Guayanilla, PR over the Guayanilla River. These photos include (A) a screenshot from a drone flyover taken 50 days after hurricane Maria ( Maria, 2017 ), (B) an overall view of the bridge taken on the final reconnaissance mission, (C) the geotechnical impacts observed at the abutment, (D) cracking on support column on Pier 1, and (E) cracking of support column on Pier 2.
This case study was included to show an example of where the multihazard effect was not clear. While there was no direct evidence, the soil washout that occurred at the abutment and piers may have contributed to more extensive damage to the bridge columns than was observed at other bridges in the area. In addition, if this spalling is not repaired in a timely fashion, the column reinforcement at the critical plastic hinge zone will be subjected to premature corrosion damage, which will further weaken the structure.
The restoration of transportation infrastructure was prioritized in the aftermath of the hurricane, and the bridge structures visited were found to be in a relatively good condition. This was an important factor in avoiding any disruptive failure of the bridges after the earthquakes, thus ensuring continued access to the impacted areas.
5 Discussion
The cases presented illustrate multihazard impacts observed on different structure types and under different hazards. Multihazard reconnaissance is significantly more challenging because the state of the structure is not always documented after the first event, or through time. In addition, a consistent data collection approach is needed to ensure that the data collected at different times are comparable in terms of location and focus. The founding of the Structural Extreme Events Reconnaissance (StEER) Network in 2018 was a notable first step in this direction. However, we need to promote the use of a multihazard approach in reconnaissance. The traditional approach has been focused on documenting damage from a single event and ensuring meaningful information can be extracted from the observation data. It is important to evaluate the damages in the context of the condition of the structure and the potential interaction of multiple hazards, otherwise the findings may be misleading. Findings from reconnaissance are used to inform changes to design codes, thus it is critical to make sure the correct conclusions are drawn. For example, if we see failure of concrete elements without paying attention to level of corrosion or a change in the support conditions, we may misattribute the cause. While the data in this paper was not directly used to assess safety levels of the structures noted, it is our hope that the data presented will encourage the multihazard perspective in future reconnaissance missions. The safety assessment process requires well-calibrated and generalizable analytical models of structural types and systems, which is a major undertaking that was outside of the scope of this effort. However, as an intermediate approach, additional questions can be added to surveys in current field safety evaluation protocols and manuals to note potential evidence of prior damages due to other hazards or aging as well as the interaction of effects from multiple hazards.
Another consideration for the future of multihazard design in regions such as Puerto Rico is addressing conflicting design objectives. The design of buildings in Puerto Rico is largely dependent on the location of the structure. Most buildings in coastal areas of the island are concrete structures. These buildings resist high winds, but their large mass makes them vulnerable to the earthquakes. In addition, elevated construction is used for the buildings to prevent water damage due to storm surge. However, this system is vulnerable to earthquakes because of the soft story behavior as shown in Figure 10A . As additional regions become exposed to new and more extreme natural hazards due to climate change, the research on multihazard damages and design will need to be implemented in design codes.
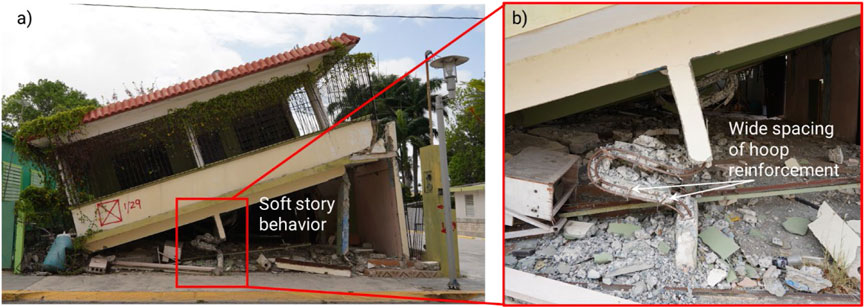
FIGURE 10 . Damage of building in Guánica, PR following earthquake series showing (A) overall damage and soft-story failure mode and (B) close up of support column collapse.
We would be remiss not to note the importance of contractors and inspectors adhering to designs. During the reconnaissance mission, the team overserved many buildings that appeared to have construction flaws such as poor-quality concrete or insufficient steel reinforcement ( Figure 10B ). Due to the limited information available to the team, it is unclear if the issues were caused in the design or construction phase. While these findings do not fall under the purview of multihazard design or inspection per se , they are critical components of forensic engineering to ascertain failure patterns.
6 Conclusion
This paper presents a series of case studies from multiple reconnaissance missions in the region surrounding Ponce, PR, analyzing the damages from the January 2020 earthquake series with linkage to Hurricane Maria, the Category four storm that struck the island in September 2017. In cases of subsequent hazards, degradation of structural capacity or shifts in boundary conditions from the primary event, along with the incremental or incomplete restoration processes prior to subsequent events, can have a significant impact on performance. The cascading effects of the two inherently different events that impacted Puerto Rico presented a unique opportunity for researchers to collect invaluable data and provide empirical evidence to characterize multihazard interactions.
Beyond the key insights gained from investigation of the case studies described in this paper, this study introduced a new field survey instrument. This instrument emphasized unique considerations for future field reconnaissance missions to systematically consider the potential for multiple event effects over short and long time periods. Moreover, the full dataset from multiple waves of reconnaissance was published on NHERI’s DesignSafe cyberinfrastructure platform to allow sharing and data reuse. Such data could provide a future opportunity to verify and validate structural system level damage and restoration models, particularly for the understudied area of life-cycle resilience in which incomplete recovery or damage accumulation affects subsequent event performance.
Data availability statement
The datasets presented in this study can be found in online repositories. The names of the repository/repositories and accession number(s) can be found in the article/supplementary material.
Author contributions
AH, AZ, and JP contributed to conception and design of the study, AT organized the database, AH, AZ, AT, and JP interpreted the results. AH wrote the first draft of the manuscript. AZ, AT, and JP wrote sections of the manuscript. All authors contributed to manuscript revision, read, and approved the submitted version.
This paper presents an overview of the reconnaissance missions in Ponce, PR and the surrounding region to document multihazard impacts from Hurricane Maria and the January 2020 earthquake series. The missions, data processing and curation were conducted under NSF Awards #2022390 and #2022427.
Acknowledgments
The authors would like to acknowledge the support and guidance of the RAPID Facility at the University of Washington, particularity Dr. Michael Grilliot who assisted with data collection and processing from the first field visit. In addition, the project would not have been possible without Angela Lanning, who supported the field missions and lead the archiving of the data.
Conflict of interest
The authors declare that the research was conducted in the absence of any commercial or financial relationships that could be construed as a potential conflict of interest.
Publisher’s note
All claims expressed in this article are solely those of the authors and do not necessarily represent those of their affiliated organizations, or those of the publisher, the editors and the reviewers. Any product that may be evaluated in this article, or claim that may be made by its manufacturer, is not guaranteed or endorsed by the publisher.
Aranda, E., Blackwell, R., Escue, M., and Rosa, A. (2022). Cascading disasters: The impact of hurricane Maria and Covid-19 on post-disaster Puerto Rican migrants’ adaptation and integration in Florida. Lat. Stud. 2022, 1–24. doi:10.1057/s41276-022-00390-3
PubMed Abstract | CrossRef Full Text | Google Scholar
Argyroudis, S. A., Mitoulis, S. A., Hofer, L., Zanini, M. A., Tubaldi, E., and Frangopol, D. M. (2020). Resilience assessment framework for critical infrastructure in a multi-hazard environment: Case study on transport assets. Sci. Total Environ. 714, 136854. doi:10.1016/j.scitotenv.2020.136854
Baheru, T., Chowdhury, A. G., Bitsuamlak, G., Masters, F. J., and Tokay, A. (2014). Simulation of wind-driven rain associated with tropical storms and hurricanes using the 12-fan Wall of Wind. Build. Environ. 76, 18–29. doi:10.1016/j.buildenv.2014.03.002
CrossRef Full Text | Google Scholar
Barbato, M., Petrini, F., Unnikrishnan, V. U., and Ciampoli, M. (2013). Performance-based hurricane engineering (PBHE) framework. Struct. Saf. 45, 24–35. doi:10.1016/j.strusafe.2013.07.002
Berman, J. W., Wartman, J., Olsen, M., Irish, J. L., Miles, S. B., Tanner, T., et al. (2020). Natural hazards reconnaissance with the NHERI RAPID facility. Front. Built Environ. 6, 573067. doi:10.3389/fbuil.2020.573067
Blake, E. S. (2018). The 2017 atlantic hurricane season: Catastrophic losses and costs. Weatherwise 71 (3), 28–37. doi:10.1080/00431672.2018.1448147
Bruneau, M., Barbato, M., Padgett, J. E., Zaghi, A. E., Mitrani-Reiser, J., and Li, Y. (2017). State of the art of multihazard design . Virginia, United States: American Society of Civil Engineers .
Google Scholar
Burke, S., and Bruneau, M. (2016). Effect of surface roughness on cyclic ductility of corroded steel. J. Struct. Eng. 142 (6), 04016014. doi:10.1061/(asce)st.1943-541x.0001425
Echevarria, A., Zaghi, A. E., Chiarito, V., Christenson, R., and Woodson, S. (2015). Experimental comparison of the performance and residual capacity of CFFT and RC bridge columns subjected to blasts. J. Bridge Eng. 21 (1), 04015026. doi:10.1061/(asce)be.1943-5592.0000762
Echevarria, A., Zaghi, A. E., Christenson, R., and Accorsi, M. (2015a). CFFT bridge columns for multihazard resilience. J. Struct. Eng. 2015, C4015002.
Echevarria, A., Zaghi, A. E., Christenson, R., and Plank, R. (2015b). Residual axial capacity comparison of CFFT and RC bridge columns after fire. Polymers 7 (5), 876–895. doi:10.3390/polym7050876
Eisenberg, D. A., Park, J., and Seager, T. P. (2020). Linking cascading failure models and organizational networks to manage large-scale blackouts in South Korea. J. Manag. Eng. 36 (5), 04020067. doi:10.1061/(asce)me.1943-5479.0000820
Emery, R., Serrano, M. I., and Foster, D. R. (2004). Landscape and regional impacts of hurricanes in Puerto Rico. Ecol. Monogr. 74 (2), 335–352. doi:10.1890/02-4057
FEMA, (2018). 2017 hurricane season FEMA after-action report . Washington, DC: Federal Emergency Management Agency .
Gill, J. C., and Malamud, B. D. (2014). Reviewing and visualizing the interactions of natural hazards. Rev. Geophys. 52 (4), 680–722. doi:10.1002/2013rg000445
Hain, A., Zaghi, A., and Padgett, J. (2022). “Data collection of damage to Puerto Rico's infrastructure due to Hurricane Maria and the 2019-2020 Puerto Rico earthquakes - mission 2,” in RAPID: Multi-hazard damage to Puerto Rico’s infrastructure - investigation of the interaction of hurricane and earthquakes . version 1 (Austin, TX: Natural Hazards Engineering Research Infrastructure: Cyberinfrastructure (DesignSafe) 2020–2025 ). Available at: https://www.nsf.gov/awardsearch/showAward?AWD_ID=2022469 . doi:10.17603/ds2-1mny-a490
Havidan, R. (1997). A socioeconomic analysis of hurricanes in Puerto Rico: An overview of disaster mitigation and preparedness. Hurricanes 1997, 121–143. doi:10.1007/978-3-642-60672-4_7
Hayes, G. P., McNamara, D. E., Seidman, L., and Roger, J. (2014). Quantifying potential earthquake and tsunami hazard in the Lesser Antilles subduction zone of the Caribbean region. Geophys. J. Int. 196 (1), 510–521. doi:10.1093/gji/ggt385
Imani, R., Mosqueda, G., and Bruneau, M. (2015). Finite element simulation of concrete-filled double-skin tube columns subjected to postearthquake fires. J. Struct. Eng. 141 (12), 04015055. doi:10.1061/(asce)st.1943-541x.0001301
Jaimes, M. A., Reinoso, E., and Esteva, L. (2015). Risk analysis for structures exposed to several multi-hazard sources. J. Earthq. Eng. 19 (2), 297–312. doi:10.1080/13632469.2014.962673
Jansma, P. E., Mattioli, G. S., Lopez, A., DeMets, C., Dixon, T. H., Mann, P., et al. (2000). Neotectonics of Puerto Rico and the Virgin Islands, northeastern Caribbean, from GPS geodesy. Tectonics 19 (6), 1021–1037. doi:10.1029/1999tc001170
Kameshwar, S., and Padgett, J. E. (2014). Multi-hazard risk assessment of highway bridges subjected to earthquake and hurricane hazards. Eng. Struct. 78, 154–166. doi:10.1016/j.engstruct.2014.05.016
Keller, W. J., and Pessiki, S. (2015). Effect of earthquake-induced damage on the sidesway response of steel moment-frame buildings during fire exposure. Earthq. Spectra 31 (1), 273–292. doi:10.1193/123112eqs362m
Kumar, R., and Gardoni, P. (2014). Effect of seismic degradation on the fragility of reinforced concrete bridges. Eng. Struct. 79, 267–275. doi:10.1016/j.engstruct.2014.08.019
Li, Y., Ahuja, A., and Padgett, J. E. (2012). Review of methods to assess, design for, and mitigate multiple hazards. J. Perform. Constr. Facil. 26 (1), 104–117. doi:10.1061/(asce)cf.1943-5509.0000279
Liu, W., Song, Z., and Ouyang, M. (2020). Lifecycle operational resilience assessment of urban water distribution networks. Reliab. Eng. Syst. Saf. 198, 106859. doi:10.1016/j.ress.2020.106859Get
Lloréns, H. (2018). Ruin Nation: In Puerto Rico, Hurricane Maria laid bare the results of a long-term crisis created by dispossession, migration, and economic predation. NACLA Rep. Am. 50 (2), 154–159. doi:10.1080/10714839.2018.1479468
López-Marrero, T., Heartsill-Scalley, T., Carlos, F-L-G-R., Escalera-Garcia, I. A., and Echevarria-Ramos, M. (2019). Broadening our understanding of hurricanes and forests on the caribbean island of Puerto Rico: Where and what should we study now? Forests 10 (9), 710. doi:10.3390/f10090710
Maniglio, M., Balomenos, G. P., Padgett, J. E., and Cimellaro, G. P. (2021). Parameterized coastal fragilities and their application to aging port structures subjected to surge and wave. Eng. Struct. 237, 112235. doi:10.1016/j.engstruct.2021.112235
Maria, H. (2017). PhotoSkyView. Hurricane Maria - the main bridge of Guayanilla after 50 Days (huracán Maria) - phantom 4Pro. https://www.youtube.com/watch?v=XHTrmqMBkDk&list=PLLvcsDpvAIDjPaWbKuWRKRFtYGtPqlILy&index=7 .
Miranda, E., Acosta Vera, A., Aponte, L., Archbold, J., Cortes, M., Du, A., et al. StEER - 7 jan. 2020 Puerto Rico Mw 6.4 earthquake: Preliminary virtual reconnaissance report (PVRR). 2020.
Ouyang, M., Dueñas-Osorio, L., and Min, X. (2012). A three-stage resilience analysis framework for urban infrastructure systems. Struct. Saf. 36, 23–31. doi:10.1016/j.strusafe.2011.12.004
Padgett, J. E., and Kameshwar, S. (2016). “Supporting life cycle management of bridges through multi-hazard reliability and risk assessment,” in Multi-hazard approaches to civil infrastructure engineering (Berlin, Germany: Springer ), 41–58.
Pasch, R. J., Penny, A. B., and Berg, R. (2017). Hurricane Maria (AL152017) . United States: National Hurricane Center .
Rathje, E., Dawson, C., Padgett, J. E., Pinelli, J-P., Stanzione, D., Adair, A., et al. (2017). DesignSafe: A new cyberinfrastructure for natural hazards engineering . Reston, VA: American Society of Civil Engineers .
Raussen, S., and Lykke-Andersen, H. (2009). “Micro plate tectonics in the Virgin Islands Basin, north eastern Caribbean,” in AGU Fall Meeting Abstracts , Chicago, IL , 12-16, December, 2022 .
Roy, T., and Matsagar, V. (2021). Multi-hazard analysis and design of structures: Status and research trends. Struct. Infrastructure Eng. 2021, 1–30. doi:10.1080/15732479.2021.1987481
Sanchez, R. (2020). For Puerto Rico’s earthquake families, trauma continues thousands of miles from home . Georgia, United States: CNN .
Shiraki, N., Shinozuka, M., Moore, J. E., Chang, S. E., Kameda, H., and Tanaka, S. (2007). System risk curves: Probabilistic performance scenarios for highway networks subject to earthquake damage. J. Infrastructure Syst. 13 (1), 43–54. doi:10.1061/(asce)1076-0342(2007)13:1(43)
Silva-Tulla, F., Pando, M., Soto, A., Morales, A., Pradel, D., Inci, G., et al. (2018). Geotechnical impacts of hurricane Maria in Puerto Rico . Virginia, United States: ASCE . Report No GEER-057.
Tillman, B. F. (2009). Measuring globalization's influence on the cultural landscape: Spatial succession in the plaza of Ponce, Puerto Rico. Southeast. Geogr. 49 (4), 340–353. doi:10.1353/sgo.0.0056
USGS, M. (2020). 6.4 - 8km S of indios, Puerto Rico. Available at: https://earthquake.usgs.gov/earthquakes/eventpage/us70006vll/map?shakemap-code=us70006vll&shakemap-source=us&shakemap-pga=true&shakemap-stations=true&shakemap-mmi-contours=false .
van de Lindt, J. W., Ellingwood, B. R., McAllister, T., Gardoni, P., Cox, D. T., Cutler, H., et al. (2015). “Computational environment for modeling and enhancing community resilience: Introducing the center for risk-based community resilience planning,” in Second International Conference on Performance-based and Lifecycle Structural Engineering (PLSE 2015) , Brisbane, AU , December 9-11, 2015 .
Van Der Elst, N., Hardebeck, J. L., and Michael, A. J. (2020). Potential duration of aftershocks of the 2020 southwestern Puerto Rico earthquake . US Geological Survey: US Geological Survey , 2331–1258.
Vičič, B., Momeni, S., Borghi, A., Lomax, A., and Aoudia, A. (2022). The 2019–2020 Southwest Puerto Rico earthquake sequence: Seismicity and faulting. Seismol. Soc. Am. 93 (2A), 533–543. doi:10.1785/0220210113
Vishnu, N., and Padgett, J. E. (2020). Interaction of life-cycle phases in a probabilistic life-cycle framework for civil infrastructure system sustainability. Sustain. Resilient Infrastructure 5 (5), 289–310. doi:10.1080/23789689.2019.1574514
Wakeman, T. H. (2013). University Transportation Research Center—Region 2 . Washington, DC: University Transportation Research Center, US Department of Transportation . 49997-56-24. Available at: https://rosap.ntl.bts.gov/view/dot/27004 .
Wang, Z., Dueñas-Osorio, L., and Padgett, J. E. (2014). Influence of scour effects on the seismic response of reinforced concrete bridges. Eng. Struct. 76, 202–214. doi:10.1016/j.engstruct.2014.06.026
Wei, L., Hu, K., Hu, X., Wu, C., and Zhang, X. (2022). Quantitative multi-hazard risk assessment to buildings in the Jiuzhaigou valley, a world natural heritage site in Western China. Geomatics, Nat. Hazards Risk 13 (1), 193–221. doi:10.1080/19475705.2021.2004244
Yang, D. Y., and Frangopol, D. M. (2019). Life-cycle management of deteriorating civil infrastructure considering resilience to lifetime hazards: A general approach based on renewal-reward processes. Reliab. Eng. Syst. Saf. 183, 197–212. doi:10.1016/j.ress.2018.11.016
Zaghi, A. E., Padgett, J. E., Bruneau, M., Barbato, M., Li, Y., Mitrani-Reiser, J., et al. (2016). Establishing common nomenclature, characterizing the problem, and identifying future opportunities in multihazard design. J. Struct. Eng. 142 (12), H2516001. doi:10.1061/(asce)st.1943-541x.0001586
Zaghi, A. P., Hain, J., Tafur, A., Lanning, A., and Grilliot, A., (2021). “Data collection of damage to Puerto Rico's infrastructure due to Hurricane Maria and the 2019-2020 Puerto Rico earthquakes,” in RAPID: Multi-hazard damage to Puerto Rico’s infrastructure - investigation of the interaction of hurricane and earthquakes . (Austin, TX: Natural Hazards Engineering Research Infrastructure: Cyberinfrastructure (DesignSafe) 2020–2025 ). Available at: https://www.nsf.gov/awardsearch/showAward?AWD_ID=2022469 . version 1. doi:10.17603/ds2-jj5g-yg64
Keywords: multihazard, reconnaissance, earthquakes, Hurricane Maria, cascading effects
Citation: Hain A, Zaghi AE, Padgett JE and Tafur A (2023) Case studies of multihazard damage: Investigation of the interaction of Hurricane Maria and the January 2020 earthquake sequence in Puerto Rico. Front. Built Environ. 9:1128573. doi: 10.3389/fbuil.2023.1128573
Received: 20 December 2022; Accepted: 27 January 2023; Published: 13 February 2023.
Reviewed by:
Copyright © 2023 Hain, Zaghi, Padgett and Tafur. This is an open-access article distributed under the terms of the Creative Commons Attribution License (CC BY). The use, distribution or reproduction in other forums is permitted, provided the original author(s) and the copyright owner(s) are credited and that the original publication in this journal is cited, in accordance with accepted academic practice. No use, distribution or reproduction is permitted which does not comply with these terms.
*Correspondence: Alexandra Hain, [email protected]
This article is part of the Research Topic
Technology Transfer from the Natural Hazards Engineering Research Infrastructure (NHERI)

An official website of the United States government
The .gov means it’s official. Federal government websites often end in .gov or .mil. Before sharing sensitive information, make sure you’re on a federal government site.
The site is secure. The https:// ensures that you are connecting to the official website and that any information you provide is encrypted and transmitted securely.
- Publications
- Account settings
Preview improvements coming to the PMC website in October 2024. Learn More or Try it out now .
- Advanced Search
- Journal List
- Springer Nature - PMC COVID-19 Collection

Post-earthquake damage classification and assessment: case study of the residential buildings after the M w = 5 earthquake in Mila city, Northeast Algeria on August 7, 2020
Hamidatou mouloud.
1 Research Center in Astronomy, Astrophysics and Geophysics, BP 63, 16340 Bouzaréah, Algiers Algeria
Amar Chaker
2 The Engineering Mechanics Institute, American Society of Civil Engineers, Reston, USA
Hallal Nassim
Saad lebdioui.
3 Faculty of Technology, University of August 20, 1955-Skikda, P. O. Box 26, 21000 Skikda, Algeria
Hugo Rodrigues
4 Civil Engineering Department, University of Aveiro, Campus Universitário de Santiago, RISCO, 3810-193 Aveiro, Portugal
Matthew R. Agius
5 Dipartiment tal-Ġeoxjenza, Fakultà tax-Xjenza, L-Università ta’ Malta, Msida, Malta
Associated Data
All data generated or analyzed during this study are included in this article.
On August 7th, 2020, a magnitude Mw = 5.0 earthquake shook 5 km north of Mila city center, northeast of Algeria, causing substantial damage directly to structures, and indirectly from induced impacts of landslides and rock falls, ultimately disrupt to everyday civilian life. Given the recent significant seismic occurrences in the region, a detailed and comprehensive examination and assessment of post-earthquake damage is critical to Algeria. This is primarily because masonry, concrete, and colonial-era structures are sensitive to horizontal motions caused by seismic waves, and because masonry and concrete structures constitute a substantial portion of today’s Algeria's build environment. We present a post-earthquake investigation of the Mila earthquake, starting from the earthquake source, and a catalogue of buildings type, damage categorization, and failure patterns of residential structures in Mila's historic old town, where colonial-era brick buildings prevail. We find that structures that represent notable architectural achievements were severely damaged as a result of the earthquake. Data acquired during the immediate post-earthquake analysis was also evaluated and discussed. The graphical representations of the damages are detailed and complemented by photos. This seismic event has shown the fragility of Algeria's building stock, which must be addressed properly in future years. This study reports on an overall estimate of residential buildings in Mila's lower city, as well as an evaluation of the seismic vulnerability of three neighborhood towns (El-Kherba, Grareme-Gouga, and Azzeba). A generic database for graphical surveys and geometric research was developed and implemented making it possible to evaluate the shear strength on-site. The broad observations, collated data, and consequences were then loaded into the 3Muri structural verification program. Nonlinear static analysis was conducted to analyze probable failure paths and compare the real damage to the software results.
Introduction
A seismic event is a rare natural occurrence that may induce enormous costs and consequences for structures, the environment, human life, and society. On August 7, 2020, at 06:15:37 UTC, an earthquake of a moderate magnitude Mw = 5.0 (Boulahia, 2022 ) and intensity VI on the European Macroseismic scale EMS-98 struck the Mila metropolitan region (Eurocode 8 2004a , b ). It was followed at 08:12:43 UTC by the strongest aftershock earthquake, with a magnitude Mw = 4.8 (Boulahia, 2022 ), and intensity of V. The primary earthquake destroyed a substantial number of structures in three areas (El-Kherba, Grareme-Gouga, and Azzeba), including both residential and public buildings. The vast majority of structures built following Algeria's earthquake code (RPA, Règulement Para-sismique Algerien 2003 ) were either undamaged or only minimally impacted by other correlated hazards, like landslides. However, most of the city (upper and lower areas) was severely affected since structures were constructed without earthquake design concerns and on unstable soils, besides, an important part of the construction was built without official approval. The destruction of buildings is considerable; over 1040 structures were affected, many of which were seriously destroyed (Fig. 1 ). At the end of the year, another moderate earthquake ( Mw = 5.2) struck Northeast Algeria, with Skikda as the epicenter, about 60 km from Mila. The seismic event triggered minor secondary impacts to already damaged constructions.

