Effects of Climate Change on Ecology
Our climate is warming , which is changing the physical environments that support living systems. In the ocean, water temperatures are rising and becoming more acidic. On land, temperatures are rising as well, and soil health and freshwater quality are declining. In many places, environments are changing so fast that plants and animals cannot keep up, endangering entire ecosystems.
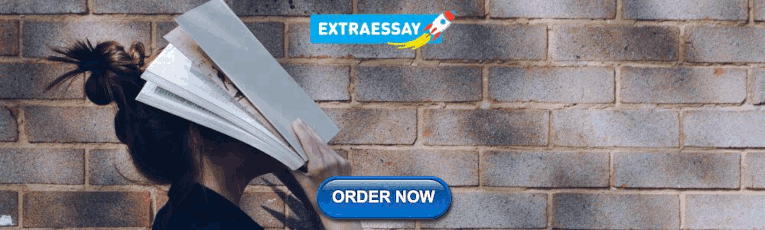
Climate change causes shifts in ecosystems.
Rising temperatures cause shifting ecosystems , either expanding or decreasing the geographical range of specific types of habitats, or changing the timing of seasons. For example, a study of European butterflies found that populations had shifted north by 114 km between 1990-2008 due to increasing temperatures and expansion of suitable habitat.
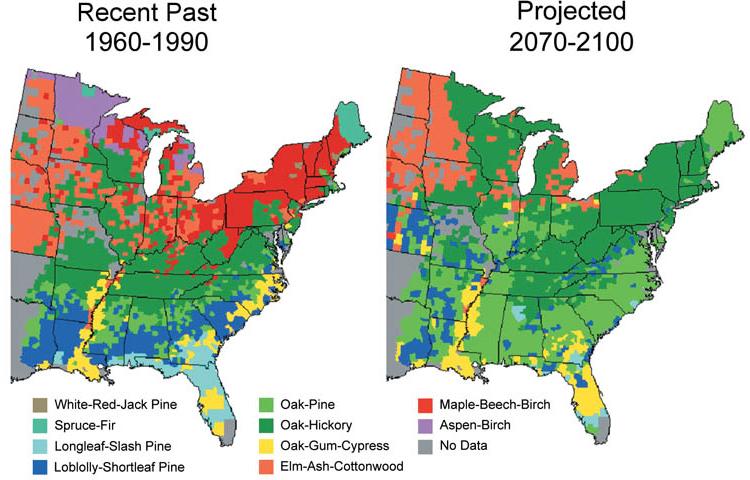
Forest types are projected to shift as the climate continues to warm. Notice that the Maple-Beech-Birch forests of the Northeast (red) are replaced with Oak-Hickory forests (green) when run under a lower emissions climate model scenario. Credit: EPA
Sometimes this expanding range brings new invasive species, which can cause native species to decline or go extinct, which alters the ecosystem. Slight changes in temperature are enough to cause spring thaw to happen earlier and fall frost to come sooner, which changes the timing of the growing season for plants and trees. This changes the availability of food, which can affect the size and health of populations within an ecosystem.
Rising temperatures threaten species diversity.
As temperatures continue to rise, many species are no longer able to thrive in places where they once lived. Scientists estimate that 8% of current animal species are at risk of extinction due to climate change alone.
Near the equator, a region with Earth’s highest biodiversity, many species are not able to adapt to rising temperatures. Reef fish are already living in the warmest water that they can tolerate and cannot survive as the water warms even more. It is also estimated that by 2070 nearly 20% of tropical plant species will be unable to germinate because of temperatures beyond their upper limit.
The frequency of hot, dry conditions increases as the climate warms, which intensifies wildfires. In the western United States, projections show that a 1°C rise in global temperature increases the average area burned by wildfires each season by 600%, impacting local populations of plants and animals in the path of the fires. The bushfire that burned over 25 million acres in Australia during 2019-2020, which started due to a lightning strike following an especially hot, dry spell, killed an estimated one billion animals. Many of the animals that died in these fires are found only in Australia, which raises concern about the future of these unique ecosystems. While local plant and animal populations usually recover following a fire, species that only exist in very small numbers or are found only in limited places are at higher risk for global extinction.
Extreme weather events impact ecosystems.
Scientists see a correlation between climate change and the increase of severe weather events, which can significantly impact species and ecosystems in a short amount of time. For example, in 2019, an extreme heatwave hit Cairns, Australia, causing the death of one-third of the spectacled flying foxes in just two days as temperatures climbed to 42°C (about 108°F). Even though animals such as flying foxes are adapted to the typical Australian heat, we see that they are unable to survive the extreme temperatures that are becoming more common due to climate change.
The intensity of tropical storms and hurricanes is also increasing due to warmer sea surface temperatures, causing more disruption to coastal plants and animals as habitat is changed or destroyed when the storms make landfall. The severe flooding and winds from these storms can impact ecosystems by disrupting nutrient cycling and the growth of plants. As the climate warms, more areas are affected by tropical storms and hurricanes, which means that more ecosystems are vulnerable to damage from extreme weather than in the recent past.
Sea level rise is causing the loss of coastal ecosystems.
Rising seas are displacing hundreds of thousands of people along the coasts and causing the loss of wetland ecosystems. By 2080 as much as 22% of the planet’s wetlands could be lost due to sea level rise. Coastal Louisiana, US, which has over two million acres of wetlands, is losing about a football field worth of land every 45 minutes, which is faster than almost any other place on Earth. Wetlands ecosystems protect coastlines from floods and store three times as much carbon as forests, which is important for mitigating climate change . The loss of coastal wetlands due to climate change threatens the diverse species of plants and animals that live within them, and impacts fishing economies that depend on marine life.