Illustration of typical structure damage following the Mila earthquake
Seismic vulnerability assessment for past earthquake in Northeast Algeria has been relatively newly initiated, starting on 1992 by Farsi and Belazougui ( 1992 ). The current work consists of regional and local studies, mainly based on seismic vulnerability assessments by (Bechtoula and Ousalem 2005 ; Laouami et al. 2006 ; Harbi et al. 2007a , 2007b ; Belazougui 2008 ; Hellel et al. 2010 ; Meslem et al. 2012 ; Mehani et al. 2013 ; Boukri et al. 2013 ; Remki and Benouar 2014 ; Hamidatou et al. 2017 ; Chimouni et al. 2018 ; Allali et al. 2018 ; Hichem et al. 2019 ; Akkouche 2019 ; Amari et al. 2020 ). We know that the first major hazard that threatens the Mila region is landslide, in this case several studies have assessed landslides’ risk as well as earthquake-triggered landslides’ risk in the region (Marmi et al. 2008 ; Atmania et al. 2010 ; Guemache et al. 2010 ; Semmane et al. 2012 ; Merghadi et al. 2018 ; Benfedda et al. 2021 ; Tebbouche et al. 2022 ; Smail et al. 2022 ; Bounemeur et al. 2022 ; Medhat et al. 2022 ).
Algeria has a vast number of modern constructions made of reinforced concrete (RC), masonry, and colonial 19th and 20th-century style buildings. Assuming that most of the ostensibly "strategic" projects of cultural and historical value are constructed of masonry, this suggests that old masonry constructions must be evaluated and repaired according to the highest standards (Atalić et al. 2019 ; Ortega et al. 2019 ; Rodríguez et al. 2019 ; Stepinac et al. 2020 ; ARES 2021 ). Pushover analysis, also known as nonlinear static evaluation, is crucial and is suggested as a reference technique in Eurocode 8–3 for such cases. Even though the engineering community has made significant advances in comprehending seismic occurrences and their consequences, there are still many unknowns and uncertainties. Earthquake engineering requires a better description of seismic motions and new robust tools for analyzing buildings and assessing seismic hazards and risks. In the previous several decades, structural standards have been carefully researched, developed, and improved to the point that they now allow for designing new types of structures while preventing major damage to human life. It is also necessary to have the ability to evaluate the response of structures to earthquake ground shaking. The seismic risk of existing masonry constructions is difficult to assess and necessitates specialized technical knowledge (Lourenco and Karanikoloudis 2019 ). Post-earthquake condition evaluation may be described as the process of determining the safety and usability of a structure following a seismic event. Many factors influence structural seismic performance, including the number of floors, roof shape, age of construction, building materials, the geometry of the structure, stiffness, strength, ductility properties, soil conditions, and seismic event characteristics. Information from multiple disciplines, such as tectonics, geophysics, seismology, geology, and civil engineering including geotechnical and structural engineering, mathematics, or applied statistics, is required.
Afterward the earthquake, the first to respond to a catastrophe were civil engineers who led and coordinated the entire organization of building assessment and construction damage analysis. Several similar post-earthquake assessment procedures are used internationally (Yavari et al. 2010 ; Marshall et al. 2013 ; Didier et al. 2017 ). In the first week after Mila earthquake, a large number of constructions were examined, with a rapid post-earthquake assessment. The most endangered buildings in the city’s center were the ones under cultural heritage protection. The aim of a rapid assessment of structures is to determine the degree of damage to buildings concerning the protection of life and property, that is, to determine if the structures are usable, temporarily unusable or unusable. Emerging technological advances allow the usage of artificial intelligence in the post-earthquake assessment process in the form of machine learning methods for more efficient and precise results (Bialas et al. 2016 ; Zhang et al. 2018 ; Kim et al. 2020 ; Natio et al. 2020 ; Stepinac and Gasparuvic 2020 ).
Mila is a rural area separated into three zones: Zone A, Zone B, and Zone C (Fig. 2 ). Mila's most ancient architectural districts are found in Zone A, which is defined by packed blocks of structure masonry, brick, or a mix of materials. The majority of structures are made out of solid longitudinal and transverse walls, brick ceiling vaults, or timber ceiling beams with reinforced concrete roofs. Some schools, commercial structures, residential and government structures, cultural institutions, and monuments are located in Zone A, and are either components of a historical city structure or stand as lone landmark buildings. The earthquake-caused landslides severely damaged 10% of the structures in Zone A. Zone B is made up of a wide range of urban designs and includes a high number of structures. The landslide zone triggered by the seismic event is Zone C, and some buildings in this area have been significantly damaged. The seismic event occurred throughout the COVID-19 pandemic lockdown and caused a critical interruption to the social restrictions adapted at that time. The research focuses on the post-earthquake investigation, damage categorization, and failure patterns of masonry residential projects in the Mila region. We present primary data acquired by onsite inspections and provide an updated and broader insight to the seismic hazard of the Mila region. We also run 3D modelling to better understand the response of building in similar conditions.

Protected areas A, B, and C, as well as the region of the analysis within the Mila (yellow dashed line)
In fact, the main shock triggered many large landslides, of which, the traces are traceable and the impact on individual houses is significant. The most important one was observed in the El Kherba region (Zone C in Fig. 2 ), a catastrophic one which caused damage to residential structures. A substantial number of structures and infrastructures in the landslide's neighbourhood has been severely damaged. According to this study in post-earthquake categorization, about 10% of structures were damaged in zone A, 15% in zone B, and 61% in zone C. Exhibiting the important effect of the major earthquake-triggered landslide in zone C.
According to Benfedda et al. ( 2021 ), six landslide zones have been identified using InSAR analysis of two Sentinel-1A images, taken before and after the August 7 event main shock. The landslides were located along a 22 km long and 6.5 km wide corridor oriented NE-SW. Furthermore, Medhat et al. ( 2022 ) detected tow landslide zone in far-separated regions, Kherba city and Grarem Gouga city using the 2D decomposition MT-InSAR approach to detect the deformation velocity before the landslide activity, retrieving displacement velocity rates up to ~ 50 mm/year. Two regions were located at 12 km apart, indicating slow motion rather than fast movement along the damaged area. In addition, Halla et al. ( 2022 under publication) three large landslides have been observed within 13 km radius. The three landslides are located South, Southwest and Southeast. The most important one is that of the El-Kherba district: the Western extension of Mila city. The second landslide is located just near the epicenter within a radius of 5 km, East of Grrarem-Gouga village. The last landslide is the least important. It is located in the Azzeba village, situated Southeast of Mila city.
A thorough and more thorough post-earthquake damage assessment is required in light of the recent devastating earthquakes in Algeria. This is particularly crucial for Algeria since the bulk of the country's buildings are made of masonry, which makes them extremely sensitive to earthquake-induced horizontal movements. An exhaustive assessment of a residential structure in Mila's lower town is given in this paper. Geometric surveys and visual inspections were both part of a comprehensive program that was developed and executed. This earthquake brought to light Mila's vulnerable building stock, which has to be mitigated as effectively as possible going forward. To minimize earthquake losses, determining the characteristics of susceptibility and evaluating the seismic performance of existing structures are crucial (Endo et al. 2017 ; Casapulla et al. 2018 ; Ortega et al. 2018 ; Valente and Milani 2019 ; Hichem et al. 2019 ; Grillanda et al. 2020 ).
Historical seismicity and the seismic sequence in Mila
Seismicity in algeria's northeast.
Many seismic events have shaken Algeria's Northeast in the past. The strongest earthquakes were the tsunamigenic earthquakes that struck the city of Jijel (previously Djidjelli) on 21 and 22 August 1856, with an intensity of X on the EMS scale, and affected Djidjelli and the nearby region (Harbi et al. 2011 ). According to ancient sources, the earthquake generated a tsunami and caused extensive damage in the city, with more than 30 people killed and many collapsed structures. Figure 3 is a map of major earthquakes in northern Algeria with their surface magnitudes Ms . Only a few significant earthquakes have happened in Algeria's northeastern region in more than a century. As a result, public awareness and readiness were at an all-time low. Though authorities and scientists have warned for years about the repercussions of a catastrophic earthquake and about the importance of planning for a swift response after an earthquake (vulnerability assessment, rescue and care of people, damage assessments, etc.…), preparedness actions have been limited (Atalić et al. 2019 ; Stepinac et al. 2020 ).

Strong earthquakes in Algeria's Northeast and surrounding regions in the previous century from 1900 to 2021, the red square indicates the Mila region (Hamidatou et al. 2017 , 2019 , 2021 )
Mila is located in the NW of the Constantine basin, which, with its tectonic configuration, is the key cause of earthquakes in the zone (Durand 1969 ; Raoult 1974 ; Vila 1980 ; Coiffait 1992 ). The 1985 Constantine earthquake (Ms = 6.0, Ousadou et al. 2012 ), the worst seismic event registered in the area, triggered substantial damage in the city and was felt in the Mila area. According to historical records, the earthquake produced extensive damage to constructions (108 structures were damaged) and caused two fatalities and injured 10 inhabitants (Bounif et al. 1987 ). The 1985 earthquake was a watershed moment in Northeast Algeria’s development and city planning. However, since strong seismic events occur across relatively extended time periods, the lessons of the past are easily forgotten. Even though 50 percent of the North Algerian region is vulnerable to severe seismic shaking, and 70% of the population lives in this region, the public’s risk awareness is low.
The seismic sequence in Mila on July–August 2020
In the period including July and August 2020, the Mila region witnessed a seismic sequence that was marked mainly by the appearance of three important shocks. Recently, Benfedda et al. ( 2021 ) studied the main events of the July–August 2020 Mila seismic sequence, including, respectively, the events on July 17 at 08:12 UTC (Mw 4.6), August 7 at 06:15 UTC (Mw 4.8) and at 11:13 UTC (Mw 4.4). More recently, Boulahia ( 2022 ) examined the first shock of July 17, 2020 at 08:12 UTC of Mw 4.8, located 1 km North of Sidi Merouane (near Grarem-Gouga area), a second shock on the August 7, 2020, at 06:15:37 UTC, where he stated a Mw magnitude of 5.0, and a third shock at 11:13:27 UTC of a Mw 4.5 (Boulahia 2022 ). The main shock (the second one) had an intensity of VI according to the EMS-98 scale, it also triggered a spectacular landslide in the El Kherba region. This landslide caused significant damage to individual buildings (Fig. 4 ).

Preliminary Earthquake Intensity Map (left) from the earthquake of August 7, 2020, at 6:15:37 (UTC) compared with predicted peak ground accelerations (right) for a return period of 475 years (Hamidatou et al. 2021 ), the red square indicates the Mila region
Benfedda et al. ( 2021 ) performed a waveform inversion of the accelerograms to calculate the seismic moment, moment magnitude, and focal mechanisms of the three main seismic events:
- The July 17th, 2020, Mo = 1.019E + 16 Nm, Mw = 4.6, h = 5 km.
- The August 7th main shock, Mo = 1.794E + 16 Nm, Mw = 4.8, h = 8 km.
- The August 7th aftershock, Mo = 4.653E + 15 Nm, Mw = 4.4, h = 12 km.
They determined focal mechanisms generated a pure strike-slip solution for the three events with nodal plans oriented NE-SW and NE-SW and a pressure axis oriented N-S.
In addition Boulahia ( 2022 ) used an Empirical Green’s Function (EGF) method to derive the Relative Source Time Functions (RSTF’s) and high-resolution relocation to active structures and analyzed the spatiotemporal behavior and mechanics of the sequence. They managed to separate the initial seismic cloud into two densely concentrated spatial clusters of strongly correlated events, and were able to detect components of directivity toward the southeast for the shock (Mw 4.8) and directivity toward the northeast for the mainshock (Mw 5.0).
- The July 17th, 2020, Mo = 2.14 × 1016 N.m, Mw = 4.8.
- The August 7th main shock, Mo = 3.14 × 1016 N.m, Mw = 5.0.
- The August 7th aftershock, Mo = 0.67 × 1016 Nm, Mw = 4.5.
They relocate over 981 events from the sequence, with structures matching moment tensor solutions and focal mechanisms indicating predominantly right- and left-lateral strike-slip ruptures. The results reveal orthogonal conjugate structures—one trending ~ N65W and dipping 80°SW, and one trending ~ N28E, 70°NW-dipping fault plane. The earthquakes evolved in two phases, with a spatiotemporal migration of epicenters from the NW–SE fault plane to the NE-SW fault at a rate consistent with pore fluid diffusion (Boulahia 2022 ).
Furthermore, Benfedda et al. ( 2021 ) recorded the peak ground accelerations (PGAs) of the three major events in Mila by the local network. The Beni-Haroun station recorded the maximum accelerations for all events. The Beni-Haroun huge dam reservoir is just a few meters away from the BHAR station, which is situated in a free field and on hard rock on the NW side. The high values of ground motion of these relatively small earthquakes are explained by the near field and shallow depth of the seismic events. Hard rock often records such high acceleration values quite near to the hypocenters (Laouami and Slimani 2018 ).
The earthquake's epicenter was located at latitude 6.28 N and longitude 36.54E. The epicenter was located around 13 km Southwest of Mila center, in the Hamala area, at a depth of 7 km. The strongest aftershock occurred at 8:12:43 UTC, with an Mw magnitude of 4.8 (Boulahia 2022 ) and EMS-98 intensity V. The epicenter of this aftershock was about 10 km Southwest of Mila's center, at a depth of 10 km. On the same day, five aftershocks with Mw magnitudes larger than 3.0 and EMS-98 intensity V were observed. Following that, the city of Mila and its surroundings have been hit by a series of mild and medium aftershock earthquakes (Fig. 5 ).

Earthquakes with a magnitude more than 2.0 ( Mw ) occurred in Mila between August 7 and October 30, 2020
Even though the seismic event was of moderate magnitude, it caused a large amount of measurable damage. Seismic risk assessment in urban areas and building vulnerability are usually misread, therefore the majority of community media (and a section of the technical community) have consistently reported on the disparity between the magnitude of the earthquake and the degree of damage. Assuming that a thorough and complete seismic risk assessment for the city of Mila has not been conducted, such reporting is not unusual. Given that the PGA for the historic core of Mila, which was the subject of prior studies, was about 0.19 g, and that most of the structures are quite vulnerable, widespread damage should have been predicted (Hamidatou and Sbrtai 2016 , 2017 ; Hamidatou et al. 2019 ; 2021 ). The primary earthquake destroyed a substantial number of old urban structures, including residential buildings, administrative offices, colleges, and public institutions.
Procedure for immediate response and post-earthquake evaluation
The seismic event in Mila occurred during the strong restrictions imposed following the first wave of the COVID-19 pandemic, which imposed extra constraints on rescuer and researcher operations. Immediately following the earthquake, a multidisciplinary post-seismic disaster management team visited the Mila City Crisis Management Agency to examine the damaged state of the constructions and the possibility of their continued use in a timely manner. The Civil Protection Center was activated, and researchers, specialists, and experts from research centers and universities, as well as the CRAAG, were hired to organize work related to field condition assessment, installation of the seismic network and Global Positioning Systems (GPS), geological and geophysical field missions, rapid engineering assessment training, and the organization and development of an information system for dealing with disasters. All activities were conducted in partnership with the Ministry of the Interior's Civil Protection Directorate and the City of Mila's Civil Protection Authority.
Based on Italian results, a prototype technique for post-earthquake damage and useability study was proposed (Baggio et al. 2007 ) and EMS-98 (EMS 1998 ), because knowledge of seismic occurrences in northern Algeria is low, vigilance for such events and immediate actions was also poor. Until now, data on the number of structures, floor layouts, cross-sections, building materials, or function at the time of the earthquake were not available. The post-earthquake damage analysis includes a quick visual evaluation of each structural system, an indication of the degree of damage, and the categorization of the structure into each of six sections:
- CN1 (dark red color): Not feasible due to external hazards—The construction is dangerous due to the likelihood of major portions of a nearby structure collapsing. It is advised not to remain in such facilities given the considerable number of aftershocks.
- CN2 (red color): Unusable owing to damage—The structure is dangerous due to substantial structural damage, collapse, and failure. The structure has reached the limit of its loadbearing capability and ductility and cannot be utilized in any way. That does not always mean that the building must be destroyed.
- PCN1 (dark yellow color): Possibly unusable—Full assessment required—The structure has a fair extent of the damage but no risk of collapse. The loadbearing capacity has been partly reduced. A shorter visit to the structure is conceivable, and a structural engineer should provide suggestions for future repair work.
- PCN2 (yellow color): Temporarily unusable- Emergency rehabilitation measures required—The structure has some damage with no probability of collapse, but cannot be utilized owing to the possibility of failure of some structural components. The structural engineer is aware of emergency response methods and must give instructions to users. The structure, or a portion of it, is inoperable until the safeguards are put in place. Provisional usability may apply to elements of the structure (components) only.
- CU1 (dark green color): Usable without limitations—There is no damage or only minor damage that does not jeopardize the structure's load-bearing ability and usage.
- CU2 (green color): Consider Protective Measures.—Except for some elements where there is an immediate risk to a portion of the structure, the structure can be used. The building evaluator can grant authorization for sthe risk to be removed and advise the occupants to impose temporary residential limitations on specific portions of the structure. The structure can be used freely after the risk has been removed.
After Day 1, Class CU2 was used in the procedure because non-structural components of the structures were damaged and may endanger passers-by and members of the public. It was essential to eliminate these components as quickly and efficiently as possible. The structure was safe to use once the non-structural damaged components were removed.
The building typologies, structural damage, and failure patterns caused by the earthquake or landslide in the Mila city center area are depicted and discussed in the following sections. The brick structures that make up a considerable proportion of the city center and the surrounding area are given special attention. This study focuses on the typical damage and disaster to residential structures. Significant advancement has recently been achieved in understanding the seismic behavior of masonry buildings and analysis during seismic occurrences (Binda et al. 2000 ; Ortega et al. 2017 ; Vlachakis et al. 2020 ). It is hoped that the current data will positively contribute to further development in this field.
Typology of constructions in Mila
Mila's buildings typically consist of roughly 91,000 residential constructions and 5,000 non-residential constructions, according to the Algeria Population and Housing Census 2008, provided by the National Office of Statistics of Algeria. More than 20% of the construction stock is over 40 years old. The use of traditional materials and building techniques, like masonry and timber, is a defining feature of such older Mila structures. Most of the people in Mila province, particularly in the cities, work and reside in colonial constructions, especially in Mila's city core.
Throughout the city's and the surrounding areas’ history, numerous types of structures have been constructed depending on the advancement of construction technology, understanding of soil qualities, and urban planning, including urban protection measures and the demand for building areas. Knowing when a set of buildings were built provides a reasonable estimate of their seismic strength following the 1985 Constantine earthquake. Most of the structures in Mila's old city center are concrete and masonry constructions with timber floors and roofs. A single-story typical height varied from 2.5 to 3.7 m.
They followed RPA norms from 1996 to 2003, and after 2003, they followed RPA regulations (RPA 2003 ). As a result, many structures were built without any proper lateral force resisting system before any seismic regulations were implemented. In terms of floor systems, timber in older houses and reinforced concrete in later constructions are the most common options. Timber floors are more flexible than rigid concrete slabs. Because the connections between walls and floors are typically weak, this makes walls more susceptible to potential out-of-plane failure mechanisms.
New structures with four or more stories have been built in the city throughout the previous two decades. They were designed to withstand earthquakes. A mix of historic individual structures and new apartment towers distinguishes the urban area. Most single-family houses in Mila's immediate vicinity are one- or two-story brick structures. Contained masonry with reinforced concrete floor constructions is more recent than unreinforced stone masonry constructions with wood or reinforced concrete floors. About 17 percent of dwelling units in Mila were built before the first seismic code was enacted (1982), Fifteen percent of housing units were designed following the first seismic code (1962–1996), and 29% were built using the enhanced seismic code (Table (Table1). 1 ). The RPA 2003 code was used to design housing units built after 2003.
The distribution of dwelling units by year of construction, based on the most recent census data (2008)
Mila's historical city compound is a conservation area under the Act on Culture and Heritage Conservation and Maintenance. Zone A and Zone B are the two zones that make up the region (Fig. 6 ). The oldest and most architecturally key areas of Mila are in Zone A, which is the subject of this study. Both locations, however, have architectural and historic landmarks and are characterized by densely packed blocks of constructions using stone brick or a mix of materials. Many schools, businesses, residential and government structures, social organizations, and mosques are in Zone A and are conserved as part of a historical city structure or as separate historical sites, although they are not the subject of this research. Zone B contains the remaining parts of Mila's historic urban complex. It contains a wide range of urban forms as well as a considerable number of historically significant structures.

Heritage-protected zones in Mila (Zone A, Zone B, and Zone C). The yellow dashed line indicates the study's observation area
Once most of the buildings are constructed as part of bigger blocks, their sides are frequently the width of a leaf. The same building strategy was utilized even when the structures were built inside the blocks, i.e., as freestanding units, and these freestanding structures were severely damaged.
The inadequate connections between walls and floors are also observed, and since the floor structure is primarily wooden, the structures lack the so-called box-type behaviour. Timber flooring prevails despite the presence of multiple composite wooden concrete compound buildings. Because group of unreinforced masonry (URM) buildings are prone to damage from seismic excitations (Palazai et al. 2022 ), the damage was observed in a large number of structures following the Mila earthquake. The typical roof structure is a king or queen post truss; however, there are many distinct types of integrated timber roof constructions (Fig. 7 ). Due to rehabilitation work, a concrete slab beneath the roof systems can be found in a limited number of structures. Even though tie rods have been used for many years over the world, they were not widely used in Mila. Timber-reinforced masonry was used in very few examples in the ancient city core. After 1980, numerous new concrete constructions were put within the old downtown's existing building blocks, a trend that still exists today. These structures were either unaffected by the earthquake or suffered only minor non-structural damage. Newer buildings, on the other hand, tended to have more stories and less interstory height than older buildings, which clearly influenced the seismic response of neighboring structures (Fig. 8 ).

Typical residential structure (schematic representation)

Insertion of new concrete constructions "within" existing blocks during the building process and at the end
The lack of maintenance was identified as a key factor in the condition of structures following the earthquake. Because many structures were poorly or never maintained, the masonry strength deteriorated with time, the connection between the masonry and the walls was degraded, and the seismic performance of such buildings worsened. Water infiltration damaged the characteristics of both masonry and wood elements in numerous cases.
Case study: Sidi Ghanem Mosque
We studied the Sidi Ghanem Mosque, a building located in historical Mila city (Figs. 9 and and10). 10 ). The Sidi Ghanem Mosque is the oldest mosque in Africa. Mila was once a Roman settlement. The Umayyad Arab forces arrived in 675 CE, about 59 AH. Under the direction of Abu al-Muhajir Dinar, they seized the city. That same year, it appears, the order was made to clear a site adjacent to a Christian Basilica and to build a mosque. The basilica had an abundance of building materials, stones, and columns that could be reused. The mosque, on the other hand, did not mirror the familiar Roman basilica or the Roman city street architecture, but it had an important meaning. Its 62 m-high minaret, for example, was constructed with 365 steps, representing the number of days in the year. The mosque of Abu al-Muhajir in Mila does not have a marked orientation. Here's a blueprint for the structure. It began as a mosque, but was later turned into a workshop and, finally, a hospital.

North façade view of the Sidi Ghanem Mosque

South façade view of the Sidi Ghanem Mosque
The Roman basilica was demolished to make way for the mosque to be rebuilt. The main construction is made of reclaimed materials from the old town. The reuse of Roman columns and marquees allowed the mosque to have more robust construction. The other materials used were full-size bricks made on-site. There are visible elements of a second-story building that the French-built to house the soldiers.
The state of disrepair of the Sidi Ghanem Mosque was worsened by the Mila earthquake. The study of the monument’s restoration, which began in 2019, focused in its first phase on "the state of the sites and emergency measures," in light of which emergency work was undertaken.
According to the Algerian seismic hazard map (Hamidatou and Sbartai 2017 ; Hamidatou et al. 2019 , 2021 ), for a return time of 475 years, the peak ground acceleration at the building site is 0.197 g. The building serves as an educational-cultural institution. Prior to the earthquake, the structure's state in terms of vertical loads was adequate and well maintained.
Residential building damage and common failure patterns
The earthquake severely damaged key architectural achievements and shattered Mila's historically identifiable city center. The vast majority of structures were constructed after the first mandated earthquake rules (i.e., during the 1980s) withstood the earthquake unscathed or with only minor damage. However, many structures in the historic district (Upper and Lower Town) were severely damaged. It is estimated that about 10% of the entire building stock was damaged in Zone A and more than 15% in Zone B—a total of 540 damaged buildings. However, in Zone C, 61% of the buildings were damaged, with a total of 743 building, i.e., more than the total of the damaged structures in Zones A and B gathered, exhibiting the substantial effect of the major earthquake-triggered landslide in zone C. (Table (Table2 2 ).
The number of damaged structures in Mila's protected historic urban center (Zones A and B)
According to the previously mentioned post-earthquake categorization (red or N, yellow or PN, green or U), of 136 damaged structures in zone A, 97 were green-tagged, 35 were yellow-tagged, and 4 were red-tagged, whereas in zone B, of 404 damage structure, 342 were green-tagged, 55 were yellow-tagged, and 7 were red-tagged. However, in Zone C, among the 743 damaged buildings, 515 were green-tagged, 137 were yellow-tagged, and 91 were red-tagged. In Fig. 11 , the residential structures with usability tags are depicted on a map of the historical city center, whereas Fig. 12 depicts a typical building block. In this block, 17 buildings were green-tagged (13 percent of all damaged buildings), 20 (17 percent) were yellow-tagged, and 3 (4 percent) were red-tagged.

A detail of Mila city's building usability rating

Post-earthquake damage assessment for one typical block of buildings
The historic downtown is made up of blocks of aggregated buildings, a damage map for each one has been prepared (Fig. 13 ). The goal was to gather information on the risk of individual blocks as well as a prospective seismic vulnerability assessment for certain sections of Mila. Although it was assumed that blocks further away from the epicenter would be less damaged, the map in Fig. 13 reveals a significant spread and random outcomes.

Average damage index for each block of buildings
According to our post-earthquake assessment analysis, each individual building in the block was assigned a number on the map. CU1 buildings were assigned an index of 0.5, CU2 buildings were assigned an index of 1, PCN1 and PCN2 buildings were assigned an index of 3, and CN1 and CN2 buildings were assigned an index of 5.
Ni: Number of structures in each calculation mesh assigned to a damage category.
Based on the information received; these figures are enough for a basic indicator of damage or average index can be computed for the entire block. For the block depicted in Fig. 12 , the damage index is computed as a weighted average depending on the number of individual structures, as 12 × 0 , 5 CU1 + 25 × 1 CU2 + 40 × 3 PCN1 + PCN2 + 9 × 5 CN1 + CN2 / 86 = 196 / 86 = 2 , 28 .
It should be emphasized that the damage information should be viewed with caution because the criteria of various engineers were often subjective and deviated from the evaluation guidelines.
The choice to consider PN1 and PN2 designations, as well as N1 and N2 with the same damage index is also justified. Although the results may not provide an entirely accurate image of the damage in the city, they do provide a reasonable indication of which areas are most vulnerable to earthquakes. The immediate post-earthquake evaluation revealed unique structural damage typologies. Non-structural components such as chimneys and ornamental elements on facades were found to be losing their structural resistance and stability on every structure in the city center. These factors resulted in further damage to the building exterior or structural damage to nearby structures, as well as water intrusion inside the premises. Secondly, the loss of structural strength and stability of structural components jeopardized the structural integrity of entire structures. Gable walls, masonry columns, portions of walls between or under windows, vaults, ceilings, and stairs are the most frequently encountered damaged elements. Some of these features also harmed roof systems, which frequently became unstable because of the collapse of individual load-bearing walls underneath them.
Individual buildings, particularly those within the blocks of buildings, suffered significant damage and had uncertain structural resistance and stability. Table Table3 3 shows the prevalence of observed masonry structure damages and load-deformation in Mila. The structural damage observed is discussed in the following section with graphical interpretations and images of the affected buildings. There are examples of in-plane, out-of-plane, and mixed damage or failure mechanisms.
Typical masonry structure damages and failure types after the Mila earthquake
Structural damage
Masonry is a well-known heterogeneous, composite material with low tensile strength and significant self-weight, making it difficult to sustain earthquake-induced stresses. Learning from past occurrences can help us design better new structures and make post-earthquake evaluations easier. The Mila earthquake caused damage to clay brick masonry constructions similar to those observed in Japan and Italy (Penna et al. 2014 ; Binda et al. 2000 ; De Luca et al. 2018 ) and Turkey after recent earthquakes (Karimzadeh et al. 2018 ; 2020 ). Most of the residential structures in Mila's old town were constructed with URM. To prevent masonry failure, proper detailing and strengthening should be employed. However, this was not always the case in older structures. Even though “zero mechanism” was frequently mentioned in many post-earthquake evaluations in African nations (i.e., the disintegration of the material) (Indirli et al. 2013 ), due to the construction type, i.e., solid brick structure, this mechanism was not detected during the Mila earthquake. The partial collapse or overturning of gable walls was one of the most typical failure types in Mila's residential structures. Gable walls are often just 9 cm thick. In addition to the attic gable walls, the parapet walls were frequently damaged, but to a lesser extent. The preceding part depicted the roof type, and it was observed that the connections between the timber framework and the walls were frequently insufficient or non-existent. Roof rafters and ridge beams frequently collide with gable walls, causing substantial damage and failure due to their weight and magnified accelerations at the gable's height. The same thing happens with the mezzanine wood structure (which rests on the longitudinal walls) and sidewalls—the wall and horizontal parts are not or are very poorly attached. Figure 14 depicts damage examples from the most prevalent gable wall collapses.

In-plane and out-of plane damage
Many buildings have an architectural design that includes protrusions for entrances or other floor plan irregularities. Cracks frequently emerge at the junction of orthogonal load-bearing walls in these areas; torsion ensues, which can compromise the building's overall stability. Because building entrances with stairs are widespread in these locations, the staircases and related load-bearing walls are frequently damaged. Figure 15 depicts one example.

Plan irregularities caused damage to the connecting components
Non-structural damages
The Mila earthquake caused the most damage to non-structural elements. Partition walls are typically composed of 9 cm-thick masonry but can be as thin as 3.7 cm without plaster. The degree of damage to partition walls ranges from minor plaster damage to severe collapse and failure (Fig. 16 ). Non-structural walls are sometimes built on the shorter side of the brick (shiner) and are likely to be destroyed after an earthquake. Although damage to partition walls may not result in a permanent reduction in structural strength and stability, it can nonetheless represent a risk to the building's performance and peoples’ safety. Many buildings in Mila have such walls that are exceedingly high and long. This makes them comparatively thin, and their collapse can endanger people. The massive damage to partition walls reflects a major economic loss as well. Certain institutions with huge floor areas have partition walls that have sustained so much damage that cost–benefit assessments have shown that repairing them is not profitable. The building approach was to stack partition walls on top of one other, transferring no load to the slab. If the first-floor wall is destroyed or lost, the weight of the second floor (and higher levels) will be transmitted to the first-floor slab.

Damage to partition walls
Conversion of non-residential spaces to residential spaces is a common adjustment to building spaces (such as basements, attics, or service rooms). Partition walls were frequently eliminated during such renovations, undesired intrusions in load-bearing walls are also common. It is often assumed that if a steel beam is installed on top of the opening to replace the load-bearing wall, nothing will happen to the structure. Elements of the roof structure are frequently removed when transforming an attic into a living area since they interfere with the intended usage (Fig. 17 ). Roof structures have been extensively modified during the conversion of attics to residential use. Timber tie components were frequently cut to install a door (Fig. 17 ), creating significant alterations to the roof structure's basic structural system.

An example of an attic conversion for residential use
Stairs in residential structures are often positioned in the center of the floor layout, with various residential units on either side. Stairs are not necessarily non-structural features, but in the case of brick structures in Mila, they are not critical for seismic force resistance. Stairs can be monolithic at times, although they are usually fixed to the walls on one side and supported by steel profiles on the other. Many stairs lack a supporting beam and are not monolithic, as seen in Fig. 18 (right). Staircase walls are often thicker and part of a rigid core. The most typical staircase damage is the separation of individual stairs, the falling of plaster (sometimes as thick as 3 cm), and the separation of the stairs from the walls (Fig. 18 ).

Typical stairwell damage
Many staircases were built cantilevered into the wall, resulting in substantial step separations during the earthquake. Finally, in older structures, elevator shafts served as partition walls and were frequently damaged (Fig. 19 ).

Typical elevator shaft damage
Methodology
Assessment procedure.
A quick, preliminary assessment of the continued useability including all structures affected in the earthquake is the first step in a thorough post-seismic building evaluation (Stepinac et al. 2017 ; Uroš et al. 2020 ). The information thus collected may also be used to evaluate old infrastructure and build a modeling and simulation model. The following studies employed similar technologies (Dall’Asta et al. 2019 ; Betti et al. 2021 ). When required, detailed assessment and available Non-Destructive Testing (NDT) assessment methods are preferred (Stepinac et al. 2020 ). With consideration of the safety of civil engineers in the field, a quick initial survey is carried out as quickly and efficiently as possible following the earthquake. In Algeria, this type of evaluation entailed a quick visual inspection of each load-bearing structural component and an assessment of the level of damage, and the assignment of the building into one of six potential categories (Fig. 20 ):
- CU1 Unrestricted use possible (Dark Green label),
- CU2 Useful with suggestions (Green label),
- PCN1 Unusable for the time being, a thorough examination is essential (Dark Yellow label),
- PCN2 temporarily ineffective (Yellow label),
- CN1 Due to extraneous influences, it is no longer usable (Dark Red label), and
- CN2 Due to damage, it is no longer useable (Red label).

Six categories, each with a unique label
Detailed assessment results
On August 8, 2020, within the first 24 h after the earthquake, a rapid evaluation of the case study building was conducted. Following a quick thorough observation of load-bearing structural components, the building was deemed temporally uninhabitable (Dark Yellow label), with a suggestion for a further assessment (PCN1). The preliminary assessment's main findings are as follows:

First floor cracks: wall, lintel ( a ), and ceiling ( b )
- Plaster separation and localized damage;
- Structural components (walls, columns, and arches) have minor damage;
In the eastern part of the structure, diagonal cracks are apparent on load-bearing walls.
The second floor and attic had slight damage, but the eastern (Fig. 22 a, b) and central staircase wings received the most serious damage (Fig. 23 a, b). The structure should be used with caution in areas where there is a risk of plaster collapsing, according to the instructions. Furthermore, while the eastern and western stairs can be used with a limited number of people, the central staircase cannot be used until a comprehensive assessment has been conducted.

Exterior a and internal b cracks on the eastern stairwell

Exterior a and internal b diagonal cracks on the central staircase
The buildings under consideration are in the range of peak ground acceleration of 0.199 g, according to current structural engineering standards shown by RPA ( 2003 ), and governmental codes—which means the expected earthquake intensity is X on the EMS-98 scale for a return period of 475 years. Furthermore, a seismic hazard map for the Mila region was developed according to RPA 2003 criteria based on the latest findings from research conducted by CRAAG in collaboration with the University of Skikda (Hamidatou et al. 2017 ). The soil in the immediate area of the evaluated structure falls into the category of soil type C, according to the specified seismic hazard study (2017–2019).
The damage observations are depicted on the building’s floor plans (Figs. 24 and and25). 25 ). The building was evaluated from the air using an unmanned aerial vehicle, and no damage to the primary load-bearing structure or the roof structure was found. Also examined were decorative crosses, figurines, and reliefs. The 3D model of the building was created using photogrammetric photographs for digital preservation reasons.

Damage pattern and shear strength-testing locations on the building's ground floor

Damage pattern and shear strength-testing locations on the first level of the structure
After a detailed assessment of the structure, the following damages were discovered: cracks in wall hangings, vaults, and ceiling, and separation and local degradation of plaster on the bottom floor. Due to lateral motions during the earthquake, cracks in the barrel vaults are frequently parallel to the supporting joints. The cracks are caused by tensile strains that run perpendicular to the supporting joint. If such fissures develop deeply enough, they can induce hinge formation and, as a result, loss of stability. However, the defects found in the studied structure are superficial and mostly concentrated in the plaster. Minor local damage occurred to the structural elements (walls, columns, and arches). Diagonal fractures on load-bearing walls may be seen in the center core of the structure, where the main stairway is located, and in the eastern part of the building. These fissures can also be noticed on the building's north side. All the floors show visible damage, such as cracks and crumbling plaster on the walls. Minor local damage to the first-floor walls, as well as cracks on the partition walls and ceiling connectors, may be seen.
The east wing's ground and first floors were severely damaged. The cracks that developed spread along the entire wall of the wing's south front. It is unfavorable that the fissures are connected and proceed to the transversely interconnected walls and lintels. The little contribution of a torsional reaction of the structure, where the boundary elements are the most strained and collapse might induce such fractures. In addition, that component of the structure is connected to the adjacent structure. Although this can have a favorable impact in general, in the case of the east wing walls, such a boundary condition might produce extra forces. The walls may fail if they are not well connected to the diaphragms. The wall is not in danger of collapsing because there has been no out-of-plane displacement, but it should be strengthened as soon as possible to prevent further damage. Except for the main staircase, the entire structure is available for use. Depending on the probable future, a static and dynamic analysis of the structure's current condition must be done. Irrespective of how much the entire structure would be repaired, the primary staircase, as well as every other wall with cracks along their length, need to be renovated and repaired. Prior to that, preliminary research on the masonry's characteristics and other essential data for the design and analysis must be performed.
Numerical modeling
The 3Muri software is used to generate a 3D numerical model of the evaluated building. Because of its computational efficiency and excellent accuracy, the macro-element technique is used (Mouyiannou et al. 2014 ).
Its simulation adaptability (including properties of the different elements, realistic floor stiffnesses, strengthening, and many other features) makes it extremely helpful in a location where the great bulk of building stock is brick. This research was based on relevant case analyses in the 3Muri program (Lamego et al. 2017 ; Malcata et al. 2020 ).
The equivalent-frame technique, which employs non-linear beam elements, is used by the macro-element approach. The three categories of macro-elements are piers, spandrels, and stiff nodes (or non-linear beam elements). The piers and spandrels, which are connected by rigid nodes, are the focal points of all deformation. Figure 26 depicts an analogous frame model constructed from these aforementioned macro-elements.

3Muri 3D model and a 3D comparable framework
Non-linear static pushover analysis (Fajfar and Fischinger 1998 ; Cerovecki et al. 2018 ) verifies the limit load ratio employed in numerical solution and provides more comprehensive information on relevant components, failure causes, and the building's overall performance. The pushover study is conducted with constant dynamic loading and monotonically increasing lateral stiffness. Two alternative horizontal load distributions along the building's elevation are investigated in the pushover research. The horizontal load is proportionate to the mass of the building in the first distribution, which also has a high degree of similarity. The horizontal loads are transferred according to the structure's mode pattern in the second distribution first vibration mode form as established by elastic analysis (Figs. 27 and and28). 28 ). Such horizontal forces are imposed in the model at the position of the masses, i.e., at each floor level in the center of the masses. Furthermore, incidental irregularity is carefully considered to account for uncertainty in the determination of the building’s center of mass. For both the x (longitudinal) and y (transversal) directions, 3% of the structure's height perpendicular to the seismic load direction is considered on each side.

Pushover in the y-direction with a mode shape associated with a period of T = 0.26 s

Pushover mode shape in the x-direction, associated with a period T = 0.13 s
In a 3D model, floors are considered as horizontally stiff diaphragms, which is realistic due to the true in-plane rigidity of the horizontal floor elements. In software, rigid diaphragms' in-plane rigidity is limitless, and the mass of the real slab is considered. The roof is removed from the load-bearing structure in the seismic analysis since it has no substantial effect on the structure's reaction and does not contribute to the building's overall resistance. Although it did not meet the criteria, its significance to the model in the form of force was not disregarded.
The numerical model's average values of material parameters (Table (Table4) 4 ) are based on an analysis of relevant literature (Ghiassi et al. 2019 ; EC06 2021 ) as well as on-site testing. Knowledge level 2 (normal knowledge) can be characterized in terms of experimental in situ testing and extensive study of the structure. The confidence factor was set at 1.2 based on the attained knowledge level.
The properties of masonry materials
According to the study in (EC06 2021 ), the building is characterized as normal in height but irregular in floor design, necessitating 3D modeling. The structure is designated as a torsional stiff system. First, static analysis is carried out according to EC06 ( 2021 ) followed by dynamic analysis.
Because seismic resilience is crucial given the consequences of a catastrophe, the pedagogical facility is designated as having relevance class II. As a result, the significance factor is I = 1.2. For two limit states, three PGA values are employed.
The capacity curve represented the ratio of shear force at the structure's base to control node displacement resulting from the seismic analysis. The control node was chosen to be close to the center of mass and is positioned on the building's top story. Figure 29 displays the capacity curves generated from all investigations. Figures 30 and and31 31 show bi-linearized pushover curves in the x and y axes, respectively. The y-axis shows total base shear in kN, while the x-axis shows control node displacement in mm.

Pushover curves for the x (blue) and y (red) axes

The most relevant pushover curve for the x-direction

The most relevant pushover curve for the y-direction
Figures 32 and and33 33 show the damaged condition until the last stage of the pushover curves in the x and y-axis, with yellow indicating shear damage and red indicating bending damage.

Damage at maximum displacement capacity for x-direction pushover. Yellow: shear damage, red: bending damage

Damage at maximum displacement capacity for y-direction pushover. Yellow: shear damage, red: bending damage
The structure's capacity is assessed after the structure's response, and inspections are carried out in line with the fundamental standards pertaining to the status of extensive damage, as defined by limit states. Table Table5 5 shows the parameters for similar SDOF systems from Figs. 32 and and33. 33 . These characteristics are determined through bilinearization by employing the associated energy concept that is used to calculate target movement.
Shows the SDOF parameters for pushover in the x and y directions
The ratio α of the building's limit capacity acceleration to the reference peak ground acceleration on type A ground is also presented in Table Table6. 6 . For all limit states, the parameter is provided. An issue emerges with historic masonry structures, which are frequently unable to be strengthened to the extent required to meet today's seismic-resistant construction requirements.
α values
A study of the walls out-of-plane bending must then be performed. This explains the earthquake activity that occurs perpendicular to the walls. The investigation is carried out for the limited situation that is on the verge of collapsing. Acceleration for a 475-year return period is used. If a wall's MRd/MEd ratio is greater than or equal to 1.0, it is considered to have passed the out-of-plane bending test. In Fig. 34 , the walls that failed the examination are shown in red.

Out-of-plane bending findings. Red: bending damage
In the global study, the 3Muri software also does not take the out-of-plane loss of stability into account. It is believed that appropriate connections are created across walls and diaphragms. Out-of-plane local processes are thus avoided, allowing the global in-plane response of the structure to be studied (Lagomarsino et al. 2013 ). Therefore, the resistance to consolidation is put to the test in a specific program unit; specific elements of a single wall are tested, as well as the interaction of parts of many distinct walls that can generate various local mechanisms when combined. A local mechanism is seen in Fig. 35 , with the mechanical component.

Example of a local mechanism
Local mechanisms are arbitrarily established based on the geometry of the building, common failure mechanisms, and seismic damage. Local mechanisms commonly emerge because of faulty wall and wall-to-floor structural connections. The linear kinematic analysis method is applied. There are three phases to defining a local mechanism. To begin, a kinematic block is a stiff wall element that is sensitive to movement or tilting in relation to another block or the whole of the wall. The initial conditions are then found, and the load is finally calculated. Several of the local progressive collapse of the apparent building, as well as its resistance to the types of failure shown, are listed below (Fig. 36 and Table Table7). 7 ). The ratio of the response spectrum of mechanical activation to the response spectrum of earthquake excitation is represented by the α parameter.

The local mechanisms LM1 (east wing), LM2 (right wing), and LM3 (left wing) are represented in the diagram (central wing)
The findings of the inquiry into local mechanisms
Discussion and conclusions
Based on case studies from recent seismic events in Japan and Italy, (Da Porto et al. 2013 ; Lucibello et al. 2013 ; Formisano 2017 ; Formisano and Marzo 2017 ; Boschi et al. 2018 ; Chieffo and Formisano 2019 ; Malcata et al. 2020 ), the structure under study was assessed to determine its earthquake resilience. The Mila, Algeria’s recent earthquake damaged the structure. The building was not built according to seismic design principles. However, the repair and retrofit improved the structure's condition. Transverse walls are added, and old timber beams are replaced with reinforced concrete flooring. Such stiff diaphragms provide a good connection between all walls and, as a result, improved the seismic behavior of the structure. Therefore, enhancing the rigidity of conventional timber flooring in ancient brick buildings is frequently one of the first seismic retrofitting procedures. On the other hand, a recent study shows that replacing traditional hardwood floors with rigid diaphragms, such as RC flooring, improves energy efficiency. Cracks on the edges of the two materials, or, in the worst-case scenario, disintegration, and collapse of the masonry walls, might be the consequence. This strategy, however, is effective for earthquakes of expected smaller magnitudes, such as those in Mila, and serves to reinforce the existing structure against horizontal actions.
We described the damage to Mila's historic downtown following the earthquake on August 7, 2020. Despite previous calls from the scientific and technical communities, the earthquake demonstrated and verified that awareness, vulnerability mitigation, and readiness are critical to preventing catastrophic seismic consequences and enabling timely action after an earthquake. Although of low magnitude, the Mila earthquake inflicted major damage and economic loss, as well as exposed many weaknesses in the built heritage that people, decision-makers, and the professional and scientific community will have to address for many years to come. The earthquake severely destroyed older masonry structures built before seismic standards were enforced.
The information obtained during the rapid post-earthquake assessment was analyzed and discussed. Damages are graphically shown and illustrated by images. This report examines preliminary data from the database that was enhanced by field engineers. Forms for rapid post-earthquake building evaluation were developed shortly after the 1980 earthquake, and similar forms were used after subsequent earthquakes such as the Tipaza and Zemmouri earthquakes. The findings of the rapid evaluations must be calibrated and reconciled with the extensive reviews provided by the new Act for the rehabilitation of Mila city. In the impacted region, 1044 structures have been identified as severely damaged and will be submitted to further inspections.
3 Muri and an analogous frame approach were used to analyze the existing unconfined masonry structure. Because of its multiple advantages, non-linear static (pushover) seismic analysis is used instead of linear seismic analysis. For the 100, 225, and 475-year return periods, limit state checks were performed. In terms of the structure's existing wall distribution, the results are consistent with the expected behavior. The construction becomes less stiff and has higher displacements in the y direction. Furthermore, the structure's capacity is reduced in the y-direction. There is also some irregularity between the centers of stiffness and mass, which causes torsion to have a minor but unfavorable influence on the overall behavior of the structure. The middle stairwell's walls, as well as the west side of the structure’s cross walls, are crucial elements. Out-of-plane bending failure of walls was also investigated. Using linear kinematics, the causes of failure mode were also examined. The actual damage was compared to the damage calculated using the 3Muri software's non-linear static seismic analysis. From the examination of the structure's behavior during an earthquake, it is evident that strengthening is necessary to improve the structure's seismic performance. An earthquake's damage must be repaired to avoid future damage and threat to the structure's overall strength and stability.
The main results of the investigated area of the city are that the damaged structures are typically older and were built before seismic regulations were implemented. The building materials have degraded over time. Furthermore, the structures are typically under-maintained and, in many cases, designed as seismically deficient. Local (rather than global) in-plane and out-of-plane mechanisms were the most typical failure modes. Significant damage has been found in secondary and/or ornamental building components. Buildings collapsing all at once were extremely infrequent. Aside from concerns with the existing susceptible building stock built prior to seismic regulations, the amount of damage was compounded owing to unlawful conversion of ground levels for commercial activity. There appears to be a widespread lack of understanding and perception of seismic danger in the population, as well as a lack of preventative information dissemination aimed at boosting awareness.
As a result of the several devastating earthquakes that have struck Algeria, the ability to 'build back better’ is much valued. This involves using sustainable resources and producing new ideas (Funari et al. 2020 , 2021 ; Stepinac et al. 2020 ) should be implemented, and energy efficiency should be ensured (Milovanovic and Bagaric 2020 ; Valluzzi et al. 2021 ). Buildings made of masonry can be strengthened using a variety of techniques (Ortega et al. 2017 ; Kouris and Triantafillou 2018 ; Skejic et al. 2020 ). Due to their compatibility and reversibility, materials like FRP (Fiber-Reinforced Polymers) and TRM (Textile-Reinforced Mortars) can be used.
This paper did not receive any funding.
Data availability
Declarations.
The authors declare no conflict of interest. The funders had no role in the design of the study; in the collection, analyses, or interpretation of data; in the writing of the manuscript, or in the decision to publish the results.
Publisher's Note
Springer Nature remains neutral with regard to jurisdictional claims in published maps and institutional affiliations.
- Akkouche K, Hannachi NE, Hamizi M, et al. Knowledge-based system for damage assessment after earthquake: Algerian buildings case. Asian J Civ Eng. 2019; 20 :769–784. doi: 10.1007/s42107-019-00143-z. [ CrossRef ] [ Google Scholar ]
- Allali SA, Abed M, Mebarki A. Post-earthquake assessment of buildings damage using fuzzy logic. Eng Struct. 2018; 166 :117–127. doi: 10.1016/j.engstruct.2018.03.055. [ CrossRef ] [ Google Scholar ]
- Amari K, Abdessemed F, Cheikh Zouaoui M, Uva G. Seismic vulnerability of masonry lighthouses: a study of the bengut lighthouse, Dellys, Boumerdès, Algeria. Buildings. 2020; 10 :247. doi: 10.3390/buildings10120247. [ CrossRef ] [ Google Scholar ]
- ARES (2021) Project first Workshop. Available online: www.grad.hr/ares (Accessed on 15 Feb 2021)
- Atalić J, Šavor Novak M, Uroš M. Seismic risk for Croatia: overview of research activities and present assessments with guidelines for the future. Građevinar. 2019; 71 (10):923–947. [ Google Scholar ]
- Athmania D, Benaissa A, Hammadi A, Bouassida M. Clay and marl formation susceptibility in Mila Province. Algeria Geotech Geol Eng. 2010; 28 (6):805–813. doi: 10.1007/s10706-010-9341-5. [ CrossRef ] [ Google Scholar ]
- Baggio C, Bernardini A, Colozza R, Corazza L, Bella M, Di Pasquale G, Dolce M, Goretti A, Martinelli A, Orsini G et al. (2007) Field manual for post-earthquake damage and safety assessment and short-term countermeasures (AeDES), JRC Sci, Thechnical Reports. 1–100
- Bechtoula H, Ousalem H. The 21 May 2003 Zemmouri (Algeria) earthquake: damages and disaster responses. J Adv Concr Technol. 2005; 3 (1):161–174. doi: 10.3151/jact.3.161. [ CrossRef ] [ Google Scholar ]
- Belazougui M (2008) Boumerdes Algeria earthquake of May 21, 2003: damage analysis and behavior of beam-column reinforced concrete structures. In: proceedings of the 14th world conference on earthquake engineering, Beijing, Paper 14_01–1006
- Benfedda A, Serkhane A, Bouhadad Y, Slimani A, Abbouda M, Bourenane H. The main events of the July-August 2020 Mila (NE Algeria) seismic sequence and the triggered landslides. Arab J Geosci. 2021; 14 :1894. doi: 10.1007/s12517-021-08301-x. [ CrossRef ] [ Google Scholar ]
- Betti M, Bonora V, Galano L, Pellis E, Tucci G, Vignoli A. An integrated geometric and material survey for the conservation of heritage masonry structures. Heritage. 2021; 4 :35. doi: 10.3390/heritage4020035. [ CrossRef ] [ Google Scholar ]
- Bialas J, Oommen T, Rebbapragada U, Levin E. Object-based classification of earthquake damage from high-resolution optical imagery using machine learning. J Appl Remote Sens. 2016; 10 (03):036025. doi: 10.1117/1.JRS.10.036025. [ CrossRef ] [ Google Scholar ]
- Binda L, Saisi A, Tiraboschi C. Investigation procedures for the diagnosis of historic masonries. Constr Build Mater. 2000; 14 (4):199–233. doi: 10.1016/S0950-0618(00)00018-0. [ CrossRef ] [ Google Scholar ]
- Boschi S, Borghini A, Pintucchi B, Bento R, Milani G. Seismic vulnerability of historic masonry buildings: a case study in the center of Lucca. Procedia Struct Integr. 2018; 11 :169–176. doi: 10.1016/j.prostr.2018.11.023. [ CrossRef ] [ Google Scholar ]
- Boukri M, Farsi MN, Mebarki A, Belazougui M. Development of an integrated approach for Algerian building seismic damage assessment. Struct Eng Mech. 2013; 47 (4):471–493. doi: 10.12989/SEM.2013.47.4.471. [ CrossRef ] [ Google Scholar ]
- Boulahia O (2022) Identification, characterization and interaction of seismic sources: Implication on sequences of seismic events of the period 2010–2021 in the North-East of Algeria Doctoral thesis at Sétif University. http://dspace.univ-setif.dz:8888/jspui/handle/123456789/3956
- Bounemeur N, Benzaid R, Kherrouba H, et al. Landslides in Mila town (northeast Algeria): causes and consequences. Arab J Geosci. 2022; 15 :753. doi: 10.1007/s12517-022-09959-7. [ CrossRef ] [ Google Scholar ]
- Bounif MA, Haessler H, Meghraoui M. The Constantine earthquake of October 27, 1985: surface ruptures and aftershock study. Earth Planet Sci Lett. 1987; 85 :451–460. doi: 10.1016/0012-821X(87)90140-3. [ CrossRef ] [ Google Scholar ]
- Casapulla C, Argiento LU, Maione A. Seismic safety assessment of a masonry building according to Italian Guidelines on cultural heritage: simplified mechanical-based approach and pushover analysis. Bull Earthq Eng. 2018; 16 :2809–2837. doi: 10.1007/s10518-017-0281-9. [ CrossRef ] [ Google Scholar ]
- Cerovecki A, Kraus I, Moric D. N2 building design method. Gradjevinar. 2018; 70 :509–518. [ Google Scholar ]
- Chieffo N, Formisano A. Comparative seismic assessment methods for masonry building aggregates: a case study. Front Built Environ. 2019; 5 :123. doi: 10.3389/fbuil.2019.00123. [ CrossRef ] [ Google Scholar ]
- Chimouni R, Harbi A, Boughacha MS, Hamidatou M, Kherchouche R, Sebaï A. The 1790 Oran earthquake, a seismic event in times of conflict along the Algerian coast: a critical review from western and local source materials. Seismol Res Lett. 2018; 89 (6):2392–2403. doi: 10.1785/0220180175. [ CrossRef ] [ Google Scholar ]
- Coiffait PE (1992) A post-nappe basin in its structural framework: The example of the Constantine basin (North-East Algeria). Dessertation, University of Nancy
- Da Porto F, Munari M, Prota A, Modena C. Analysis and repair of clustered buildings: case study of a block in the historic city center of L’Aquila (Central Italy) Constr Build Mater. 2013; 38 :1221–1237. doi: 10.1016/j.conbuildmat.2012.09.108. [ CrossRef ] [ Google Scholar ]
- Dall’Asta A, Leoni G, Meschini A, Petrucci E, Zona A. Integrated approach for seismic vulnerability analysis of historic massive defensive structures. J Cult Herit. 2019; 35 :86–98. doi: 10.1016/j.culher.2018.07.004. [ CrossRef ] [ Google Scholar ]
- De Luca FGED, Woods C, Galasso D, D’Ayala RC. In filled building performance against the evidence of the 2016 EEFIT Central Italy post-earthquake reconnaissance mission: empirical fragilities and comparison with the FAST method. Bull Earthq Eng. 2018; 16 (7):2943–2969. doi: 10.1007/s10518-017-0289-1. [ CrossRef ] [ Google Scholar ]
- Didier M, Baumberger S, Tobler R, Esposito S, Ghosh S, Stojadinovic B. Improving post-earthquake building safety evaluation using the 2015 Gorkha, Nepal, Earthquake rapid visual damage assessment data. Earthq Spectra. 2017; 33 :415–438. doi: 10.1193/112916eqs210m. [ CrossRef ] [ Google Scholar ]
- Durand DM. Focus on the structure of the Northeast of the BERBERIE. Bull Serv Carte Geol Agerie NS. 1969; 39 :89–131. [ Google Scholar ]
- EMS (1998) Comision Sismologica Europea, Escala Macro Sísmica Europea EMS 98(15)
- Endo Y, Pelà L, Roca P. Review of different pushover analysis methods applied to masonry buildings and comparison with nonlinear dynamic analysis. J Earthq Eng. 2017; 21 :1234–1255. doi: 10.1080/13632469.2016.1210055. [ CrossRef ] [ Google Scholar ]
- Eurocode 6 (2021) Design of masonry structures—Part 1–1: general rules for reinforced and unreinforced masonry structures. Available online: https://www.phd.eng.br/wp-content/uploads/2015/02/en.1996.1.1.2005.pdf
- Eurocode 8 (2004a): Design of structures for earthquake resistance—Part 1: general rules, seismic actions and rules for buildings. EN 1998–1: European Committee for Standardization, Brussels. https://www.phd.eng.br/wp-content/uploads/2015/02/en.1998.1.2004a.pdf
- Eurocode 8 (2004b) Design of structures for earthquake resistance—Part 3: assessment and retrofitting of buildings. Available online: https://www.phd.eng.br/wp-content/uploads/2014/07/en.1998.3.2005.pdf
- Fajfar P, Fischinger M (1998) N2—a method for non-linear seismic analysis of regular buildings. In: proceedings of the 9th world conference in earthquake engineering, Tokyo-Kyoto, Japan, 2–9 August 1998, pp 111–116
- Farsi MN, Belazougui M (1992) The Mont Chenoua (Algeria) earthquake of October 29th 1989; damage assessement and distribution", Proceedings of the 10th world conference on earthquake engineering, Madrid, Spain
- Formisano A. Theoretical and numerical seismic analysis of masonry building aggregates: case studies in San Pio Delle Camere (L’Aquila, Italy) J Earthq Eng. 2017; 21 :227–245. doi: 10.1080/13632469.2016.1172376. [ CrossRef ] [ Google Scholar ]
- Formisano A, Marzo A. Simplified and refined methods for seismic vulnerability assessment and retrofitting of an Italian cultural heritage masonry building. Comput Struct. 2017; 180 :13–26. doi: 10.1016/j.compstruc.2016.07.005. [ CrossRef ] [ Google Scholar ]
- Funari MF, Mehrotra A, Lourenço PB. A tool for the rapid seismic assessment of historic masonry structures based on limit analysis optimisation and rocking dynamics. Appl Sci. 2021; 11 :942. doi: 10.3390/app11030942. [ CrossRef ] [ Google Scholar ]
- Funari MF, Spadea S, Lonetti P, Fabbrocino F, Luciano R. Visual programming for structural assessment of out-of-plane mechanisms in historic masonry structures. J Build Eng. 2020; 31 :101425. doi: 10.1016/j.jobe.2020.101425. [ CrossRef ] [ Google Scholar ]
- Ghiassi B, Vermelfoort AT, Lourenço PB (2019) Masonry Mechanical Properties. In: Numerical modeling of masonry and historical structures. Woodhead Publishing, pp 239–261
- Grillanda N, Valente M, Milani G, Chiozzi A, Tralli A. Advanced numerical strategies for seismic assessment of historical masonry aggregates. Eng Struct. 2020; 212 :110441. doi: 10.1016/j.engstruct.2020.110441. [ CrossRef ] [ Google Scholar ]
- Guemache MA, Machane D, Beldjoudi H, Gharbi S, Djadia L, Benahmed S, Ymmel H. On a damaging earthquake-induced landslide in the Algerian Alps: the March 20, 2006 Laâlam landslide (Babors chain, northeast Algeria), triggered by the Kherrata earthquake (Mw = 5 3) Nat Hazards. 2010; 54 (2):273–288. doi: 10.1007/s11069-009-9467-z. [ CrossRef ] [ Google Scholar ]
- Halla N, Hamidatou M, Hamai L, Atmane L, Yelles chaouche A (2022) Earthquake induced landslide in Milla region: the August 07, 2020 (El-Kherba, Grarem-Gouga and Azzeba) landslides Northeast Algeria, triggered by the Mila earthquake (Mw = 5). In process for publication, Naturals hazards journal
- Hamidatou M, Mohammedi Y, Hallal N, Yelles-Chaouche A, Lebdioui S, Thallak I, Stromeyer D, Khemici O. Reply to the comment on the paper “seismic hazard analysis of surface level, using topographic condition in Northeast of Algeria” by Mohamed Hamdache and José A. Pelàez. Pure Appl Geophys. 2021; 178 :305–312. doi: 10.1007/s00024-020-02644-4. [ CrossRef ] [ Google Scholar ]
- Hamidatou M, Mohammedi Y, Yelles-Chaouche A, Thallak I, Stromeyer D, Lebdioui S, Cotton F, Hallal N, Khemici O. Seismic hazard analysis of surface level, using topographic condition in the Northeast of Algeria. Pure Appl Geophys. 2019; 178 (3):823–846. doi: 10.1007/s00024-019-023104. [ CrossRef ] [ Google Scholar ]
- Hamidatou M, Sbartai B. Deterministic assessment of seismic risk in Constantine city, Northeast Algeria. Nat Hazards. 2016; 86 (2):441–464. doi: 10.1007/s11069-016-2693-2. [ CrossRef ] [ Google Scholar ]
- Hamidatou M, Sbartai B. Probabilistic seismic hazard assessment in the Constantine region, Northeast of Algeria. Arab J Geosci. 2017; 10 (6):1–20. doi: 10.1007/s12517017-2876-5. [ CrossRef ] [ Google Scholar ]
- Harbi A, Maouche S, Ousadou F, Rouchiche Y, Yelles-Chaouche A, Merahi M, Heddar A, Nouar O, Kherroubi A, Beldjoudi H, Ayadi A, Benouar D. Macroseismic study of the Zemmouri earthquake of 21 May 2003 (Mw 6.8, Algeria) Earthq Spectra. 2007; 23 (2):315–332. doi: 10.1193/1.2720363. [ CrossRef ] [ Google Scholar ]
- Harbi A, Maouche S, Vaccari F, Aoudia A, Oussadou F, Panza GF, Benouar D. Seismicity, seismic input and site effects in the Sahel-Algiers Region (North Algeria) Soil Dyn Earth Eng. 2007; 27 (5):427–447. doi: 10.1016/j.soildyn.2006.10.002. [ CrossRef ] [ Google Scholar ]
- Harbi A, Meghraoui M, Maouche S. The Djidjelli (Algeria) earthquakes of 21 and 22 August 1856 (I0 VIII, IX) and related tsunami effects Revisited. J Seismol. 2011; 15 :105–129. doi: 10.1007/s10950-010-9212-9. [ CrossRef ] [ Google Scholar ]
- Hellel M, Chatelain JL, Guillier B, Machane D, Ben Salem R, Oubaiche E, Haddoum H. Heavier damages without site effects and site effects with lighter damages: Boumerdes City (Algeria) after the May 2003 earthquake. Seismol Res Lett. 2010; 81 (1):37–43. doi: 10.1785/gssrl.81.1.37. [ CrossRef ] [ Google Scholar ]
- Hichem N, Ahmed M, Mohamed A. Post-quake structural damage evaluation by neural networks: theory and calibration. Eur J Environ Civ Eng. 2019; 23 :710–727. doi: 10.1080/19648189.2017.1304277. [ CrossRef ] [ Google Scholar ]
- Indirli MS, Kouris LA, Formisano L, Borg RP, Mazzolani FM. Seismic damage assessment of unreinforced masonry structures after the abruzzo 2009 earthquake: the case study of the historical centers of L’Aquila and castelvecchio subequo. Int J Architect Herit. 2013; 7 (5):536–578. doi: 10.1080/15583058.2011.654050. [ CrossRef ] [ Google Scholar ]
- Karimzadeh S, Askan A, Erberik MA, Yakut A. Seismic damage assessment based on regional synthetic ground motion dataset: a case study for Erzincan, Turkey. Nat Hazards. 2018; 92 (3):1371–1397. doi: 10.1007/s11069-018-3255-6. [ CrossRef ] [ Google Scholar ]
- Karimzadeh S, Kadas K, Askan A, Erberik MA, Yakut A. Derivation of analytical fragility curves using SDOF models of masonry structures in Erzincan (Turkey) Earthq Struct. 2020; 18 (2):249–261. [ Google Scholar ]
- Kim T, Song J, Kwon OS. Pre- and post-earthquake regional loss assessment using deep learning. Earthq Eng Struct Dyn. 2020; 49 :657–678. doi: 10.1002/eqe.3258. [ CrossRef ] [ Google Scholar ]
- Kouris LAS, Triantafillou TC. State-of-the-art on strengthening of masonry structures with textile reinforced mortar (TRM) Constr Build Mater. 2018; 188 :1221–1233. doi: 10.1016/j.conbuildmat.2018.08.039. [ CrossRef ] [ Google Scholar ]
- Lagomarsino S, Penna A, Galasco A, Cattari S. TREMURI program: an equivalent frame model for the nonlinear seismic analysis of masonry buildings. Eng Struct. 2013; 56 :1787–1799. doi: 10.1016/j.engstruct.2013.08.002. [ CrossRef ] [ Google Scholar ]
- Lamego P, Lourenço PB, Sousa ML, Marques R. Seismic vulnerability and risk analysis of the old building stock at urban scale: application to a neighbourhood in Lisbon. Bull Earthq Eng. 2017; 15 :2901–2937. doi: 10.1007/s10518-016-0072-8. [ CrossRef ] [ Google Scholar ]
- Laouami N, Slimani A, Larbes S. Ground motion prediction equations for Algeria and surrounding region using site classification based H/V spectral ratio. Bull Earthq Eng. 2018; 16 (7):2653–2684. doi: 10.1007/s10518-018-0310-3. [ CrossRef ] [ Google Scholar ]
- Laouami N, Slimani N, Bouhadad Y, Chatelain JL, Nour A. Evidence for fault-related directionality and localized site effects from strong motion recordings of the 2003 Boumerdes (Algeria) earthquake: consequences on damage distribution and the Algerian Seismic Code. Soil Dyn Earth Eng. 2006; 26 :991–1003. doi: 10.1016/j.soildyn.2006.03.006. [ CrossRef ] [ Google Scholar ]
- Lourenco P, Karanikoloudis G. Seismic behavior and assessment of masonry heritage structures. Needs in engineering judgement and education. RILEM Tech Lett. 2019; 3 :114–120. doi: 10.21809/rilemtechlett.2018.76. [ CrossRef ] [ Google Scholar ]
- Lucibello G, Brandonisio G, Mele E, De Luca A. Seismic damage and performance of Palazzo Centi after L’Aquila earthquake: a paradigmatic case study of effectiveness of mechanical steel ties. Eng Fail Anal. 2013; 34 :407–430. doi: 10.1016/j.engfailanal.2013.09.011. [ CrossRef ] [ Google Scholar ]
- Malcata M, Ponte M, Tiberti S, Bento R, Milani G. Failure analysis of a Portuguese cultural heritage masterpiece: Bonet building in Sintra. Eng Fail Anal. 2020; 115 :104636. doi: 10.1016/j.engfailanal.2020.104636. [ CrossRef ] [ Google Scholar ]
- Marmi R, Kacimi M, Boularak M. Landslides in the Mila region (North-Eastern Algeria): impact on infrastructure. Revista De Geomorphologie. 2008; 10 :51–56. [ Google Scholar ]
- Marshall JD, Jaiswal K, Gould N, Turner F, Lizundia B, Barnes JC. Post-earthquake building safety inspection: lessons from the canterbury, New Zealand, earthquakes. Earthq Spectra. 2013; 29 :1091–1107. doi: 10.1193/1.4000151. [ CrossRef ] [ Google Scholar ]
- Medhat NI, Yamamoto M-Y, Tolomei C, Harbi A, Maouche S (2022) Multi-temporal InSAR analysis to monitor landslides using the small baseline subset (SBAS) approach in the Mila Basin, Algeria, Terra Nova published by John Wiley & Sons Ltd. 10.1111/ter.12591
- Mehani Y, Bechtoula H, Kibboua A, Naili M. Assessment of seismic fragility curves for existing RC buildings in Algiers after the 2003 Boumerdes earthquake. Struct Eng Mech. 2013; 46 (6):791–808. doi: 10.12989/SEM.2013.46.6.791. [ CrossRef ] [ Google Scholar ]
- Merghadi A, Abderrahmane B, Tien Bui D. Landslide susceptibility assessment at Mila Basin (Algeria): a comparative assessment of prediction capability of advanced machine learning methods. ISPRS Int J Geo Inf. 2018; 7 (7):268. doi: 10.3390/ijgi7070268. [ CrossRef ] [ Google Scholar ]
- Meslem A, Yamazaki F, Maruyama Y, Benouar D, Kibboua A, Mehani Y. The effects of building characteristics and site conditions on the damage distribution in Boumerdès after the 2003 Algeria earthquake. Earthq Spectra. 2012; 28 (1):185–216. doi: 10.1193/1.3675581. [ CrossRef ] [ Google Scholar ]
- Milovanovic B, Bagaric M. How to achieve nearly zero-energy buildings standard. Gradjevinar. 2020; 72 :703–720. [ Google Scholar ]
- Mouyiannou A, Rota M, Penna A, Magenes M. Identification of suitable limit states from nonlinear dynamic analyses of masonry structures. J Earthq Eng. 2014; 18 :231–263. doi: 10.1080/13632469.2013.842190. [ CrossRef ] [ Google Scholar ]
- Naito S, Tomozawa H, Mori Y, Nagata T, Monma N, Nakamura H, Fujiwara H, Shoji G. Building-damage detection method based on machine learning utilizing aerial photographs of the Kumamoto earthquake. Earthq Spectra. 2020; 36 :1166–1187. doi: 10.1177/8755293019901309. [ CrossRef ] [ Google Scholar ]
- Ortega J, Vasconcelos G, Rodrigues H, Correia M. Assessment of the influence of horizontal diaphragms on the seismic performance of vernacular buildings. Bull Earthq Eng. 2018; 16 :3871–3904. doi: 10.1007/s10518-018-0318-8. [ CrossRef ] [ Google Scholar ]
- Ortega J, Vasconcelos G, Rodrigues H, Correia M, Ferreira TM, Vicente R. Use of post-earthquake damage data to calibrate, validate and compare two seismic vulnerability assessment methods for vernacular architecture. Int J Disaster Risk Reduct. 2019; 39 :101242. doi: 10.1016/j.ijdrr.2019.101242. [ CrossRef ] [ Google Scholar ]
- Ortega J, Vasconcelos G, Rodrigues H, Correia M, Lourenço PB. Traditional earthquake resistant techniques for vernacular architecture and local seismic cultures: a literature review. J Cult Herit. 2017; 27 :181–196. doi: 10.1016/j.culher.02.015. [ CrossRef ] [ Google Scholar ]
- Ousadou F, Dorbath L, Dorbath C, Bounif MA, Benhallou H. The Constantine (Algeria) seismic sequence of 27 October 1985: a new rupture model from aftershock relocation, focal mechanisms, and stress tensors. J Seismol. 2012; 17 (2):207–222. doi: 10.1007/s10950-012-9320-9. [ CrossRef ] [ Google Scholar ]
- Palazzi NC, Barrientos M, Sandoval C de la Llera JC (2022) Seismic Vulnerability Assessment of the Yungay's Historic Urban Center in Santiago, Chile. J Earthq Eng 1–28
- Penna AP, Morandi M, Rota CF, Manzini F, Da Porto G. Magenes, Performance of masonry buildings during the Emilia 2012 earthquake. Bull Earthq Eng. 2014; 12 (5):2255–2273. doi: 10.1007/s10518-013-9496-6. [ CrossRef ] [ Google Scholar ]
- Raoult JF (1974) Geology of the Center of the numidic chain (North Constantinois, Algeria). Thesis Paris Mem Soc Geol 121: 63
- Remki M, Benouar D. Damage potential and vulnerability functions of strategic buildings in the city of Algiers. KSCE J Civ Eng. 2014; 18 :1726–1734. doi: 10.1007/s12205-014-0184-0. [ CrossRef ] [ Google Scholar ]
- Rodríguez AS, Rodríguez BR, Rodríguez MS, Sánchez PA (2019) 9-Laser scanning and its applications to damage detection and monitoring in masonry structures. In: Ghiassi B, Lourenço PB (eds) Woodhead Publishing Series in Civil and Structural Engineering, Long-term Performance and Durability of Masonry Structures, Woodhead Publishing, pp 265–285
- RPA (2003) Algerian Earthquake Rules 1999. Version 2003. National Center of Applied Research in Earthquake Engineering (CGS)
- Semmane F, Abacha I, Yelles-Chaouche AK, et al. The earthquake swarm of December 2007 in the Mila region of northeastern Algeria. Nat Hazards. 2012; 64 :1855–1871. doi: 10.1007/s11069-012-0338-7. [ CrossRef ] [ Google Scholar ]
- Skejic D, Lukacevic I, Curkovic I, Cudina I. Application of steel in refurbishment of earthquake-prone buildings. Gradjevinar. 2020; 72 :955–966. [ Google Scholar ]
- Smail T, Abed M, Mebarki A, Lazecky M. Earthquake-induced landslide monitoring and survey by means of InSAR. Nat Hazards Earth Syst Sci. 2022; 22 :1609–1625. doi: 10.5194/nhess-22-1609-2022. [ CrossRef ] [ Google Scholar ]
- Stepinac M, Gašparovic M. A review of emerging technologies for an assessment of safety and seismic vulnerability and damage detection of existing masonry structures. Appl Sci. 2020; 10 :5060. doi: 10.3390/app10155060. [ CrossRef ] [ Google Scholar ]
- Stepinac M, Kisicek T, Renic T, Hafner I, Bedon C. Methods for the assessment of critical properties in existing masonry structures under seismic loads-The ARES project. Appl Sci. 2020; 10 (5):1576. doi: 10.3390/app10051576. [ CrossRef ] [ Google Scholar ]
- Stepinac M, Rajcic V, Barbalic J. Inspection and condition assessment of existing timber structures. Gradjevinar. 2017; 69 :861–873. [ Google Scholar ]
- Tebbouche MY, Ait Benamar D, Hassan HM, et al. Characterization of El Kherba landslide triggered by the August 07, 2020, Mw = 4.9 Mila earthquake (Algeria) ambient noise analysis. Environ Earth Sci. 2022; 81 :46. doi: 10.1007/s12665-022-10172-8. [ CrossRef ] [ Google Scholar ]
- Uroš M, Šavor Novak M, Atalc J, Sigmund Z, Banicek M, Demšic M, Hak S. Post-earthquake damage assessment of buildings–procedure for conducting building inspections. Gradjevinar. 2020; 72 :1089–1115. [ Google Scholar ]
- Valente M, Milani G. Damage assessment and collapse investigation of three historical masonry palaces under seismic actions. Eng Fail Anal. 2019; 98 :10–37. doi: 10.1016/j.engfailanal.2019.01.066. [ CrossRef ] [ Google Scholar ]
- Valluzzi MR, Salvalaggio M, Croatto G, Dorigatti G, Turrini U. Nested buildings: an innovative strategy for the integrated seismic and energy retrofit of existing masonry buildings with CLT panels. Sustainability. 2021; 13 :1188. doi: 10.3390/su13031188. [ CrossRef ] [ Google Scholar ]
- Vila JM (1980) Geological map at 1/50.000. Dissertation, University of Paris VI
- Vlachakis G, Vlachaki E, Lourenço PB. Learning from failure: damage and failure of masonry structures, after the 2017 lesvos earthquake (Greece) Eng Fail Anal. 2020; 117 :104803. doi: 10.1016/j.engfailanal. [ CrossRef ] [ Google Scholar ]
- Yavari S, Chang SE, Elwood KJ. Modeling post-earthquake functionality of regional health care facilities. Earthq Spectra. 2010; 26 :869–892. doi: 10.1193/1.3460359. [ CrossRef ] [ Google Scholar ]
- Zhang Y, Burton HV, Sun H, Shokrabadi M. A machine-learning framework for assessing post-earthquake structural safety. Struct Saf. 2018; 72 :1–16. doi: 10.1016/j.strusafe.2017.12.001. [ CrossRef ] [ Google Scholar ]
Thank you for visiting nature.com. You are using a browser version with limited support for CSS. To obtain the best experience, we recommend you use a more up to date browser (or turn off compatibility mode in Internet Explorer). In the meantime, to ensure continued support, we are displaying the site without styles and JavaScript.
- View all journals
- My Account Login
- Explore content
- About the journal
- Publish with us
- Sign up for alerts
- Open access
- Published: 17 October 2023
The overall-subshear and multi-segment rupture of the 2023 Mw7.8 Kahramanmaraş, Turkey earthquake in millennia supercycle
- Liuwei Xu ORCID: orcid.org/0000-0001-8881-5834 1 ,
- Saeed Mohanna ORCID: orcid.org/0000-0003-2101-5124 1 ,
- Lingsen Meng ORCID: orcid.org/0000-0003-2428-0548 1 ,
- Chen Ji ORCID: orcid.org/0000-0002-0350-5704 2 ,
- Jean-Paul Ampuero ORCID: orcid.org/0000-0002-4827-7987 3 ,
- Zhang Yunjun ORCID: orcid.org/0000-0001-9441-7082 4 ,
- Masooma Hasnain ORCID: orcid.org/0009-0004-6702-6181 1 ,
- Risheng Chu ORCID: orcid.org/0000-0002-1062-5982 5 &
- Cunren Liang 6
Communications Earth & Environment volume 4 , Article number: 379 ( 2023 ) Cite this article
3176 Accesses
4 Citations
27 Altmetric
Metrics details
- Natural hazards
On February 6, 2023, an Mw7.8 earthquake hit the East Anatolian Fault (EAF) and Narlı Fault (NF), followed by an Mw7.5 event on the Sürgü Fault. We combine multiple seismic datasets, global navigation satellite system recordings, and radar satellite images with finite fault inversion and slowness enhanced back-projection to study the rupture kinematics. Our analysis reveals that the rupture originated on the NF, propagating 120 km northeast at 3.05 km/s and 200 km southwest at 3.11 km/s after reaching the EAF junction, exhibiting overall subshear speeds. Further Mach wave analysis confirms the subshear rupture, matching the prediction using close-Rayleigh speeds. The unexpectedly-large slip on some EAF segments suggests a supercycle lasting ≥900 years. The EAF geometry is similar to the San Andreas-San Jacinto Fault system, while the latter has higher slip rates but without large earthquakes on its southern segments since 1857, carrying the potential of an M8 earthquake.
Similar content being viewed by others
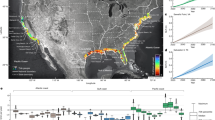
Disappearing cities on US coasts
Leonard O. Ohenhen, Manoochehr Shirzaei, … Robert J. Nicholls
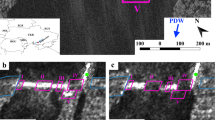
Pre-collapse spaceborne deformation monitoring of the Kakhovka dam, Ukraine, from 2017 to 2023
Amin Tavakkoliestahbanati, Pietro Milillo, … Giorgia Giardina
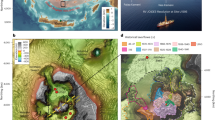
Hazardous explosive eruptions of a recharging multi-cyclic island arc caldera
Jonas Preine, Jens Karstens, … Dimitrios Papanikolaou
Introduction
On February 6, 2023, two powerful earthquakes of moment magnitude (Mw) 7.8 and 7.5 hit south-central Turkey and northern Syria (Fig. 1 ). The earthquakes, which occurred only 9 h apart, shook the regions around Gaziantep, Kahramanmaraş, Malatya, and Hatay, severely affecting the local population. These earthquakes produced ruptures that are among the longest ever recorded in continental strike-slip earthquakes, with fault lengths of ~368 km and ~133 km for the Mw 7.8 and Mw 7.5 ruptures, respectively 1 . The earthquakes triggered a basin-wide tsunami alert, and a small tsunami was generated in the Eastern Mediterranean Sea. The two earthquakes resulted in severe damage to buildings and infrastructure in Turkey and Syria, with a reported loss of at least 50,000 lives and many more injured 2 .
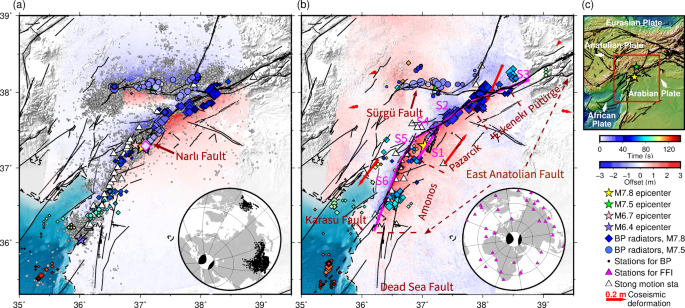
Diamonds denote the High-Freq radiators for the Mw 7.8 earthquake, color-coded by rupture time relative to the origin time of the event and with size proportional to the normalized BP power. Radiators imaged by the China array are shown in a and by the Alaska array are shown in b . All China and Alaska stations are shown in the lower inset of a . Color circles denote the same as diamonds but for the Mw 7.5 earthquake. Blue to red background shows the ground displacement in east-west direction ( a ) and in north-south direction ( b ) from radar satellites (Methods). a The gray dots denote the seismicities occurring from Jan 1st, 2023 to Mar 14th, 2023, from the AFAD catalog. The white triangles denote the strong motion stations used in SEBP validation (Methods). The diamonds with magenta edges correspond to the 5 s radiator (see Results section). b S1–S6 denote the vertical fault planes adopted for FFI. The red arrows indicate the coseismic deformation measured by GNSS stations. The white and magenta triangles denote the strong motion stations and teleseismic stations used by joint FFI, respectively. The segments of the EAF by Duman and Emre 32 are denoted by the brown dash lines and labeled names. c The tectonic setting of the 2023 Mw 7.8 Kahramanmaraş earthquake. The red rectangle indicates the study region in a and b . The fault trace data are from Emre et al. 14 , Mahmoud et al. 95 , and Styron et al. 96 .
The earthquake doublet occurred in the East Anatolian Fault Zone (EAFZ), and the rupture reached the northern end of the Dead Sea Fault Zone (DSFZ). The DSFZ is a major tectonic boundary between the African and Arabian plates in the Middle East. It has a complex geometry and has caused devastating earthquakes in the past, including the 1138 M7.1 Aleppo, 1837 M7.1 Galilee, and 1927 M6.3 Jericho earthquakes. The EAFZ is a major left-lateral strike-slip fault that runs for approximately 700 km (ref. 3 ) along the eastern edge of the Anatolian Plateau. It has been responsible for numerous large earthquakes in the past, including the 995 (M7.4), 1114 (M ≥ 7.8), 1789 (M7.4), 1893 (M7.1), 1905 (M6.8) events 4 , 5 , 6 . More recently, there has been a series of damaging earthquakes across southeastern Turkey including the 1998 M6.3 Adana-Ceyhan, 2003 M6.4 Bingol, 2011 M7.1 Van, 2010 M6.1, and 2020 M6.7 Elazig earthquakes. Fault mapping of the EAFZ shows several fault segments and splay faults that have accommodated varying degrees of seismicity throughout their histories 3 , 7 . Prior to the 2023 earthquakes, the 2020 Elazig earthquake was the most recent M > 6.5 event to rupture the EAF. It occurred further east, on the Pütürge segment of the EAF, and it was not estimated to increase the Coulomb stress on the Pazarcık segment 8 , 9 . The 2023 Mw 7.8 earthquake started close to the junction of the Pazarcık and Amonos segments of the EAF. The Disaster and Emergency Management Authority of Turkey (AFAD) placed its epicenter close to a splay fault referred to as the Narlı Fault (NF). The Mw 7.5 aftershock occurred about 90 km north, on the Cardak fault segment of the Sürgü Fault.
To analyze the source processes associated with the Kahramanmaraş earthquakes in Turkey, we utilize two methodologies: seismic array Slowness Enhanced Back-Projection (SEBP) 10 and joint Finite Fault Inversion (FFI) 11 , 12 . SEBP is applied to both the Mw 7.8 mainshock and the Mw 7.5 aftershock, while FFI is specifically applied to the Mw 7.8 mainshock and resolves the slip distribution on the NF and EAF. SEBP and joint FFI indicate that during the Mw 7.8 mainshock, the rupture first initiates and propagates on the NF. After reaching the junction with the EAF, it propagates bilaterally at overall subshear speeds, extending 120 km to the northeast at 3.05 km/s and 200 km to the southwest at 3.11 km/s. We further validate subshear speeds by local seismic recordings and far-field Rayleigh waves analysis. Our investigations reveal the presence of an earthquake supercycle on the EAF whose complete period is ≥900 years. We discover geometric similarities between the EAF system and the San Andreas Fault system. These findings hold crucial implications for seismic hazard evaluations in California and other global strike-slip fault systems.
Results and discussion
Rupture kinematics of the mw 7.8 and 7.5 events.
We apply the SEBP and joint FFI to analyze the source processes associated with the 2023 Mw 7.8 Kahramanmaraş, Turkey earthquake and apply SEBP to the Mw 7.5 event. The slip planes of the Mw 7.8 Kahramanmaraş earthquake are constructed on six vertical fault segments (segments S1–S6, Fig. 1b ) extracted from the SAR image-based surface traces (Fig. 1 a, b) and aftershock distributions. During the joint FFI study, we divide these fault segments into subfaults and invert for the rupture histories of individual subfaults simultaneously 11 , 12 using seismic waveforms observed in both close-fault (<50 km) strong motion and teleseismic (3000–10,000 km) stations as well as the ground deformation captured by high-rate Global Navigation Satellite System (GNSS), and Synthetic Aperture Radar (SAR) speckle tracking from Sentinel-1 satellite, InSAR and multiple aperture interferometry (MAI) from ALOS-2 and LuTan-1 satellites (Supplementary Figs. 1 – 8 ). The SEBP analysis is based on the technique developed by Meng et al. 10 (see Methods for more details). We collect the broadband seismograms from two large-aperture arrays at teleseismic distance, located in China (CH) and Alaska (AK; the lower inset in Fig. 1a ). Slowness correction terms for the mainshock are derived using nearby aftershocks (Supplementary Table 1 and Supplementary Figs. 9 and 10 ) to further mitigate the spatial bias caused by path effects (Methods).
To account for the influence of random seed selection in simulated annealing inversions, we conduct 10 FFIs for the Mw 7.8 event using different random seeds. This approach helps us explore the uncertainty arising from multiple optimal solutions within the model space. All 10 inversions result in similar solutions as expected, with negligible standard deviation on the objective function values (~0.6% of the average), coseismic slip distributions and moment rate functions (Supplementary Fig. 11 ). Considering that all 10 models are plausible solutions, we present the model with the smallest objective function value. Its coseismic slip distribution is shown in Fig. 2 and Supplementary Figs. 12 and 13 , the rupture evolution on fault planes is presented in Supplementary Movie 1 , and the coseismic slip rate is shown in Supplementary Fig. 13b . The final coseismic moment is M 0 = 7.67 × 10 20 Nm (Mw 7.85), with an average slip of 3.1 m. The preferred slip model features three large slip asperities north and south of the hypocenter, one located on the EAF near the junction with the splay fault, another at 70 km northeast of the junction, and one at the south Pazarcik and north Amanos segments. The peak slip is 9.2 m and occurs on the Pazarcık segment. The south rupture on the Amanos segment of the EAF has relatively uniform and shallow slip, with an average value of ~3.1 m. The along-fault averaged slip as a function of depth is shown in Supplementary Fig. 14 . The largest average slip occurs at ~4 km depth, with a value of 4.4 m. According to the FFI, the rupture duration is ~100 s, with the southwest rupture tip reaching the southern Amanos fault, close to the Karasu Fault (Fig. 1 ). The total rupture length is estimated to be ~370 km, based on our SEBP results. This value is very close to that estimated by field and satellite data (~368 km, ref. 1 ).
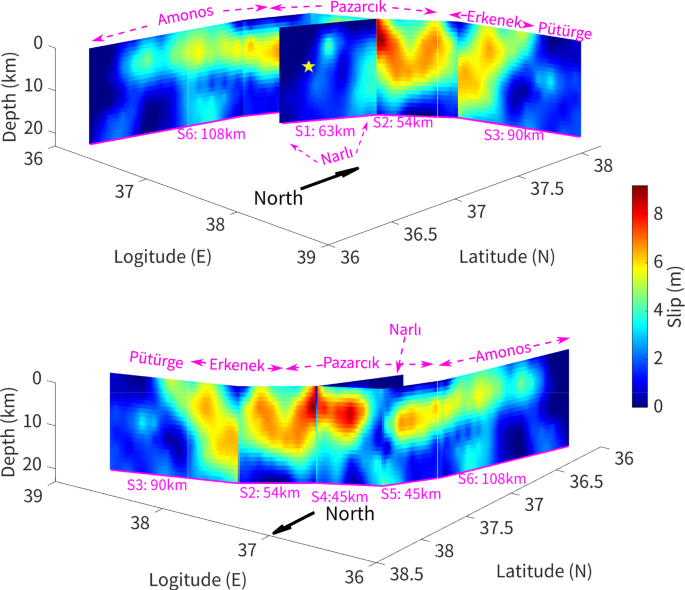
Segment indices and lengths are labeled at bottom. Fault names are annotated on the top. The yellow star denotes the hypocenter.
Our FFI analysis reveals that the 2023 Mw 7.8 Kahramanmaraş mainshock has a heterogeneous rupture process. As shown in Supplementary Fig. 13a and Movie 1 , the rupture starts on the splay NF, but 92% of the seismic moment is released on the Erkenek, Pazarcik, and Amanos segments of the EAF as three high slip patches (Fig. 2 and Supplementary Fig. 15 ). The rupture first propagates on the ~N33.5°E oriented NF for ~50 km and reaches the NF’s northern end at ~20 s, consistent with the SEBP results of the AK and CH arrays (Fig. 3b ). At the junction of the NF and the EAF, our slip model shows spatially and temporally complex rupture fronts. The earliest slip on the EAF occurs at ~15 s, then propagates bilaterally towards the northeast and southwest. The northeast rupture lasts ~40 s and extends to the Pütürge segment. The southwest rupture continues on the Pazarcık and Amanos segments for ~80 s after the junction. To better compare the rupture evolution imaged by the SEBP and FFI, we project the high-frequency (High-Freq) radiators imaged by SEBP onto the FFI fault planes (Fig. 3b ). The subfaults’ slip rates, defined as the slip amplitude divided by the rise time, are averaged across the depth and plotted as a function of their curvilinear distance along the FFI faults. The High-Freq radiators imaged by SEBP show an overall subshear northeast rupture process consistent with the FFI slip model, with an average propagation speed of ~3.05 km/s. The first 80 km of the southwest rupture is only partially imaged by SEBP and is obscured by the strong northeast front; this interference is expected when the separation distance between radiators of a bilateral rupture is shorter than the BP resolution 13 . The interference of the bilateral fronts also slightly affects BP imaging between −80 and −150 km, during which the slip in FFI appears 1–5 s earlier than High-Freq radiators. The overall south rupture speed resolved by SEBP is ~3.11 km/s, which also indicates a subshear rupture. A secondary BP energy peak appears in the power curves between 120 and 140 s, after the trough at 100 s (Fig. 3a , Supplementary Movies 2 – 5 ). This peak corresponds to the radiators in the Eastern Mediterranean Sea (Fig. 1 a, b) and could be generated by an immediate aftershock or a subevent on the offshore part of the Karasu fault, close to the Cyprus arc (see Rupture Speed and Extent section for more discussion).
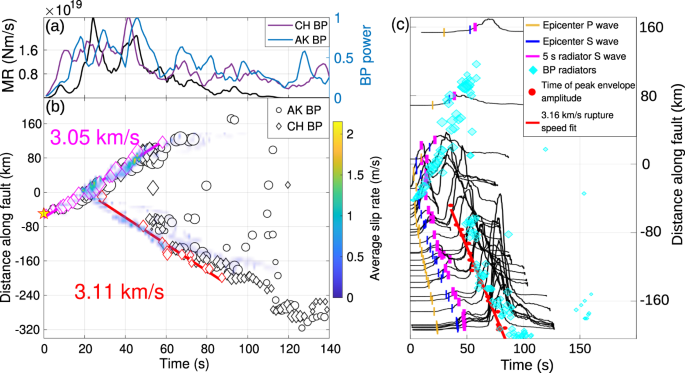
a The moment rate function (MR, black line) and the BP power for SEBP results of CH array (purple line) and AK array (blue line). b Along-fault distance and time of BP radiators and slip rates. The junction of the splay fault and the main fault is set as the origin of the distance axis, and the northeast is the positive direction. The origin time of the Mw 7.8 event is set as the origin of the time axis. Unless otherwise stated, subsequent references to distance and time use this definition. The diamonds and circles denote the High-Freq radiators imaged by the CH and AK arrays, respectively. The colormap denotes the slip rate averaged across depth. The red and magenta slants show the fitted rupture speeds for the SW and NE fronts delineated by High-Freq radiators, respectively. c Vertically exaggerated envelopes of detrended, demeaned, bandpass filtered (1–4 Hz) fault-parallel components of strong motion stations shown in Fig. 1a . The red line indicates the SW speed fit using the timing and distance of second envelope peaks at southern stations. The red dots indicate the time at which these peaks occur for a particular station’s distance along the fault.
The SEBP results are further validated by local strong motion recordings (Fig. 3c ). The recordings show two pulses of energy that form easily distinguishable peaks at most displayed stations. The first pulse attenuates with distance from the epicenter as expected from the geometrical spreading of seismic waves. We trace S-phases radiated from selected High-Freq radiators obtained from the BP results of the CH array to test whether the arrival times of these S-phases at station locations coincide with time frames of high energy release. The magenta vertical bars indicating the theoretical S-wave arrival from the 5 s High-Freq radiator (the diamond with a magenta edge in Fig. 1a ) coincide well with this first energy pulse. However, the second pulse of energy does not attenuate with distance. In Fig. 3c , we notice an abundance of High-Freq radiators, indicated by the cyan diamonds, at southern stations at the time of the second energy pulse. They correspond to the SW-ward rupture front passing through these stations along the Amonos segment. The amplitude of the second pulse of energy is thus maintained by the contribution of source areas closest to each station. We do not see two envelopes of energy at stations in the north, which we attribute to the rupture pattern observed from SEBP: the north rupture travels at speed comparable to the local shear wave speed (Vs = 3.39 km/s, ref. 3 ), so that S waves from the hypocenter and the rupture front arrive near northern stations almost simultaneously. In contrast, the south stations see two envelope peaks separately because the SW rupture starts at least 15 s after the earthquake’s initiation and north from the epicenter.
For the SEBP of the Mw 7.5 event (Fig. 1 and Supplementary Fig. 10 , Supplementary Movies 6 – 9 ), the CH array result shows that the rupture propagates bilaterally along the East-West trending Sürgü Fault. Further east, the rupture traveled along a curved path, which corresponds well with bends seen in the fault mapping of the Sürgü Fault Zone 14 . The rupture fronts then terminate at ~65 km east and west of the epicenter, spanning a rupture length of ~130 km, which is also close to the length estimated from field and satellite data 1 . The rupture speeds are ~2 km/s eastward and ~3.6 km/s westward. The rupture seems to stagnate in the first 8 s of the event, likely due to the aforementioned signal interference in bilateral ruptures 13 . The westward rupture speed of 3.6 km/s is very close to the local shear wave speed of 3.3–3.5 km/s (refs. 3 , 15 ), and supershear rupture might occur on parts of the west Sürgü Fault. Faster western rupture is reported in the slip model by Melgar et al. 16 , in which a maximum rupture speed of 4.8 km/s is preferred.
Rupture speed and extent of the mainshock
The Mw 7.8 event ruptured multiple faults and involved a complex propagation process. A key question is whether the rupture speeds during the mainshock exceed local shear wave speed (Vs). Supershear ruptures, which propagate faster than Vs, can generate stronger ground motion 17 and cause more severe damage than subshear ones. Supposing a local Vs of 3.39 km/s at 4–12 km depth where most slip occurs 3 , the overall speeds for the northeast (V NE = 3.05 km/s) and southwest (V SW = 3.11 km/s) are both subshear. Contemporary studies show highly debated rupture speeds (Supplementary Table 2 ). Mai et al. 18 perform beam-forming BP and report a V NE of 3.1 km/s and a V SW of 2 km/s. Petersen et al. 19 utilize finite source inversion and BP techniques, revealing an average velocity range of 1.8–2.6 km/s and a maximum velocity of 3.4 km/s. Yao & Yang 20 determine a V SW range of 3.1–3.4 km/s and a V NE of ~3.19 km/s through near-field data analysis and numerical rupture simulations. Melgar et al. 16 conduct FFI based on high-rate GNSS and local strong-motion data, identifying a preferred rupture speed of 3.2 km/s for both the northeast and southwest branches. Delouis et al. 21 perform finite source inversion and find that the rupture speeds on the EAF are globally subshear, with several transiently supershear fault portions. Apart from Delouis et al. 21 , supershear ruptures are also declared in some studies, ranging from 5.1 km/s at the splay fault 22 to 6 km/s at the southwest EAF 23 . Rosakis et al. 22 analyze three strong motion stations near the splay fault and identify that the rupture propagates at speed near 1.55 Vs (~5.1 km/s) on the NF after traveling 19.45 km at subshear speeds. Okuwaki et al. 23 perform a potency-density tensor inversion and find a 5–6 km/s speed for the southwest branch of the rupture. Wang et al. 24 simulate the dynamic rupture process of the mainshock, and report that V NE is supershear while V SW varies repeatedly between supershear and subshear. Abdelmeguid et al. 25 report the occurrence of supershear ruptures at the end of the southwest segment and the northeast segment of the EAF based on their observation of larger fault-parallel amplitudes compared to fault-normal amplitudes in near-field seismic recordings. However, it should be noted that the interpretation of component amplitudes alone as strong evidence for supershear ruptures is a subject of debate 21 .
We resolve the discrepancy in reported rupture speeds between our results and other studies by showing that Mach wave signatures of supershear rupture are absent in Rayleigh waves recorded globally (Methods). Long and persistent ruptures propagating faster than Rayleigh and Love waves produce surface wave Mach cones with distinguishing signatures in seismograms. At stations located near a pair of azimuths around the rupture direction, surface waves emitted by all portions of the source arrive simultaneously and interfere constructively 26 , 27 , leading to waveform shapes highly similar to those of a nearby smaller event with shorter rupture duration (e.g., an M5-6 aftershock or foreshock). The waveform amplitude ratio between the mainshock and the smaller event should equal their seismic moment ratio. Figure 4a, b shows the cross-correlation coefficients (CCs) between the 15–25 s period mainshock waveforms and a nearby M5.3 aftershock (Supplementary Table 3 ) waveforms, and Fig. 4c, d shows their amplitude ratios over moment ratios. We find that the azimuthal distributions of CCs and amplitude ratios are distinct from those predicted by a supershear rupture. In simulations 28 and observations of supershear events 26 , 27 , the CC and amplitude ratios feature two maxima at azimuths of 25°–50° relative to the rupture direction and a local minimum at the rupture direction. But here the CC and amplitude ratios peak at the two rupture directions rather than on their sides. This distribution pattern is consistent with simulation results of a subshear rupture traveling at Rayleigh wave speed (V Rayleigh = 0.92 Vs) (ref. 28 ). For the southwest rupture, several stations record highly-similar waveforms (CCs > 0.9; Fig. 4a and Supplementary Fig. 16 ), indicating a rupture speed around V Rayleigh . Using a local Vs of 3.39 km/s and V Rayleigh = 0.92 Vs, the inferred rupture speed of 3.12 km/s remarkably matches our SEBP results (3.11 km/s). For the northeast rupture, the maximum CCs are slightly below 0.9, indicating its speed should be marginally lower than V Rayleigh , consistent with our SEBP results.
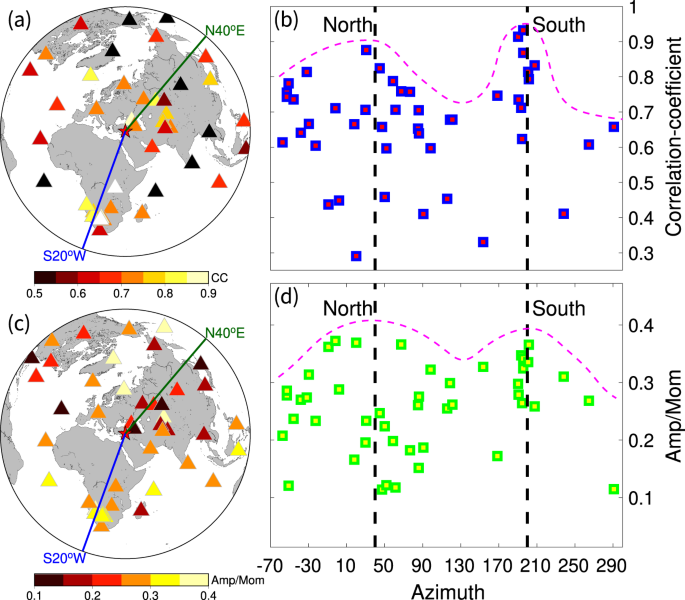
a Spatial distribution of stations. Triangles denote the stations used for analysis, color-coded by the cross-correlation coefficients (CCs) between 15 to 25 s Rayleigh waves of the mainshock waveforms and its M5.3 aftershock (Supplementary Table 3 ) waveforms. The dark green line and word indicate the direction of the northeast rupture. The blue line and word indicate the direction of the southwest direction. b CCs distribution as a function of the station azimuth. The vertical black dashed lines indicate the northeast and southwest rupture directions. The pink dash curve indicates the envelope delineating the distribution pattern of CCs. c Spatial distribution of stations. Triangles denote the stations used for analysis, color-coded by [waveform amplitude ratio]/[seismic moment ratio] between the mainshock and the aftershock. d [Amplitude ratio]/[moment ratio] distribution as a function of the station azimuth.
The rupture process of the mainshock involves bilateral rupture propagation across multiple phases. To examine the effects of this bilateral rupture and the rupture kink on the cross-correlation coefficient of the empirical Green’s function (EGF) and mainshock waveform, we conduct synthetic tests (Methods, Supplementary Figs. 17 and 18 ). Based on the results of our bilateral rupture synthetic tests, it is observed that when a supershear rupture occurs, there is a distinct peak couple of CCs and amplitude ratios observed at specific azimuths around the strike of the supershear branch (Supplementary Fig. 17 c, d, f, g). These findings are consistent with observations from previous supershear earthquakes such as the 2017 Komandorski and the 2016 Romanche events, where similar peak couples were observed within Mach cones 28 . The synthetic waveform’s amplitude contributed by the subshear branch is found to be low, indicating its negligible influence on the observation of Mach waves and Mach cones. The results from the third synthetic case (Supplementary Fig. 17h–j ) where rupture speeds are set to be identical to those resolved by our SEBP (V NE = 0.9Vs and V SW = 0.92Vs) align with the observations: when both V NE and V SW are close to the Rayleigh wave speed, one peak of CCs and amplitude ratios appears at the northeast rupture strike, and another one occurs at the southwest rupture strike (Fig. 4 , Supplementary Fig. 17i, j ). The consistency between the observed and synthetic CCs and amplitude ratio distributions further verify our conclusion that both V NE and V SW are close to the Rayleigh wave speeds and are overall subshear. Additionally, we conduct a synthetic test to simulate a supershear rupture with a 20° kink on the northeast of the EAF (Supplementary Fig. 18 ). The results indicate that the presence of a small angle kink has little impact on the distribution patterns of CC and amplitude ratio, and the Mach cone pair remains clearly identifiable. The results of our synthetic tests confirm the overall subshear speeds observed by our SEBP and joint FFI. However, we acknowledge the possibility of local and transient supershear behavior, as found by Delouis et al. 21 . For supershear ruptures with lengths comparable to the fault width, Mach waves could not be identified due to the long-period nature of surface waves 26 .
The small event corresponding to the secondary BP power peak and occurring around 120 s after the origin time (Fig. 3b ) could be a subevent of the mainshock on the offshore portion of the Karasu Fault or an immediately triggered aftershock. Similar short-period emissions are also observed in 108–117 s of BP results by Petersen et al. 19 and are identified as the last stage of the mainshock by them. We observe scattered and discontinuous BP radiators and a minimum BP power during 90–120 s (Figs. 1 and 3a ), as well as an offshore aftershock gap near Karasu Fault and the coast (Fig. 1a ). In contrast, many aftershocks concentrate near the southern end of the Amanos segment. Since aftershocks tend to happen on the edge of major coseismic slip areas to release the increased stress caused by the mainshock 29 , 30 , we interpret that the mainshock rupture ended at the Amanos segment and the offshore event is a triggered aftershock. To further validate our hypothesis, we calculate the theoretical S wave travel time from the last High-Freq radiator on the Amanos segment to the first radiator in the sea (Supplementary Fig. 19 ) using the local velocity model from Guvercin et al. 3 . The theoretical travel time (30.84 s) matches the time interval between these two radiators (31.33 s) very well. The time consistency suggests that the offshore event was dynamically triggered by the S wave emitted by the stopping phase at the south end of the mainshock rupture.
Earthquake supercycle
During the Mw 7.8 event, most of the slip and seismic moment took place on the Pazarcık, Amanos, and Erkenek segments of the EAF (Supplementary Fig. 15 ). During the instrumental period between 2007 and 2020, these segments had far less seismic activity than the northeast part of the EAF 3 . Two major patches with coseismic slip amplitude greater than 8 m on the Pazarcık and Erkenek segments (Fig. 2 ) coincide with the seismicity voids on them (Supplementary Fig. 20 ). Ambraseys 4 investigated the historical earthquakes in SE Turkey and reported temporary seismic quiescence in the 20th century, though violent events occurred within the preceding few centuries. The lack of contemporary seismicity on the main fault contributes to the stress accumulation and gives the main fault potential to host large coseismic slip during earthquakes. Historical documents and field investigations demonstrate that the most recent events on or near the Pazarcık segment occurred in 1114, 1513, and 1795, with magnitudes of 7.8+, 7.4, and 7.0, respectively (Fig. 5b ; refs. 4 , 5 , 6 ). On the Amanos segment, researchers have identified an ~M7 event in 601, an M7.5 event in 1822, and an M7.2 event in 1872 (Fig. 5b ) (ref. 5 ). On the Pazarcık and Amanos segments, the time intervals since the reported last large earthquakes are 218 and 141 years, respectively. The long-term slip rates inferred from GPS data are 7 mm/year and 3 mm/year, respectively 31 , 32 . The maximum earthquake magnitudes are 7.0–7.3 every 237–772 years (Pazarcık segment) and 7.2–7.4 every 414–915 years (Amanos segment) 3 .
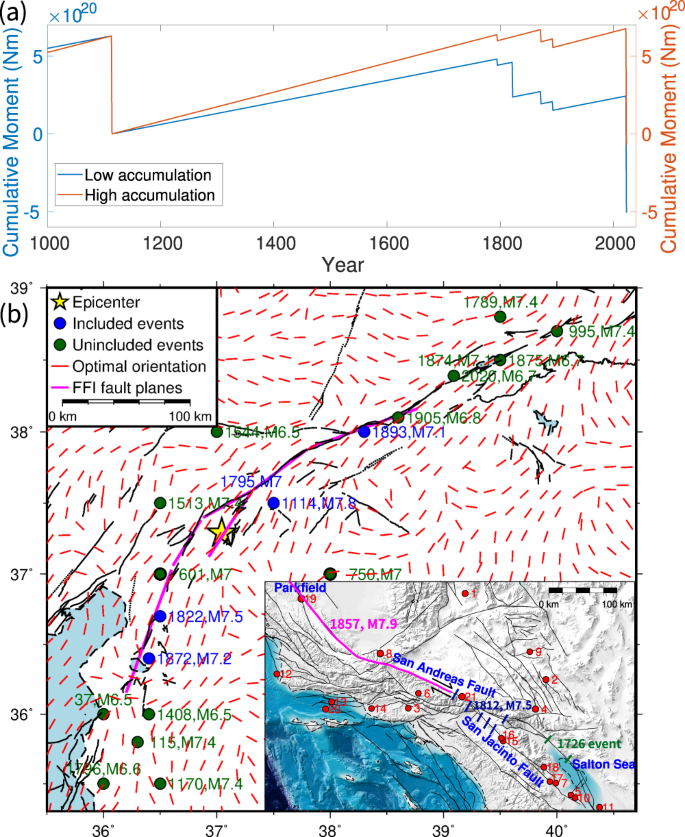
a History of potential moment accumulation on the EAF segments involved in the Mw 7.8 mainshock. The blue curve is the moment history of the low accumulation case, and the orange curve is the moment history of the high accumulation case. See text for details. The last big drop is the moment released by the 2023 Mw 7.85 event. b Map of historic earthquakes 4 , 5 , 6 , 8 and optimal slip orientation. The blue circles and numbers indicate the location, year and magnitude of historic earthquakes close to the faults, and included in the moment history in a . The dark-green circles denote historic events far from the FFI fault planes and are not included in a . The red bars indicate the optimal slip orientation computed based on the regional strain rate field 49 by assuming a rock friction coefficient of 0.6 and the local strain direction being the same as the regional horizontal strain rate direction. The lower inset shows the geometry of San Andreas Fault and San Jacinto Fault and historical events. The black arrows near San Andreas Fault indicate the relative motion of two blocks. Dark blue bars represent the paleoseismic sites of the 1812 earthquake, adapted from Lozos 58 . Dark green bars represent the paleoseismic sites of the latest 1726 earthquake, adapted from refs. 52 , 53 . The pink line denotes the ruptured fault during the 1857 M7.9 event 39 . Red dots and indices denote all M ≥ 6.5 events since 1812. Information of events 1–21 in the lower inset can be found in Supplementary Table 6 .
During the Mw 7.8 earthquake, the equivalent coseismic Mw on the Pazarcık and Amanos segments are both greater than 7.49 (Supplementary Fig. 15 ). Such large magnitudes, released within relatively short time periods since the last large earthquakes (100–200 years), exceed the expectation based on the characteristic earthquake cycle model. The pattern can be explained within the framework of the earthquake supercycle model on segmented faults, in which a series of events breaking fault segments separately can be followed by a larger earthquake that breaks multiple segments at once 33 . In the supercycle model, the magnitude of one earthquake is not only determined by the cumulative strain since the last earthquake, but also related to the residual stresses left by earlier events 34 . Such supercycle models might also feature clusters of events separated by quiescence periods 35 . Supercycle behaviors have been identified on the San Andreas Fault, the Sumatra subduction zone, the Cascadia margin, and the Tohoku region 34 , 36 , 37 , 38 , 39 , and could be common, as suggested by Wallace 35 .
The latest very large earthquake (M ≥ 7.8) on the EAF before 2023 took place in 1114 (ref. 5 ), demonstrating a possible supercycle return period of ~900 years. To evaluate the validity of the 900-year period, we compute the seismic moment accumulation history since 1114 and check if the balance could be achieved between build-ups and releases. We first compute the moment build-up rates on the Amanos, Pazarcık, and Erkenek segments where coseismic slip occurred during the Mw 7.8 mainshock. The moment build-up rate: m’=μ·L·H·r, where μ is the rock rigidity inferred from the 1D model 3 , L is the segment length, H is the seismogenic zone depth, and r is the long-term fault slip rates. We set H as 20 km based on the coseismic slip distribution in the preferred model and the seismicity distribution presented by Güvercin et al. 3 . The long-term slip rates are from Duman and Emre 32 , and the segment lengths are determined according to our FFI fault planes and fault maps from Duman and Emre 32 (Supplementary Table 4 ).
We assume all moment build-ups are released seismically. For major earthquakes (M ≥ 7), historical investigations 4 , 5 provide information on their magnitudes, locations, and rupture lengths (Supplementary Table 5 ). Assuming these catalogs are complete, we can compute the moment release by those major events. As depicted in Fig. 5b and Supplementary Table 5 , the events in 1872 (M7.2), 1795 (M7), and 1893 (M7.1) are believed to have occurred on the Amanos, Pazarcık, and Erkenek segments, respectively (refs. 3 , 4 , 5 ). The 1513 event (M7.4) is likely to have taken place on a secondary fault rather than the EAF 4 . The occurrence of the 1822 event (M7.5) on the EAF 4 remains a subject of debate due to the absence of fresh fault-related topographical features 32 . On the other hand, it is hard for these studies to include all smaller events (M < 7) before the instrumental period. We utilize the Gutenberg–Richter (G-R) law 40 to evaluate the seismicity rates of events between Mw 1–7 (excluding 7). These background seismicity rates are influenced by the b values in the G-R relations, where a lower b value corresponds to a higher rate. Güvercin et al. 3 reports two sets of b values, differing by approximately 0.2. Thus, we explore two scenarios: a low accumulation case with b values around 0.9 (ref. 3 ), and a high accumulation case with b values around 1.1 (ref. 3 ; see Table 1 and Supplementary Table 4 ). Concurrently, we include the previously mentioned 1822 event (M7.5) in the low accumulation case but exclude it from the high accumulation case (Table 1 ). Our calculations show that in the low accumulation scenario, the net moment accumulation between 1114 and 2022 is approximately 32% of the coseismic moment of the 2023 Mw 7.85 mainshock (Fig. 5a ), which is equivalent to an Mw 7.52 event. In the high accumulation case, the percentage and equivalent Mw are 90% and 7.82, respectively. Although these estimates are subject to many uncertainties, such as historic earthquake magnitude estimations 41 and temporal variations of geodetic slip rates 42 , 43 , it suggests that the 900-year period is close to the low bound of the supercycle duration. Additional estimates of the interseismic coupling rate and the fraction of moment released seismically 44 are required to explore the upper bound of the super-cycle duration. A contemporary study 45 also conducted similar moment calculations and estimated cycle lengths, employing lower rates of geodetic strain accumulation and a smaller dataset of historical events. They also observe that the accumulated moment since 1114 is insufficient to account for the Mw 7.8 event in 2023 and attribute this deficit to potential overestimations of historical events.
Nucleation on splay faults and implication for San Andreas-San Jacinto Fault system
SEBP and FFI results show that the rupture initiates on the splay Narlı Fault and continues on the main EAF. Similar phenomena have been observed in other fault systems. The 2001 Kokoxili earthquake 46 , the 2002 Mw 7.9 Denali 47 , and the 2016 Mw 7.8 Kaikoura 48 earthquakes also nucleated on secondary faults and continued onto the main faults. The reason why Mw 7.8 Kahramanmaraş earthquake started on the splay NF deserves investigation. One possibility is that current stress fields make the NF more favorable for earthquake nucleation, which is supported by more abundant seismicity observed on the NF than on its nearby EAF between 2007 and 2020 (Supplementary Fig. 20 ; ref. 3 ). To validate the hypothesis, we calculate the optimal orientation for the failure planes on and near EAF (Fig. 5b ) based on the strain rate field 49 , assuming a rock friction coefficient of 0.6 and that the strain shares the same direction as the horizontal strain rate. Near the hypocenter, the optimal orientations align better with the strike of the NF than that of the Pazarcık segment. This is consistent with the active seismicity on the NF 3 . The regional optimal failure orientations also align well with the strike of the Amanos and the Pütürge segments of the EAF, but the former shows quiescent seismicity, and the latter is seismically active. This contrast might be attributed to the discrepancy in long-term slip rates. From the east to the west along the EAF, the slip rates substantially decrease from 10 mm/yr to 1–4 mm/yr.
There is a high resemblance between the San Andreas Fault (SAF) and the EAF systems (Fig. 5b and Supplementary Fig. 21 ) as similar main fault-splay fault geometry is observed at many sites along the SAF, such as its junction with the San Jacinto Fault (SJF), the San Gabriel Fault, and the San Gregorio Fault (Supplementary Fig. 22 ). Among them, the SJF has the largest slip rate thus should be paid close attention. At Cajon Pass, the N40-45°W oriented SJF branches out from the old and mature SAF, which strikes ~N60°W at this site. Both the SJF and NF are relatively immature compared to the main faults (the SAF and EAF), and feature many stepovers 32 , 50 and dense off-fault seismicity 3 , 51 . The long-term slip rates on the SAF (19–34 mm/year) and SJF (16 mm/year; ref. 43 ) are much higher than on the EAF (10 mm/year), and there has been a lack of major earthquakes (M > 7) on the ~500-km-long SAF segment from Parkfield to the Salton Sea since 1857 (the lower inset in Fig. 5b ). In the southernmost section of the SAF, specifically near the Salton Sea and Palm Springs, the most recent surface ruptures are attributed to the 1726 earthquake (Fig. 5b ; refs. 52 , 53 ). On the SJF further south of the 1812 event, the time elapsed since the last great earthquake (Mw >7, Supplementary Table 6 ) is also more than 250 years 39 , 54 , 55 , which is longer than the time interval between the 2023 Mw 7.8 event and the 1800s ones on the EAF. An ~1000 years supercycle on the SAF was proposed based on observations at Wrightwood site, where the strain accumulation is currently approaching the highest level seen in the past 1500 years 39 . The recent Kahramanmaraş earthquakes, therefore, have important implications for California. Resemblant to the NF in Turkey, the SJF also has very active seismicity 51 and the rupture on SJF could potentially trigger the rupture on the SAF. In fact, the 1812 M7.5 San Juan Capistrano earthquake on the SJF and SAF might to some extent resemble the rupture propagation process of the Mw 7.8 Kahramanmaraş earthquake: surface ruptures in the early 1800s are identified along the SAF and SJF 56 , 57 , and a dynamic rupture model built by Lozos 58 shows a plausible scenario in which the rupture began near Mystic Lake on the south SJF, and propagated towards the northwest. After arriving at the junction of SJF and SAF, the rupture jumped through the stepover and went further northwest along SAF. If a “Kahramanmaraş earthquake” scenario happens: a rupture starts on the SJF and branches bilaterally on the SAF and releases most moment accumulation at once, the higher slip rate and longer time interval suggest a potentially more severe earthquake in California, with a magnitude of ~8. In-depth investigations into fault interactions during the 2023 Mw 7.8 Kahramanmaraş earthquake sequence will yield valuable insights applicable to the SAF and other major fault systems. One aspect worth exploring is how the rupture on the splay NF triggers the bilateral failure on the EAF. This investigation is particularly relevant because the NF and the southwest EAF have an orientation difference of only approximately 28°. Consequently, slip on the NF is expected to decrease the static Coulomb failure stress on the southwest EAF, creating a “stress shadow” that hinders fault failure 59 . Detailed examinations are needed to understand how the southwest rupture surpasses this stress shadow and its correlation with the background stress conditions. Such inspections will contribute to a better estimation and mitigation of seismic hazard risks associated with the global strike-slip faults.
SAR data processing
We apply speckle tracking to the Sentinel-1 SAR images for the surface deformation in slant range (radar line-of-sight, or cross-track) and ground azimuth (along-track) directions via the InSAR Scientific Computing Environment (ISCE) version 2 software 60 using the “topsApp.py” application 61 (Supplementary Fig. 6a–f ). The two level 1 Single Look Complex (SLC) data are coregistered with each other using the geometrical co-registration with refinement from the enhanced spectral diversity technique 62 . Then we applied the speckle tracking (also known as pixel tracking, amplitude cross-correlation) analysis to pairs of Sentinel-1 SLC images (detailed pairs information are listed in Supplementary Table 7 ) to estimate the coseismic surface deformation. We oversampled the coregistered SLC by a factor of 32, used a cross-correlation window or chip with a relatively large size of 128 pixels in range direction and 64 pixels in azimuth direction (about 585 ×900 m on the ground) to reduce the estimation noise 63 . To further reduce the offset estimation noise, we applied a median filter with a ground size of 1.5 ×2.2 km; masked out pixels on the water body or with abnormal offset value (>5 m); for range offsets masked out pixels with offset standard deviation (STD) > 0.5 m, and for azimuth offsets masked out pixels with offset STD > 3.0 m. Then we geocoded all offsets to a grid in size of 0.00277778° (~300 m) in latitude and longitude.
We apply InSAR and MAI to the ALOS-2 ScanSAR images for the surface deformation in slant range and ground azimuth directions via the ISCE-2 software 60 using the “alos2App.py” and “alos2burstApp.py” applications 64 , 65 , 66 (Supplementary Fig. 6g–j ); and apply InSAR to the LuTan-1 stripmap images for the surface deformation in the slant range direction via a combination of Gamma 67 and ISCE-2 60 software packages (Supplementary Fig. 6k ). For all interferograms from ALOS-2 and LuTan-1, we use the SRTM DEM 68 (SRTMGL1, 1 arc second with void-filled, ~30 m), apply the Goldstein filter with a strength of 0.8, unwrap the interferogram using the minimum cost flow method 69 with near-fault pixels masked out using the surface rupture traces from Sentinel-1 speckle tracking 1 . For ALOS-2 InSAR, we multilook the interferogram by 5 and 28 looks in range and azimuth directions, respectively; correct for ionospheric delay using the range split-spectrum technique 66 ; geocode into a grid in size of 0.000833334° (~90 m; Supplementary Fig. 23 a, d). For LuTan-1 InSAR, we coregister the single look complex (SLC) images using DEM and precise orbits with refinements from cross correlation, multilook the interferogram by 24 and 28 looks in range and azimuth directions, respectively; correct for phase unwrapping errors by adding an integer number of phase cycles (2 π ) to one side of the Mw 7.8 rupture using the ALOS-2 descending interferogram as reference; geocode to a grid in size of 0.00055556° (~60 m; Supplementary Fig. 24 ). The ionospheric delay is not corrected due to the expected weak impact at 6:30 am local time. For ALOS-2 MAI, we extract burst SLC images from ALOS-2 full aperture ScanSAR products. The burst SLCs are used to form burst interferograms. We then compute the MAI interferograms using the burst interferograms. We multilook the MAI interferograms by 14 and 4 looks in range and azimuth directions, respectively. Subsequent processing is the same as regular InSAR processing. The ALOS-2 ScanSAR system is a four-look burst system, so we can get three MAI interferograms with burst separations one, two and three. The final MAI result is a weighted mean of the three MAI interferograms 65 , and geocoded to a grid in size of 0.000833334° (~90 m).
For all measurements in the range direction (Sentinel-1 range offsets, ALOS-2 and LuTan-1 interferograms), we correct for tropospheric delay using the ERA5 global atmospheric model 70 via PyAPS 71 (Supplementary Fig. 23 b, e), for solid Earth tides following the 2010 IERS convention 72 via PySolid 73 (Supplementary Fig. 23 c, f).
We combine all observations above to derive the three dimensional co-seismic deformation (Supplementary Fig. 7 ), including three range offsets and three azimuth offsets from Sentinel-1, two regular interferograms and two MAI interferogram from ALOS-2, one regular interferogram from LuTan-1. Built upson Fialko et al. (ref. 74 ), we use a weighted approach to balance the different uncertainties among speckle tracking, InSAR and MAI techniques, and propagate the uncertainties into the east, north and up components for each pixel. More specifically, we use the pixelwised speckle tracking STD for Sentinel-1, a constant STD of 0.32 and 0.30 m for ALOS-2 ascending and descending MAI (estimated from the non-deforming region in the far-field) to account for the ionospheric impact, and a constant STD of 0.05 m for ALOS-2 and LuTan-1 interferograms to account for residual tropospheric delays. The estimated east, north and up components are masked using a STD threshold of 0.4, 0.8 and 0.3 m, respectively.
Joint Finite Fault Inversion
The Finite Fault Inversion (FFI) method was developed after the 1979 Imperial Valley earthquake 75 , 76 to study the rupture complexities of earthquakes. With decades of development, current FFI algorithms could include global and local data to simultaneously determine the slip amplitude, rupture time, and rise time 11 , 77 , 78 . The method has been widely applied to large earthquakes and provides important information for hazard assessment and mitigation (e.g., 2004 Sumatra earthquake 79 ; 2011 Tohoku earthquake 80 ).
We use the joint FFI to image the fault’s rupture process and slip distribution 11 , 12 . The seismic waveform and geodetic data are adopted to constrain the slip model. The seismic waveform data include 20 broadband teleseismic P waves (bandpass filtered to 2–333 s, Supplementary Fig. 1 ), 13 broadband teleseismic S waves (bandpass filtered to 2–333 s, Supplementary Fig. 1 ), 23 long-period teleseismic Rayleigh waves (bandpass filtered to 166–250 s, Supplementary Fig. 2 ), 17 long-period teleseismic Love waves (bandpass filtered to 166–250 s, Supplementary Fig. 2 ), 57 near-field strong motion recordings (P, SH, SV for 19 stations, bandpass filtered to 4–40 s and integrate to velocity seismograms, Supplementary Figs. 3 and 5 ). The geodetic data include 24 high-rate GNSS recordings (UD, EW, NS for 8 stations, displacement waveforms, Supplementary Figs. 4 and 5 ), static ground displacements derived from Sentinel-1 SAR data, ALOS-2 and LuTan-1 InSAR and MAI data (Fig. 1 a, b, Supplementary Figs. 6 – 8 ; see SAR Data Processing for more details). The combination of multiple datasets could provide better constraints on the slip amplitude, slip distribution, rupture speed, and rise time than any single dataset 81 , 82 . The seismic and high-rate GNSS data are transformed into wavelet domains. Compared with conventional inversion only in frequency or time domain, the wavelet transform allows us to simultaneously capture the characteristics in the high-frequency and low-frequency and preserve the information in the time-domain 11 , 12 . The misfits for static ground deformation measured by SAR, InSAR and MAI are calculated separately since they could not be included in the wavelet transform. We use the weighted sum-squared residuals to measure the difference between observed and synthetic data. The weights are inversely proportional to the standard deviation at each data point. We identify the fault surface traces from the SAR images. Six vertical fault planes (segments S1–S6 in Fig. 1b ) are set to model the rupture. We parameterize the fault planes with 45 and 7 subfaults in the along strike and dip directions, respectively. We adopt the Laplacian smoothing constraints for fault slip 11 and perturbations of rupture initiation time at individual subfaults 83 . The subfault size is 9 km (along strike) by 3 km (downdip; Supplementary Fig. 12 ). The hypocenter is set at 37.218°N, 37.007°E, 7.5 km depth (adapted from a relocated hypocenter shared by Sezim Ezgi Güvercin on Twitter). The rupture velocity is allowed between 1.5 km/s to 4.9 km/s with a reference speed of 3.0 km/s, and the rake angle is allowed ±30° from the reference angle of 11° provided by Global Centroid Moment Tensor Catalog. The Green’s functions for local strong motion waveforms and static displacements are computed using the f-k integration approach by Zhu and Rivera 84 and the 1-D crust model by Güvercin et al. 3 . Subfaults are interpolated to 1 km × 1 km when plotting, to improve the visualization (Fig. 2 and Supplementary Fig. 13 ).
Slowness-Enhanced Back-Projection (SEBP) and validation
A method that allows for clear source images to be obtained as soon as data is available and without accurate fault parameters is back-projection (BP). This source imaging technique tracks the propagation of earthquake rupture fronts based on coherent seismic recordings from dense networks of seismometers 85 . Results of BP show the spatiotemporal evolution of ~1 Hz radiators and are useful in obtaining rupture parameters such as length and speed 86 , 87 .
In our study, we use the Multitaper-MUltiple SIgnal Classification (MUSIC) type of BP enhanced by the use of ‘reference windows’. This removes an apparent drift in the radiators towards stations in the array that would otherwise be displayed in the image results, allowing for sharper images to be obtained 88 . In standard BP, 1-dimensional velocity models (e.g., IASP91, ref. 89 ) are used to obtain travel times of P-waves from the mainshock hypocenter to station locations. In reality, these travel times deviate from those predicted using a 1D model due to ray path effects caused by Earth’s 3D structure. To account for this, BP corrects the travel times using the hypocenter alignment method 86 , 90 . This method assumes that the first arrival on observed seismograms comes from the hypocenter location obtained by a local catalog (Disaster and Emergency Management Authority of Turkey; AFAD for our application) and then cross-correlates the first 8 s of the P-wave recordings. The key assumption of this standard approach is that these travel time errors are uniform over the whole rupture area, which is only valid for locations close to the hypocenter.
To accurately account for travel time errors further away from the hypocenter, the BP method we use utilizes the spatial derivatives of travel times (slowness) with respect to source locations for a given station, and is hereafter referred to as Slowness-Enhanced back-projection (SEBP 10 ). This additional correction term is computed using locations of aftershocks within the rupture area. For each aftershock, the travel times relative to the hypocenter are compared with the predictions without the slowness correction term. Then, any differences are mapped to the slowness correction term by dividing them by the distance between the mainshock and the aftershock. Several studies have shown that SEBP provides more precise rupture location and speed estimates 10 , 27 , 91 , 92 .
We collect the vertical components of the P wave broadband seismograms from two large-aperture arrays at teleseismic distance, located in China (CH) and Alaska (AK, the lower inset in Fig. 1a ). The regular-size AK array contains ~200 stations, and the frequency band with high resolution and enough signal-to-noise ratio (SNR) is 0.5–2 Hz. The vast CH array includes more than 700 stations available for BP, which ensure high SNR in 1–4 Hz BP and provide better resolution than regular-size arrays. For the SEBP of the Mw 7.8 event, 718 seismograms from the CH array in 1–4 Hz and 182 seismograms from the AK array in 0.5–2 Hz are included. For the SEBP of the Mw 7.5 event, we process 719 and 153 seismograms from the CH and AK arrays, respectively. We carried out SEBP with a 10-s-long sliding window with a 1-s-long step. Slowness correction terms are derived using 11 and 7 nearby aftershocks for the CH and AK applications to the Mw 7.8 event, with 6 and 5 aftershocks for the CH and AK array applications to the Mw 7.5 event, respectively. The mainshock and aftershocks’ hypocenter locations and magnitudes based on the AFAD catalog are shown in Supplementary Tables 1a–d , along with comparisons of our aftershock locations before and after slowness calibration (Supplementary Figs. 9 and 10 ). The root-mean-square distance error relative to the AFAD locations decreases by at least 61% after calibration for all our applications.
The dense network of accelerometer stations along the Pazarcık and Amanos segments (white triangles in Fig. 1a ) provides us with a good benchmark to validate the SEBP results. We collect recordings from 33 near-field accelerometer stations. After detrending and demeaning accelerometer recordings, we bandpass-filter them using a frequency band of 1–4 Hz to match the BP band of the CH array application. We then calculate and smooth the envelopes of each recording using the Hilbert transform method. We do not include several northern stations on our plot in Fig. 3c since, at the time of this study, they did not have available data in the time window of interest. We trace S-phases radiated from the fixed High-Freq radiators obtained from CH array BP results (Fig. 1a ) to test whether the arrival times of these S-phases at station locations coincide with time frames of high energy release. We use the TauP toolkit for ray tracing, where we assumed a 1-D velocity model from Guvercin et al. 3 . Since that velocity model does not provide a Moho depth estimate, we adopt a Moho depth of 37 km from the CRUST1.0 global velocity model at the location of the mainshock. The High-Freq radiators resolved by CH array BP are also plotted in Fig. 3c according to their along-fault distance and time.
Mach wave searching and synthetic tests
For ruptures propagating at speeds lower than Rayleigh and Love waves speeds, surface waves generated by all portions of the source arrive at far-field stations at different time. If the rupture speeds ( V r) increase to faster than Rayleigh and Love speeds, at stations located near a pair of azimuths around the rupture direction, surface waves emitted by all portions of the source arrive simultaneously and interfere constructively 26 . The azimuth pair is called Mach cones, and the surface waves recorded within Mach cones are Mach waves. Due to the simultaneous arrival within Mach cones, the waves from all sub-sources interfere constructively. Theory predicts that the shapes of bandpassed seismograms should be identical or highly similar to those from a smaller reference event (hereinafter referred to as EGF event) with analogous focal mechanisms 26 . The EGF event usually has a magnitude of 5–6. The amplitude ratios of waveforms between the large supershear and EGF events equal their seismic moment ratios. Since the Rayleigh and Love waves have speeds very close to the shear wave speeds ( V Rayleigh = 0.92 V s), Mach waves and Mach cones are also believed to be unique features of supershear ruptures.
The similarities between waveforms are measured by standard cross-correlation coefficients (CCs): CC(U,u) = \({\sum }_{i=1}^{N}{U}_{i}{u}_{i}/\sqrt{{\sum }_{i=1}^{N}{U}_{i}{U}_{i}{\sum }_{i=1}^{N}{u}_{i}{u}_{i}}\) , where U and u are the mainshock and EGF event displacement waveforms, respectively. We use Φ to denote the angle between the rupture direction and the source-station azimuth. Theory predicts Mach cones at Φ M : Φ M = arccos(V Rayleigh /V r ) , where V Rayleigh is the Rayleigh wave speed and V r is the rupture speed. The Mach angle is then positively related to V r. For the rupture with a speed of V Rayleigh , the Mach cone appears in the frontal direction of the rupture with Φ M =0. When Vr increases to 1.5 V Rayleigh (1.38 V s), Φ M increases to 49°.
We collect 46 vertical components of broadband seismic recordings (BHZ). To limit the effect of surface wave dispersion, seismograms are bandpass filtered to 15–25 s. The period is shorter than the mainshock duration but longer than the EGF duration, keeping the point source effect of the EGF event and the finite source effect of the mainshock rupture. In actual observation, due to the dispersion of surface waves and the source directivity effect, highly-similar waveforms can be found in an azimuth range around Φ M . The apparent source duration is T a = T 0 × D(Φ) , where D( Φ ) = 1-cos(Φ) × V r /V Rayleigh . Similar waveforms appear in angle ranges satisfying T a « T c, where T c is the center period of waves and is the 20 s in our case. Considering the possible travel time errors caused by the 3D path effect, we manually pick the EGF Rayleigh wave arrivals. We calculate the CCs in the 200–300 s window to include the entire envelope of EGF Rayleigh waveforms. Apart from CCs , we also compute the ratio: R = R amp /R mom , where R amp is the amplitude ratio between the waveforms of the mainshock and EGF event, R mom is the seismic moment ratio between the mainshock and EGF event.
To better understand how the distribution patterns of CC and R vary with rupture speeds, Bao et al. 28 performed synthetic tests to explore scenarios with different rupture speeds and present CC and R ’s distribution as a function of Φ . When V r ≈ V Rayleigh , CC and R are maximized in the rupture direction. For supershear ruptures, CC and R reach their maximum on the two Mach cones, whose Φ M varies from 25° to 50° depending on the rupture speeds. The 2023 Mw 7.8 Kahramanmaraş earthquake ruptured bilaterally, with the northeast branch striking ~N40°E and the southwest branch striking ~S20°W. The maximum peaks of CC and R appear near these two directions (Fig. 4 b, d), indicating that both branches should propagate by speeds close to V Rayleigh (see the main text for more detailed discussions).
Vallée and Dunham 26 derive the theory and present observations of Mach waves based on the assumption of a long unilateral rupture, where the rupture length is substantially greater than the fault width. Moreover, the selected stations should be in far-field, where the station epicenter distance is much larger than the rupture length. To meet the far-field assumption, 44 of our stations are located beyond 20° (>2200 km), with 2 closer stations (6–8°, approximately 660–880 km) included as well. In a compound rupture comprising segments with different rupture speeds and directions, waveforms from subshear segments interfere destructively at stations along the Mach cone, contributing minimally to the overall recordings 26 . It is confirmed by the observations that Mach waves from long and sustained supershear segments can be distinctly identified in many large strike-slip events involving multiple rupture phases. These events include the following examples: the 2001 Mw 7.8 Kokoxili earthquake, where a supershear segment is enclosed between two subshear segments 26 ; the 2016 Mw 7.1 Romanche earthquake, characterized by a westward supershear back-propagation following an eastward subshear rupture 93 ; and the 2021 Mw 7.4 Maduo earthquake, exhibiting simultaneous westward subshear and eastward supershear propagations 94 .
The rupture process of the 2023 Mw 7.8 Kahramanmaraş earthquake is highly complex, involving bilateral rupture propagation across multiple phases. To examine the effects of this bilateral rupture and the rupture kink on the cross-correlation coefficient of the empirical Green’s function (EGF) and mainshock waveform, we conduct synthetic tests. In these tests, the synthetic mainshock rupture propagation is simulated as a series of sub-sources. The waveforms of the synthetic mainshock rupture are modeled as the superposition of EGF waveforms, considering the rupture times and locations of different sub-sources. The slip distribution on the East Anatolian Fault is used to establish a bilateral rupture model (Supplementary Fig. 17a ). The northeast rupture has a strike of N50°E and a length of 140 km, while the southwest rupture has a strike of S20°W and a length of 200 km. The interval between sub-sources is 9 km, evenly distributed along the rupture path. We tested three rupture scenarios: (1) northeast rupture speed V NE = 1.2 V s, southwest rupture speed V SW = 0.7 V s; (2) V NE = 0.7 V s , V SW = 1.2 V s; (3) V NE = 0.9 V s , V SW = 0.92 V s. Case (3) represents the rupture speeds resolved by our SEBP and joint FFI (as discussed in the main text). The synthetic waveforms and EGF waveforms are filtered to 15–25 s, and we assume a Rayleigh wave speed of 0.92 V s at the center frequency (1/20 Hz). Cross-correlation coefficients are then measured between the synthetic waveforms and EGF waveforms (Supplementary Fig. 17 c, f, i ), as well as the amplitude ratios of the synthetic waveforms to the seismic moment ratios (Amp_ratio/Mom_ratio, Supplementary Fig. 17 d, g, j ). We also conduct a synthetic test to simulate a 140-km-long supershear rupture ( V r = 1.2 V s) with a 20° kink in the middle (Supplementary Fig. 18 ). This case simulates the kinked fault we observe on the northeast East Anatolian Fault.
Moment accumulation history
We include the Amanos, Pazarcik, Erkenek segments of the EAF, where the coseismic slip of the Mw 7.8 quake occurred, in the computation. The moment accumulation history consists of two parts: the moment build-up and moment release. The build-up rate:
m’=μ·L·H·r , where μ is the rock rigidity, L is the segment length, H is the seismogenic zone depth, and r is the long-term fault slip rates. We set H as 20 km based on the coseismic slip distribution in the preferred model (Fig. 2 ) and the seismicity distribution presented by Güvercin et al. 3 . The long-term slip rates are from Duman and Emre 32 . The segment lengths are determined according to our FFI fault planes and fault maps in Duman and Emre 32 (parameters are listed in Supplementary Table 4 ).
We assume all moment build-ups are released seismically and divide the release into two parts. The first part is the moment unloading by major events (M ≥ 7). Historical investigations 4 , 5 , 6 provide information about their magnitudes, epicenter locations, and rupture lengths. Assuming these catalogs are complete, we can compute the moment release by major events. However, it is hard for these studies to include all smaller events (M < 7) before the instrumental period. We utilize the Gutenberg–Richter (G-R) law 44 to evaluate the seismicity rates of events between Mw 1 and 7. The G-R law states that the number (N) of earthquakes with a magnitude larger than M over a certain period on a given fault obeys:
log 10 (N) = a − b · M , where a and b are G-R law constants determined empirically by regional seismicity data.
Güvercin et al. 3 investigated the seismicity of the EAF between 2007 and 2020. They evaluated the a and b values for each segment of EAF (listed in Supplementary Table 4 ). With these values, we can calculate the seismicity rate (R) for magnitudes 1–7 on each segment. The annual seismic moment ( Mo’ ) of earthquakes with magnitude M is then:
Mo’ = R · 10 (1.5M+9.1) .
Summing Mo’ for M1–7 earthquakes, we get their annual moment release rate on the fault. The final moment accumulation history is the moment build-up minus moment release (Fig. 5a ).
Data availability
The Copernicus Sentinel-1 data are provided by the European Space Agency and obtained from the Alaska Satellite Facility ( https://search.asf.alaska.edu/ ) via Seamless SAR Archive (SSARA), a service provided by the EarthScope Consortium. The ALOS-2 data are provided by the Japan Aerospace Exploration Agency through Sentinel Asia and the International Disaster Charter. The LuTan-1 data are provided by the China Centre for Resources Satellite Data and Application. The fault rupture traces are obtained from USGS 1 . The high-rate GNSS data are provided by TUSAGA-Aktif ( https://www.tusaga-aktif.gov.tr/ ). The strong motion data are available at the Disaster and Emergency Management Authority of Turkey (AFAD; https://tadas.afad.gov.tr/event-detail/ 15499 ). The moment tensor solutions come from the Global Centroid Moment Tensor project (CMT; http://www.globalcmt.org ). The seismic data are provided by the IRIS ( https://ds.iris.edu/wilber3/ ) and the Data Management Center of China National Seismic Network at Institute of Geophysics, China Earthquake Administration ( http://www.seisdmc.ac.cn ). The local earthquake catalog is available at AFAD ( https://deprem.afad.gov.tr/event-catalog ). The SAR data used in inversions, the station list used in Mach wave searching, SEBP results, and the FFI slip model are available at: https://doi.org/10.5281/zenodo.8402190 .
Code availability
The SAR processing is performed using the ISCE-2 software 60 , available at https://github.com/isce-framework/isce2 with postprocessing recipe available at https://doi.org/10.5281/zenodo.8098260 . The MATLAB code of SEBP is available at https://github.com/lsmeng/MUSICBP/tree/SEBP . The Python code of Mach wave analysis is available at https://github.com/lsmeng/MUSICBP/tree/MachWave . The finite fault inversion codes used in this study are available upon request.
Reitman, N. G. et al., Preliminary fault rupture mapping of the 2023 M7.8 and M7.5 Türkiye Earthquakes. Turkey Earthquake Emergency Response (2023). https://doi.org/10.5066/P985I7U2 . Accessed 16 May 2023.
Göçümlü, B. Ç. “Sağlık Bakanı Koca: 10 ilde 17 bin 929’u hekim olmak üzere 143 bin 829 personelimiz hizmet veriyor” [Health Minister Husband: 143 thousand 829 personnel, 17 thousand 929 of whom are physicians, provide service in 10 provinces] (in Turkish). Anadolu Agency. Retrieved 10 February (2023).
Güvercin, S. E., Karabulut, H., Konca, A. Ö., Doğan, U. & Ergintav, S. Active seismotectonics of the East Anatolian Fault. Geophys. J. Int. 230 , 50–69 (2022).
Google Scholar
Ambraseys, N. Temporary seismic quiescence: SE Turkey. Geophys. J. Int. 96 , 311–331 (1989).
Ambraseys, N. & Jackson, J. Faulting associated with historical and recent earthquakes in the Eastern Mediterranean region. Geophys. J. Int. 133 , 390–406 (1998).
Palutoglt, M. & Sasmaz, A. 29 November 1795 Kahramanmaras Earthquake. Southern Turkey. Bull. Mineral Res. Explor. 155 , 187–202 (2017).
Westaway, R. 1994. Present-day kinematics of the Middle East and eastern Mediterranean. J. Geophys. Res. 99 , 12071–12090 (1994).
Chen, K., Zhang, Z., Liang, C., Xue, C. & Liu, P. Kinematics and dynamics of the 24 January 2020 Mw6.7 elazig, Turkey earthquake. Earth Space Sci. 7 , 11 (2020).
Lin, X. et al. Coseismic slip distribution of the 24 January 2020 Mw 6.7 Doganyol Earthquake and in relation to the foreshock and aftershock activities. Seismol. Res. Lett. 92 , 127–139 (2020).
Meng, L., Zhang, A. & Yagi, Y. Improving back projection imaging with a novel physics-based aftershock calibration approach: a case study of the 2015 Gorkha earthquake. Geophys. Res. Lett. 43 , 628–636 (2016).
Ji, C., Wald, D. J. & Helmberger, D. V. Source description of the 1999 Hector Mine, California, Earthquake, Part I: wavelet domain inversion theory and resolution analysis. Bull. Seism. Soc. Am. 92 , 1192–1207 (2002).
Ji, C., Helmberger, D. V., Wald, D. J. & Ma, K.-F. Slip history and dynamic implications of the 1999 Chi-Chi, Taiwan, earthquake. J. Geophys. Res. Solid Earth. 108 , 2412 (2003).
Xu, L. et al. Understanding the rupture kinematics and slip model of the 2021 Mw 7.4 Maduo earthquake: a bilateral event on bifurcating faults. J. Geophys. Res. Solid Earth. 128 , e2022JB025936 (2023).
Emre, Ö. et al. Active Fault Map of Turkey with an Explanatory Text 1:1,250,000 Scale. Special Publication Series. 30 (General Directorate of Mineral Research and Exploration, 2013).
Acarel, D., Cambaz, M. D., Turhan, F., Mutlu, A. K. & Polat, R. Seismotectonics of Malatya Fault, Eastern Turkey. Open Geosci. 11 , 1098–1111 (2019).
Melgar, D. et al. Sub- and super-shear ruptures during the 2023 Mw 7.8 and Mw 7.6 earthquake doublet in SE Türkiye. Seismica 2 , 3 (2023).
Dunham, E. M. & Bhat, H. S. Attenuation of radiated ground motion and stresses from three-dimensional supershear ruptures. J. Geophys. Res. Solid Earth. 113 , B08319 (2008).
Mai, P. M. et al. The destructive earthquake doublet of 6 February 2023 in South‐Central Türkiye and Northwestern Syria: initial observations and analyses. Seism. Record 3 , 105–115 (2023).
Petersen, G. M. et al. The 2023 Southeast Türkiye seismic sequence: rupture of a complex fault network. Seism. Record 3 , 134–143 (2023).
Yao. S., & Yang. H. Rupture phase in near-fault records of the 2023 Turkey Mw 7.8 earthquake. EarthArXiv https://doi.org/10.31223/X51662 (2023).
Delouis, B., van den Ende, M., & Ampuero, J.-P. Kinematic rupture model of the February 6th 2023 Mw 7.8 Turkey earthquake from a large set of near-source strong motion records combined by GNSS offsets reveals intermittent supershear rupture. Authorea https://doi.org/10.22541/essoar.168286647.71550161/v1 (2023).
Rosakis, A., Abdelmeguid, M., & Elbanna, A. Evidence of Early Supershear Transition in the Mw 7.8 Kahramanmaras Earthquake From Near-Field Records. Preprint at https://arxiv.org/abs/2302.07214 (2023).
Okuwaki, R., Yagi, Y., Taymaz, T. & Hicks, S. P. Multi-scale rupture growth with alternating directions in a complex fault network during the 2023 south-eastern Türkiye and Syria earthquake doublet. Geophys. Res. Lett. 50 , e2023GL103480 (2023).
Wang. Z. et al. Dynamic rupture process of the Mw 7.8 Kahramanmaraş earthquake (SE Türkiye): variable rupture speed and implications for seismic hazard. Authorea https://doi.org/10.22541/essoar.168614604.40356164/v1 (2023).
Abdelmeguid, M. et al. Revealing the dynamics of the Feb 6th 2023 M7.8 Kahramanmaracs/Pazarcik Earthquake: near-field records and dynamic rupture modeling. arXiv https://doi.org/10.48550/arXiv.2305.01825 (2023).
Vallée, M. & Dunham, E. M. Observation of far-field Mach waves generated by the 2001 Kokoxili supershear earthquake. Geophys. Res. Lett. 39 , L05311 (2012).
Bao, H. et al. Early and persistent supershear rupture of the 2018 magnitude 7.5 Palu earthquake. Nat. Geosci. 12 , 200–205 (2019).
CAS Google Scholar
Bao, H. et al. Global frequency of oceanic and continental supershear earthquakes. Nat. Geosci. 15 , 942–949 (2022).
Hartzell, S. H. & Heaton, T. H. Rupture history of the 1984 Morgan Hill, California, earthquake from the inversion of strong motion records. Bull. Seism. Soc. Am. 76 , 649–674 (1986).
Mendoza, C. & Hartzell, S. H. Aftershock patterns and main shock faulting. Bull. Seism. Soc. Am. 78 , 1438–1449 (1988).
Reilinger, R. et al. GPS constraints on continental deformation in the Africa-Arabia-Eurasia continental collision zone and implications for the dynamics of plate interactions. J. geophys. Res. 111 , B05411 (2006).
Duman, T. Y. & Emre, Ö. The East Anatolian Fault: geometry, segmentation and jog characteristics. Geol. Soc. Spec. Publ. 372 , 495–529 (2013).
Philibosian, B. & Meltzner, A. J. Segmentation and supercycles: a catalog of earthquake rupture patterns from the Sumatran Sunda Megathrust and other well-studied faults worldwide. Quat. Sci. Rev. 241 , 106390 (2020).
Salditch, L. et al. Earthquake supercycles and long-term fault memory. Tectonophysics 774 , 228289 (2020).
Wallace, R. E. Grouping and migration of surface faulting and variation in slip rates on faults in the Great Basin province. Bull. Seismol. Soc. Am. 77 , 868–877 (1987).
Goldfinger, C., Ikeda, Y., Yeats, R. S. & Ren, J. Superquakes and supercycles. Seismol. Res. Lett. 84 , 24–32 (2013).
Satake, K. Geological and historical evidence of irregular recurrent earthquakes in Japan. Phil. Trans. R. Soc. A 373 , 20140375 (2015).
Sieh, K. et al. Earthquake supercycles inferred from sea-level changes recorded in the corals of West Sumatra. Science. 322 , 1674–167 (2008).
Weldon, R., Scharer, K., Fumal, T. & Biasi, G. Wrightwood and the earthquake cycle: what a long recurrence record tells us about how faults work. GSA Today. 14 , 4–10 (2004).
Gutenberg, B. & Richter, C. F. Frequency of earthquakes in California. Bull. Seism. Soc. Am. 34 , 185–188 (1944).
Kokum, M. & Özcelik, F. An example study on re-evaluation of historical earthquakes: 1789 Palu (Elazığ) earthquake, Eastern Anatolia, Turkey. Bull. Min. Res. Explor. 161 , 157–170 (2020).
Savage, J. & Prescott, W. Asthenosphere readjustment and the earthquake cycle. J. Geophys. Res. Solid Earth. 83 , 3369–3376 (1978).
Tong, X., Smith-Konter, B. & Sandwell, D. T. Is there a discrepancy between geological and geodetic slip rates along the San Andreas Fault System? J. Geophys. Res. Solid Earth. 119 , 2518–2538 (2014).
Avouac, J. P. From geodetic imaging of seismic and aseismic fault slip to dynamic modeling of the seismic cycle. Ann. Rev. Earth Planet. Sci. 43 , 233–271 (2015).
Karabulut, H., Güvercin, S. E., Hollingsworth, J. & Konca, A. Ö. Long silence on the East Anatolian Fault Zone (Southern Turkey) ends with devastating double earthquakes (6 February 2023) over a seismic gap: implications for the seismic potential in the Eastern Mediterranean region. J. Geol. Soc. 180 , jgs2023–021 (2023).
Lasserre, C. G. et al. Coseismic deformation of the 2001 Mw = 7.8 Kokoxili earthquake in Tibet, measured by synthetic aperture radar interferometry. J. Geophys. Res. 110 , B12408 (2005).
Eberhart-Phillips, D. et al. The 2002 Denali Fault earthquake, Alaska: a large magnitude, slip-partitioned event. Science. 300 , 1113–1118 (2003).
Cesca, S. et al. Complex rupture process of the Mw 7.8, 2016, Kaikoura earthquake, New Zealand, and its aftershock sequence. Earth Planet. Sci. Lett. 478 , 110–120 (2017).
Weiss, J. R. et al. High-resolution surface velocities and strain for Anatolia from Sentinel-1 InSAR and GNSS data. Geophys. Res. Lett. 47 , e2020GL087376 (2020).
Wesnousky, S. Seismological and structural evolution of strike-slip faults. Nature. 335 , 340–343 (1988).
Ross, Z. E., Hauksson, E. & Ben-Zion, Y. Abundant off-fault seismicity and orthogonal structures in the San Jacinto fault zone. Sci. Adv. 3 , e1601946 (2017).
Scharer, K. M. & Yule, D. A maximum rupture model for the Southern San Andreas and San Jacinto Faults, California, derived from paleoseismic earthquake ages: observations and limitations. Geophys. Res. Lett. 47 , e2020GL088532 (2020).
Rockwell, T. K., Meltzner, A. J. & Haaker, E. C. Dates of the two most recent surface ruptures on the Southernmost San Andreas fault recalculated by precise dating of Lake Cahuilla dry periods. Bull. Seism. Soc. Am. 108 , 2634–2649 (2018).
Fialko, Y. Interseismic strain accumulation and the earthquake potential on the southern San Andreas fault system. Nature. 441 , 968–971 (2006).
Olsen, K. B. et al. Strong shaking in Los Angeles expected from southern San Andreas earthquake. Geophys. Res. Lett. 33 , L07305 (2006).
Seitz, G., Weldon, R. II & Biasi, G. P. The Pitman Canyon paleoseismic record: a re-evaluation of southern San Andreas Fault segmentation. J. Geodyn. 24 , 129–138 (1997).
Kendrick, K. J. & Fumal, T. E. Paleoseismicity of the northern San Jacinto fault, Colton and San Bernardino, southern California; preliminary results. Geol. Soc. Am. Abstr. Prog. 37 , 559 (2005).
Lozos, J. C. A case for historic joint rupture of the San Andreas and San Jacinto faults. Sci. Adv. 2 , e1500621 (2016).
Yamashita, T. & Umeda, Y. Earthquake rupture complexity due to dynamic nucleation and interaction of subsidiary faults. Pure Appl. Geophys. 143 , 89–116 (1994).
Rosen, P. A., Gurrola, E., Sacco, G. F., & Zebker, H. The InSAR scientific computing environment. In EUSAR 2012; 9th European Conference on Synthetic Aperture Radar . Nuremberg, Germany, 23-26 April 2012, 730–733 (VDE, 2012).
Fattahi, H., Agram, P. & Simons, M. A network-based enhanced spectral diversity approach for TOPS time-series analysis. IEEE Trans. Geosci. Remote Sens. 55 , 777–786 (2017).
Yagüe-Martínez, N. et al. Interferometric processing of sentinel-1 TOPS data. IEEE Trans. Geosci. Remote Sens. 54 , 2220–2234 (2016).
De Zan, F. Accuracy of incoherent speckle tracking for circular Gaussian signals. IEEE Geosci. Remote Sens. Lett. 11 , 264–267 (2014).
Liang, C. & Fielding, E. J. Interferometry with ALOS-2 full-aperture ScanSAR data. IEEE Trans. Geosci. Remote Sens. 55 , 2739–2750 (2017a).
Liang, C. & Fielding, E. J. Measuring azimuth deformation with L-band ALOS-2 ScanSAR interferometry. IEEE Trans. Geosci. Remote Sens. 55 , 2725–2738 (2017b).
Liang, C., Liu, Z., Fielding, E. J. & Bürgmann, R. InSAR time series analysis of L-band wide-swath SAR data acquired by ALOS-2. IEEE Trans. Geosci. Remote Sens. 56 , 4492–4506 (2018).
Werner, C., Wegmüller, U., Strozzi, T., & Wiesmann, A. Gamma SAR and interferometric processing software. Proceedings of the ERS-Envisat symposium . (Gothenburg, Sweden, 2000).
Farr, T. G. et al. The shuttle radar topography mission. Rev. Geophys. 45 , 2 (2007).
Chen, C. W. & Zebker, H. A. Two-dimensional phase unwrapping with use of statistical models for cost functions in nonlinear optimization. J. Opt. Soc. Am. A 18 , 338–351 (2001).
Hersbach, H. et al. The ERA5 global reanalysis. Q. J. R. Meteorol. Soc. 146 , 1999–2049 (2020).
Jolivet, R., Grandin, R., Lasserre, C., Doin, M. P. & Peltzer, G. Systematic InSAR tropospheric phase delay corrections from global meteorological reanalysis data. Geophys. Res. Lett. 38 , L17311 (2011).
Petit, G., & Luzum, B. IERS Conventions (IERS Technical Note No. 36). Retrieved from Frankfurt, Germany: http://www.iers.org/TN36/ .
Yunjun, Z. et al. Range geolocation accuracy of C-/L-band SAR and its implications for operational stack coregistration. IEEE Trans. Geosci. Remote Sens. 60 , 5227219 (2022).
Fialko, Y., Simons, M. & Agnew, D. The complete (3-D) surface displacement field in the epicentral area of the 1999 MW7.1 Hector Mine Earthquake, California, from space geodetic observations. Geophys. Res. Lett. 28 , 3063–3066 (2001).
Olson, A. H., & Apsel, R. Finite fault and inversion theory with applications to the 1979 Imperial Valley earthquake, Bull. Seism. Soc. Am . 72 , 1969–2001 (1982).
Hartzell, S. H. & Heaton, T. H. Inversion of strong ground motion and teleseismic waveform data for the fault rupture history of the 1979 Imperial Valley, California earthquake. Bull. Seism. Soc Am. 73 , 1553–1583 (1983).
Hartzell, S. H., Liu, P. & Mendoza, C. The 1994 Northridge, California, earthquake: investigation of rupture velocity, rise time, and high-frequency radiation. J. Geophys. Res. Solid Earth . 101 , 20091–20108 (1996).
Yue, H. et al. Depth varying rupture properties during the 2015 Mw 7.8 Gorkha (Nepal) earthquake. Tectonophysics. 714 , 44–54 (2017).
Ammon, C. J. et al. Rupture process of the 2004 Sumatra-Andaman Earthquake. Science 308 , 1133–1139 (2005).
Minson, S. E. et al. Bayesian inversion for finite fault earthquake source models – II: the 2011 great Tohoku-oki, Japan earthquake. Geophys. J. Int. 198 , 922–940 (2014).
Kanamori, H. & Given, J. W. Use of long-period surface waves for rapid determination of earthquake-source parameters. Phys. Earth Planet. Inter. 27 , 8–31 (1981).
Wald, D. J. & Graves, R. W. Resolution analysis of finite fault source inversion using 1D and 3D Green’s functions. II Combining seismic and geodetic data. J. Geophys. Res. Solid Earth. 106 , 8767–8788 (2001).
Shao, G. et al. Focal mechanism and slip history of the 2011 Mw 9.1 off the Pacific coast of Tohoku Earthquake, constrained with teleseismic body and surface waves. Earth Planet. Sp. 63 , 559–564 (2011).
Zhu, L. & Rivera, L. A. A note on the dynamic and static displacements from a point source in multilayered media. Geophys. J. Int. 148 , 619–627 (2002).
Kiser, E. & Ishii, M. Back-projection imaging of earthquakes. Annu. Rev. Earth Planet. Sci. 45 , 1 (2017).
Ishii, M. et al. Extent, duration and speed of the 2004 Sumatra–Andaman earthquake imaged by the Hi-Net array. Nature. 435 , 933–936 (2005).
Kiser, E. & Ishii, M. The 2010 M w 8.8 Chile earthquake: triggering on multiple segments and frequency‐dependent rupture behavior. Geophys. Res. Lett. 38 , L07301 (2011).
Meng, L., Inbal, A. & Ampuero, J. P. A window into the complexity of the dynamic rupture of the 2011 Mw 9 Tohoku-Oki earthquake. Geophys. Res. Lett. 38 , L00G07 (2011).
Kennet, B. L. N. I. A. S. P. E. I. 1991 seismological tables. Terra Nova. 3 , 122 (1991).
Ishii, M., Shearer, P. M., Houston, H. & Vidale, J. E. Teleseismic P Wave imaging of the 26 December 2004 Sumatra-Andaman and 28 March 2005 Sumatra earthquake ruptures using the Hi-net array. J. Geophys. Res. 112 , B11307 (2007).
Meng, L. et al. Double pincer movement: encircling rupture splitting during the 2015 M w 8.3 Illapel earthquake. Earth Planet. Sci. Lett. 495 , 164–173 (2018).
Meng, L., Huang, H., Xie, Y., Bao, H. & Dominguez, L. A. Nucleation and kinematic rupture of the 2017 Mw 8.2 Tehuantepec earthquake. Geophys. Res. Lett. 46 , 3745–3754 (2019).
Hicks, S. P. et al. Back-propagating supershear rupture in the 2016 Mw 7.1 Romanche transform fault earthquake. Nat. Geosci. 13 , 647–653 (2020).
Zhang, X., Feng, W., Du, H., Samsonov, S. & Yi, L. Supershear rupture during the 2021 MW 7.4 Maduo, China, earthquake. Geophys. Res. Lett. 49 , e2022GL097984 (2022).
Mahmoud, Y. et al. Kinematic study at the junction of the East Anatolian fault and the Dead Sea fault from GPS measurements. J Geodyn. 67 , 30–39 (2013).
Styron, R. & Pagani, M. The GEM global active faults database. Earthq. Spectra. 36 , 160–180 (2020).
Download references
Acknowledgements
We thank Gilles Peltzer for valuable comments and discussions, Qingyue Yang, Junfeng Li and Yachao Wang for help with the LuTan-1 data processing. We thank the editor and anonymous reviewers for helping improve the quality of the manuscript. We thank the Disaster and Emergency Management Authority of Turkey (AFAD) for providing strong motion data and local earthquake catalog. We thank TUSAGA-Aktif for providing high-rate GNSS data. L.X., S.M., and L.M. were supported by NSF grant no. EAR-1848486 and Leon and Joanne V.C. Knopoff Fund. C.J. was supported by grants from the Southern California Earthquake Center (SCEC)-funded by NSF cooperative agreement EAR-0109624 and USGS cooperative agreement 02HQAG0008. J.-P.A. was supported by the French government through the UCAJEDI Investments in the Future project (ANR-15-IDEX-01) managed by the French National Research Agency (ANR). R.C. was supported by NSFC grants U20A2095 and 42325401. C.L. was supported by NSFC grant 42274026. Figures were produced using Generic Mapping Tools (GMT), MATLAB, and Python. The Python software package ObsPy was used for data requesting, waveform filtering, and CCs calculation.
Author information
Authors and affiliations.
Department of Earth, Planetary and Space Sciences, University of California Los Angeles, Los Angeles, CA, USA
Liuwei Xu, Saeed Mohanna, Lingsen Meng & Masooma Hasnain
Department of Earth Science, University of California Santa Barbara, Santa Barbara, CA, USA
Observatoire de la Côte d’Azur, Université Côte d’Azur, IRD, CNRS, Valbonne, France
Jean-Paul Ampuero
National Key Laboratory of Microwave Imaging Technology, Aerospace Information Research Institute, Chinese Academy of Sciences, Beijing, China
Zhang Yunjun
State Key Laboratory of Geodesy and Earth’s Dynamics, Innovation Academy for Precision Measurement Science and Technology, Chinese Academy of Sciences, Wuhan, China
Risheng Chu
Institute of Remote Sensing and Geographical Information System, School of Earth and Space Sciences, Peking University, Beijing, China
Cunren Liang
You can also search for this author in PubMed Google Scholar
Contributions
L.X.: performed the SEBP, the joint FFI, and the Rayleigh Mach wave analysis; designed the figures and tables; participated in the interpretation of the results; wrote the original draft. S.M.: performed the SEBP and the SEBP validation; designed the figures and tables; participated in the interpretation of the results; wrote the original draft. L.M.: conceived and led the study; performed the SEBP and the SEBP validation; designed the figures and tables; participated in the interpretation of the results; wrote the original draft. C.J.: conceived and led the study; performed the joint FFI; designed the figures and tables; participated in the interpretation of the results; wrote the original draft. J.-P.A.: performed the SEBP validation; participated in the interpretation of the results; wrote the original draft. Z.Y.: processed the SAR images; wrote the original draft. M.H.: performed the SEBP. R.C.: processed the China array seismic data. C.L.: processed the SAR images. All authors contributed to finalize the manuscript.
Corresponding authors
Correspondence to Lingsen Meng or Chen Ji .
Ethics declarations
Competing interests.
The authors declare no competing interests.
Peer review
Peer review information.
Communications Earth & Environment thanks the anonymous reviewers for their contribution to the peer review of this work. Primary Handling Editor: Joe Aslin. A peer review file is available
Additional information
Publisher’s note Springer Nature remains neutral with regard to jurisdictional claims in published maps and institutional affiliations.
Supplementary information
Supplementary information, supplementary movie 1, supplementary movie 2, supplementary movie 3, supplementary movie 4, supplementary movie 5, supplementary movie 6, supplementary movie 7, supplementary movie 8, supplementary movie 9, peer review file, description of additional supplementary files, rights and permissions.
Open Access This article is licensed under a Creative Commons Attribution 4.0 International License, which permits use, sharing, adaptation, distribution and reproduction in any medium or format, as long as you give appropriate credit to the original author(s) and the source, provide a link to the Creative Commons licence, and indicate if changes were made. The images or other third party material in this article are included in the article’s Creative Commons licence, unless indicated otherwise in a credit line to the material. If material is not included in the article’s Creative Commons licence and your intended use is not permitted by statutory regulation or exceeds the permitted use, you will need to obtain permission directly from the copyright holder. To view a copy of this licence, visit http://creativecommons.org/licenses/by/4.0/ .
Reprints and permissions
About this article
Cite this article.
Xu, L., Mohanna, S., Meng, L. et al. The overall-subshear and multi-segment rupture of the 2023 Mw7.8 Kahramanmaraş, Turkey earthquake in millennia supercycle. Commun Earth Environ 4 , 379 (2023). https://doi.org/10.1038/s43247-023-01030-x
Download citation
Received : 29 June 2023
Accepted : 29 September 2023
Published : 17 October 2023
DOI : https://doi.org/10.1038/s43247-023-01030-x
Share this article
Anyone you share the following link with will be able to read this content:
Sorry, a shareable link is not currently available for this article.
Provided by the Springer Nature SharedIt content-sharing initiative
By submitting a comment you agree to abide by our Terms and Community Guidelines . If you find something abusive or that does not comply with our terms or guidelines please flag it as inappropriate.
Quick links
- Explore articles by subject
- Guide to authors
- Editorial policies
Sign up for the Nature Briefing newsletter — what matters in science, free to your inbox daily.

What the New Jersey earthquake tells us about the fault system beneath the Northeast
Friday morning's earthquake , an event more commonly associated with California, was the first many Northeasterners had ever felt.
The temblor, which measured 4.8 on the Richter scale, was considered a shallow earthquake by the U.S. Geological Survey, meaning that it occurred at a depth of zero to 70 kilometers below ground. The USGS reported Friday morning that there was a 38% chance of an aftershock measuring magnitude 3.0 or more over the next week, and approximately eight hours later, one measuring 4.0 was recorded.
The energy released by an earthquake is weakened the greater the distance from where it occurs, so while the New Jersey earthquake occurred at a depth of 5 kilometers, the shaking it produced would have been even less if it had emanated from a deeper level.
But Scott Brandenberg, a professor of civil and environmental engineering at the UCLA Samueli School of Engineering, told Yahoo News that Friday’s earthquake is probably not indicative that longer-term earthquake activity is on the rise in the region.
What are the fault lines under New York and New Jersey?
Fault lines are fractures between blocks of rock in the Earth’s crust, the layer closest to the surface. These lines allow tectonic plates to move and earthquakes occur when two plates slide past each other.
The Ramapo Fault System is the longest in the northeastern U.S., stretching from Pennsylvania to southeastern New York.
“It’s pretty inactive,” Brandenberg told Yahoo News. “The reason why earthquakes are rare here is that the central and eastern U.S. is considered a stable continental region that is far away from the plate boundaries where tectonic plates move relative to each other.”
New Jersey has had several small earthquakes since the end of 2020, but they were all of a magnitude of less than 2.5, which barely registers, according to Michigan Tech University .
Since 1957, the USGS has logged 188 earthquakes with a magnitude of 2.5 or higher that have occurred within a 250-mile radius of New York City. The earthquake on Friday had the third-highest magnitude out of the data set.
Are fault lines monitored?
The USGS maintains several monitoring instruments around the country for known fault lines and volcanos. Data from these measurements is transmitted in real-time to researchers in California. While the fault lines are being monitored, scientists cannot predict when earthquakes will occur. Researchers can review scientific data and calculate probabilities for future earthquakes, but a short-term earthquake prediction method does not exist.
Is the Northeast’s infrastructure built to sustain earthquakes?
“There are earthquake building codes throughout the United States, so every structure is built to withstand some shaking level,” Brandenberg explained. “Other hazards, like storms, pose more risk than earthquakes.”
In terms of underground public transportation systems — with New York City having one of the oldest and largest transit systems in the world — Brandenberg again evinced little concern.
“Underground structures, like subways, tend to perform pretty well during earthquake-shaking,” he said. “There are potential problems if the fault ruptures all the way to the surface, but that's unlikely for the magnitude of earthquakes that tend to occur in the Northeast.”
- 0 Shopping Cart
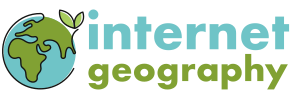
Christchurch Earthquake Case Study
The cause, effects and responses to the Christchurch Earthquake .
A case study of an earthquake in a HIC.
What caused the Christchurch earthquake?
The earthquake occurred on New Zealand’s South Island, 10km west of Christchurch, at 12.51 pm on 22nd February 2011 and lasted just 10 seconds. Measuring 6.3 on the Richter Scale and, at 4.99 km deep, the earthquake was very shallow. The earthquake occurred along a conservative margin between the Pacific Plate and the Australasian Plate. Take a look at the Christchurch earthquake animated map to see the swarm of foreshocks and aftershocks.
What were the effects of the Christchurch earthquake?
The primary effects included:
- Christchurch, New Zealand’s second city, experienced extensive damage
- 185 people were killed
- 3129 people were injured
- 6800 people received minor injuries
- 100,000 properties were damaged, and the earthquake demolished 10,000
- $28 billion of damage was caused
- water and sewage pipes were damaged
- the cathedral spire collapsed
- liquefaction destroyed many roads and buildings
- 2200 people had to live in temporary housing
The video below shows the effects of the earthquake one minute after it struck.
The secondary effects included:
- five Rugby World Cup matches were cancelled
- schools were closed for two weeks
- 1/5 of the population migrated from the city
- many businesses were closed for a long time
- two large aftershocks struck Christchurch less than four months after the city was devastated
- Economists have suggested that it will take 50 to 100 years for New Zealand’s economy to recover
- 80% of respondents to a post-event survey stated that their lives had changed significantly since the earthquake
What were the immediate responses to the Christchurch earthquake?
The immediate responses included:
- around $6-7 million of international aid was provided
- The Red Cross and other charities supplied aid workers
- rescue crews from all over the world, including the UK, USA, Taiwan and Australia, provided support
- more than 300 Australian police officers flew into Christchurch three days after the earthquake. They were sworn in with New Zealand policing powers and worked alongside New Zealand officers enforcing law and order and reassuring the people of Christchurch
- 30,000 residents were provided with chemical toilets
What were the long-term responses to the Christchurch earthquake?
The long-term responses included:
- the construction of around 10,000 affordable homes
- water and sewage were restored by August 2011
- the New Zealand government provided temporary housing
- Many NGOs provided support, including Save the Children
- Canterbury Earthquake Recovery Authority was created to organise rebuilding the region. It had special powers to change planning laws and regulations.
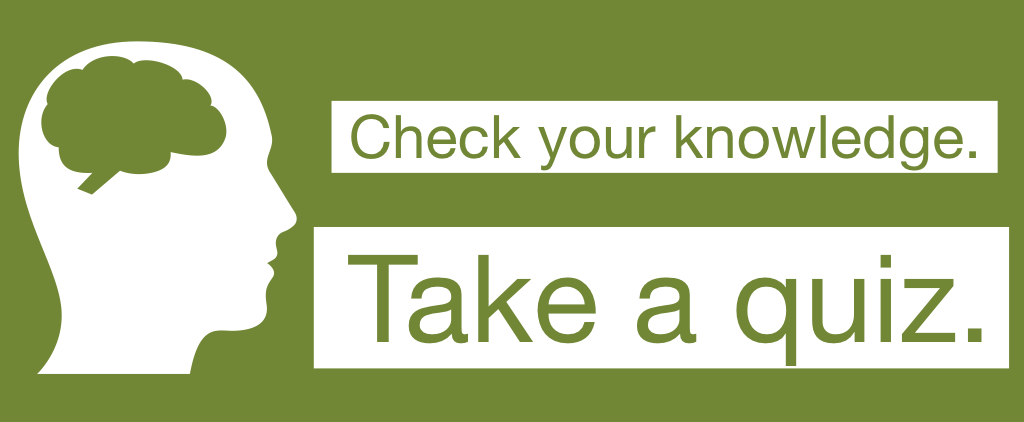
Premium Resources
Please support internet geography.
If you've found the resources on this page useful please consider making a secure donation via PayPal to support the development of the site. The site is self-funded and your support is really appreciated.
Related Topics
Use the images below to explore related GeoTopics.
Japan Earthquake 2011
Earthquake home, nepal earthquake 2015, share this:.
- Click to share on Twitter (Opens in new window)
- Click to share on Facebook (Opens in new window)
- Click to share on Pinterest (Opens in new window)
- Click to email a link to a friend (Opens in new window)
- Click to share on WhatsApp (Opens in new window)
- Click to print (Opens in new window)
If you've found the resources on this site useful please consider making a secure donation via PayPal to support the development of the site. The site is self-funded and your support is really appreciated.
Search Internet Geography
Top posts and pages.

Latest Blog Entries

Pin It on Pinterest
- Click to share
- Print Friendly
- Work & Careers
- Life & Arts
Become an FT subscriber
Try unlimited access Only $1 for 4 weeks
Then $75 per month. Complete digital access to quality FT journalism on any device. Cancel anytime during your trial.
- Global news & analysis
- Expert opinion
- Special features
- FirstFT newsletter
- Videos & Podcasts
- Android & iOS app
- FT Edit app
- 10 gift articles per month
Explore more offers.
Standard digital.
- FT Digital Edition
Premium Digital
Print + premium digital.
Today's FT newspaper for easy reading on any device. This does not include ft.com or FT App access.
- 10 additional gift articles per month
- Global news & analysis
- Exclusive FT analysis
- Videos & Podcasts
- FT App on Android & iOS
- Everything in Standard Digital
- Premium newsletters
- Weekday Print Edition
Essential digital access to quality FT journalism on any device. Pay a year upfront and save 20%.
- Everything in Print
- Everything in Premium Digital
Complete digital access to quality FT journalism with expert analysis from industry leaders. Pay a year upfront and save 20%.
Terms & Conditions apply
Explore our full range of subscriptions.
Why the ft.
See why over a million readers pay to read the Financial Times.
International Edition
New York City Rattled by 4.8 Magnitude Earthquake and Aftershock
A 4.8 magnitude earthquake struck near Whitehouse Station, New Jersey, at 10:23 a.m. ET on Friday morning. Tremors were felt throughout the state, New York City, Philadelphia, and as far south as Baltimore. The earthquake’s epicenter was in Lebanon, N.J., a city in the North-Central part of the state.
Per the United States Geological Survey (USGS), the earthquake is one of the strongest recorded in the Northeast in over a decade. There were no immediate reports of major injuries or damage.
There have been at least 18 aftershocks, ranging in magnitude, following the morning earthquake, according to the USGS. The most notable aftershock occurred in Gladstone, New Jersey, just before 6 p.m. on Friday and initially had a registered magnitude of 4.0, before being adjusted to 3.8 . This came after Alexandra Hatem of the (USGS) warned TIME there was around a 45% chance of an aftershock of magnitude greater than 3.0 occurring within the next week.
On Saturday morning, ABC7 New York reported two further aftershocks—of 2.5 and 1.9 magnitude—had taken place since 6 a.m. local time, noting that people had reported feeling the 2.5 magnitude aftershock.
Unsettled conditions continue today- not just because we’re still monitoring aftershocks (2 since 6am) but because the brisk breeze & spotty rain continues today too! Gusts ~30mph make our 52° high feel cooler under a mostly cloudy sky. Less wind & more sun Sunday! 🌥️☔️💨 @ABC7NY pic.twitter.com/LrioOkn2ZL — Dani Beckstrom (@danibeckstrom) April 6, 2024
Speaking during a media briefing on Friday afternoon, Sarah McBride of USGS remarked that the morning earthquake was so widely felt, it may have set a record. “This earthquake was widely felt and we know this because we have received more than 160,000 ‘Felt Reports’ on our website.” If you felt the earthquake, you can report it to the USGS here . This helps scientists gather more information about the shaking caused by earthquakes around the world.
At a press conference on Friday, Governor of New York Kathy Hochul said that Newark Liberty International Airport in New Jersey and John F. Kennedy International Airport had undergone full ground stops in the aftermath of the earthquake while the potential for aftershocks was being assessed. Flights resumed thereafter.
Hochul urged New Yorkers to take precautionary measures in case of an aftershock. “Drop to the floor, cover your neck, and hold on to something that is sturdy,” she advised. “If you hear any shifting or any unusual noises, leave your home and go outside. You're safer there than in a building that could be crumbling around you.”
Hochul also encouraged residents to check their homes for damage, including gas and water lines. An overloading of cell service was reported immediately in the aftermath of the main event, but networks then resumed normal service.
“These are emerging situations. It could be over, but also there could be another effect. We have to be prepared for that and warn New Yorkers to be particularly vigilant in the days following an earthquake,” said Hochul on Friday.
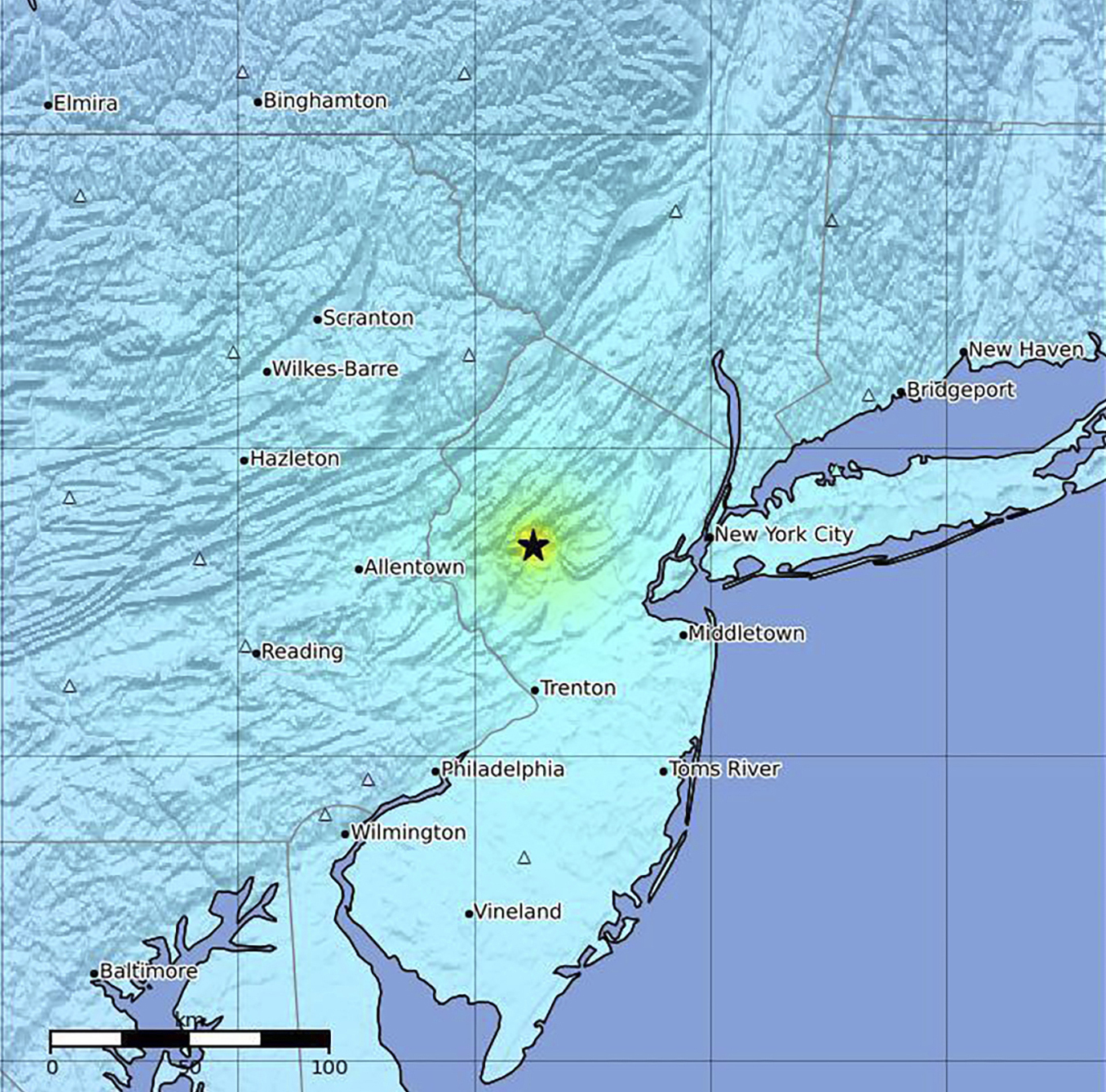
Are earthquakes becoming more frequent?
Seismologist Angie Lux of the Berkeley Seismological Laboratory tells TIME that the current level of seismic activity is consistent with what would be expected in the region. “Looking at the sort of historical seismicity in the area, I would definitely say that there's no clear trend of more earthquakes that are happening.”
A 5.8 magnitude earthquake occurred on the East Coast in August 2011. It struck Virginia, and was felt as far as Boston.
While earthquakes are not as common in the Northeast as they are on the West Coast, earthquakes east of the Rocky Mountains can often cause shaking at much further distances than earthquakes of a similar magnitude on the West Coast. This is thought to be because rocks on the West Coast are younger and more broken up than rocks found along the East Coast, which means that they are able to absorb more of an earthquake’s seismic energy, according to an article published by the USGS.
Lux says that large earthquakes are capable of happening anywhere across the mainland U.S. “The potential for really significant earthquakes is lower [on the East Coast] than we see in other places, but there's always a possibility of earthquakes if we look at the seismic hazard maps,” says Lux.
Human activity can also impact the frequency of earthquakes. Practices like fracking and drilling have been shown to increase the seismic activity in places like Oklahoma and Texas.
Nevertheless, the Northeast remains vulnerable since unlike California and other Western states, building codes in the East Coast are not designed with major earthquakes in mind and earthquake early warning alert systems are not in place.
Reactions to the earthquake from New York and New Jersey
TIME employees across New York City and New Jersey shared their experiences of the earthquake on Friday morning. “I live on the first floor of an old building in Flatbush, Brooklyn, and I truly thought that something was about to explode in the basement,” says Erin, who was working from home when the earthquake struck.
Meanwhile Chardia, who lives on the 23rd floor of a building in the Bronx, says she was on a virtual call with her team when the earthquake hit. “We all live in different parts of New York, and you could see the confusion across each face as the screens began to shake and the ‘Did y’all feel that’ messages came rushing through,” she says. “It's a surreal feeling of helplessness.”
In downtown Manhattan, Melody noticed the earthquake when she saw colleagues shaking on her computer screen during a weekly video call. “I thought my dog was stuck under the couch trying to rattle out or something because my couch and the floor moved,” she says. “I realized it was an earthquake and got worried she would be smooshed under the couch, but then I see[n] her calm as ever laying in her doggy bed, staring at me.”
California-raised Melody says that she remembered earthquake drills from her upbringing, telling her to stay inside, away from windows, and seek cover under hard objects. “Drop, cover and hold onto something,” she adds.
Tameka—who lives in South Jersey—says her dog reacted before her, by running into her workspace area “seeming frantic and worried.” She says that “by the time I could respond to her, the shaking started. It was over before we made it to the nearest doorway in my apartment.”
Recent news coverage of the earthquake in Taiwan left Tameka feeling “mildly prepared” for Friday’s events. “Without such timely news of another earthquake, one hitting here would've been the farthest from my mind," she notes.
More Must-Reads From TIME
- Exclusive: Google Workers Revolt Over $1.2 Billion Contract With Israel
- Stop Looking for Your Forever Home
- Jane Fonda Champions Climate Action for Every Generation
- Hormonal Birth Control Doesn’t Deserve Its Bad Reputation
- The Sympathizer Counters 50 Years of Hollywood Vietnam War Narratives
- Essay: The Relentless Cost of Chronic Diseases
- The Best TV Shows to Watch on Peacock
- Want Weekly Recs on What to Watch, Read, and More? Sign Up for Worth Your Time
Write to Armani Syed at [email protected] and Olivia-Anne Cleary at [email protected]
You May Also Like
Advertisement
Was Today’s Earthquake Connected to the Solar Eclipse?
The tidal forces on Earth grow as the sun, moon and Earth begin to align, a configuration that can lead to a solar eclipse. But the results of several studies of the relationship between earthquakes and tides are inconclusive, a geophysicist said.
- Share full article

By Katrina Miller
- April 5, 2024
With a total solar eclipse set to pass through the United States on Monday, it is easy to imagine a linkage between unusual events in the heavens and on Earth. But geoscientists were cautious about making such a connection.
Earthquakes happen along fault lines, or cracks between two blocks of rock on Earth’s crust. Tides stretch and squish the land on Earth just as they contribute to waves in the ocean, and those tidal forces grow as the sun, moon and Earth begin to align — a configuration that sometimes creates a solar eclipse.
One theory is that this may introduce additional stress along Earth’s fault lines.
“We do know that the relative position of the Earth and the moon and the sun does exert tidal forces,” said William Frank, a geophysicist at the Massachusetts Institute of Technology. “And we know that changes the stress that can be on a fault that can host an earthquake.”
But the results of several studies of the relationship between earthquakes and tides are inconclusive, according to Seth Stein, a geophysicist at Northwestern University. “If there’s any effect, it would be incredibly weak,” he said.
Earthquakes are driven most often by the motion between two tectonic plates making up Earth’s crust — either when two plates slide along each other in opposite directions, or when one slides under the other.
Both types of movements introduce strain at the junction, which often gets relieved by an earthquake.
But at the moment, it’s difficult to say that plate motion was responsible for the quake that shook the Northeast Friday morning.
“It’s not quite as obvious, because there is no tectonic plate boundary that is active,” Dr. Frank said.
Still, he added, fault lines from past activity are everywhere on Earth’s crust.
“Some of these faults can still be storing stress and be closure to failure,” he said. “And it can just require a little bit more to push it over the edge.”
Katrina Miller is a science reporting fellow for The Times. She recently earned her Ph.D. in particle physics from the University of Chicago. More about Katrina Miller
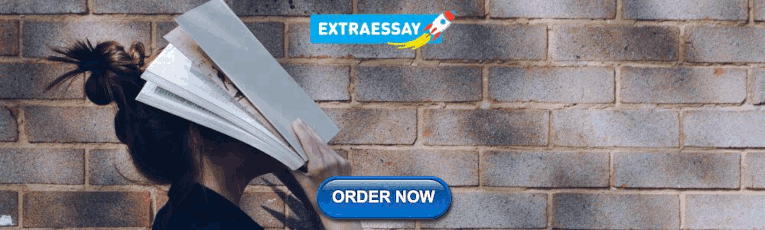
COMMENTS
Case study: Haiti Earthquake, 2021; Prediction, protection and preparation; Causes of tsunamis; Case study: Haiti Earthquake, 2021. On 14th August 2021 a magnitude 7.2 earthquake struck Haiti in ...
In the first in the series, on 29 July, a 6.4 magnitude quake triggered landslides in the mountain region of the island and killed at least 16 people. Following this a shallow, magnitude 6.9 earthquake struck Lombok and Bali on August 5th, 2018, killing over 555 people, injuring 1300 and leaving at least 353000 homeless.
Sources: U.S. Geological Survey (epicenter); NASA/JPL-Caltech (Copernicus Sentinel-1 data analysis) · Note: This change in the earth's surface illustrates the scale of the earthquake, but it ...
Overview. On Friday 28th September 2018 a magnitude 7.5 earthquake struck Palu, on the Indonesian island of Sulawesi, just before dusk wreaking havoc and destruction across the city and triggering a deadly tsunami on its coast. The 7.5 magnitude earthquake hit only six miles from the country's coast. A map to show the location of Palu.
The strongest so far is a M w 4.7 [1] Casualties. 2 dead, 12 injured. The 2021 Assam earthquake struck 11 km (7 miles) away from Dhekiajuli, Assam, India at 07:51 (IST) on April 28, 2021 with a moment magnitude of 6.0 at 34.0 km (21.1 mi) depth. [2] [3] The quake struck with an epicenter 140 km (86 miles) north of the main city of Guwahati.
A magnitude-7.8 earthquake hit southeastern Turkey and parts of Syria in the early hours of the morning of 6 February. At least 17,000 people are known to have lost their lives, with thousands ...
Wednesday's earthquake shook more parts of Taiwan with greater intensity than any other quake since 1999, when a 7.7 magnitude tremor hit the middle of the island, killing 2,400 people and ...
Geologists knew decades ago that a quake would strike southeastern Turkey, but precise prediction is still the stuff of science fiction. The magnitude-7.8 earthquake in Turkey last month destroyed ...
In 1999, a 7.6-magnitude earthquake in Taiwan killed nearly 2,500 people. That quake, which struck about 90 miles south-southwest of Taipei, was the second-deadliest in the island's history ...
Recent Earthquake Activity. Earth is a dynamic planet. Its crust is continuously forming and deforming. The crust is constantly pushed and pulled as it moves, causing a strain to build up over time. When this built-up strain is suddenly released, the crust shakes, and we call this movement an earthquake. Depending upon their strength and where ...
Scientific Reports - Using mobile phone data to map evacuation and displacement: a case study of the central Italy earthquake. ... In a recent study on mobile phone data published by 31, the ...
An aftershock of 4.0-magnitude hit later in the day. An earthquake struck the East Coast of the United States on Friday morning, according to the U.S. Geological Survey, causing buildings to shake ...
The earthquake. The 7.8-magnitude temblor, striking in the early hours of Feb. 6, was Turkey's deadliest earthquake since 1939, when more than 30,000 people were killed, and among the deadliest ...
On August 7th, 2020, a magnitude Mw = 5.0 earthquake shook 5 km north of Mila city center, northeast of Algeria, causing substantial damage directly to structures, and indirectly from induced impacts of landslides and rock falls, ultimately disrupt to everyday civilian life. Given the recent significant seismic occurrences in the region, a detailed and comprehensive examination and assessment ...
The earthquake that struck Santa Rosa on April 2nd, 2024, had a magnitude of 3.2 and occurred at a very shallow depth of 6 miles beneath the epicenter. While the quake was not strong enough to ...
Moderate-size earthquakes of about magnitude 6 have occurred on the Parkfield section of the San Andreas fault at fairly regular intervals - in 1857, 1881, 1901, 1922, 1934, and 1966. The first, in 1857, was a foreshock to the great Fort Tejon earthquake which ruptured the fault from Parkfield to the southeast for over 180 miles.
Photo: John Minchillo/AP. A 4.8 magnitude earthquake shook New Jersey and New York City on Friday morning, followed by a 4.0 magnitude aftershock in the evening — surprising and confusing area ...
2.1. Study area. The 512 Wenchuan Earthquake in 2008 was the most destructive and wide-ranging earthquake in the last 70 years in China, killing more than 69,000 people, injuring more than 370,000 people, and causing more than 123 billion dollars loss for the economy.
This article provides a case study of the massive and tragic earthquake that occurred in Türkiye, and the bordering country of Syria, in February 2023. Earthquakes are not uncommon in this part of the world. However, the one that happened on 6 February 2023 had a magnitude of 7.8 and was the deadliest and strongest recorded in Türkiye since ...
What to Know. Earthquakes are the result of rapid motion along a fault line. In Friday's case, it occurred along the Ramapo Fault that runs down the center of the northern part of the state.
This paper is motivated by the unique findings and observations from reconnaissance visits after the earthquake series in Puerto Rico in January 2020. It aims to discuss the potential interactions of Hurricane Maria and 2020 earthquake series and the considerations they underscore for future field reconnaissance missions. Traditionally, post-disaster damage assessment activities focus on one ...
The Mila, Algeria's recent earthquake damaged the structure. The building was not built according to seismic design principles. ... Seismic damage assessment of unreinforced masonry structures after the abruzzo 2009 earthquake: the case study of the historical centers of L'Aquila and castelvecchio subequo. Int J Architect Herit. 2013; 7 (5 ...
Prior to the 2023 earthquakes, the 2020 Elazig earthquake was the most recent M > 6.5 event to rupture the EAF. ... a case study of the 2015 Gorkha earthquake. Geophys. Res.
New Jersey has had several small earthquakes since the end of 2020, but they were all of a magnitude of less than 2.5, which barely registers, according to Michigan Tech University. . Since 1957 ...
The earthquake occurred on New Zealand's South Island, 10km west of Christchurch, at 12.51 pm on 22nd February 2011 and lasted just 10 seconds. Measuring 6.3 on the Richter Scale and, at 4.99 km deep, the earthquake was very shallow. The earthquake occurred along a conservative margin between the Pacific Plate and the Australasian Plate.
Levi Strauss lifted its full-year earnings guidance after a forecast-beating quarter, helping send shares up more than 10 per cent in after-hours trading. The company raised its forecast for ...
India is a seismically active region and experiences frequent earthquakes. Therefore, earthquake safety and prevention is of utmost importance in India. Recent studies have focused on improving ...
A 4.8 magnitude earthquake struck near Whitehouse Station, New Jersey, at 10:23 a.m. ET on Friday morning. Tremors were felt throughout the state, New York City, Philadelphia, and as far south as ...
Article ID IJIR-2292, Pages 568- 572. www.ijirem.org. Innovative Research Publication 568. Case Study o f Earthquake Resistant Structure and Its Recent. Innovation In Construction. Abhilash Thakur ...
Aubrey Gemignani/NASA. With a total solar eclipse set to pass through the United States on Monday, it is easy to imagine a linkage between unusual events in the heavens and on Earth. But ...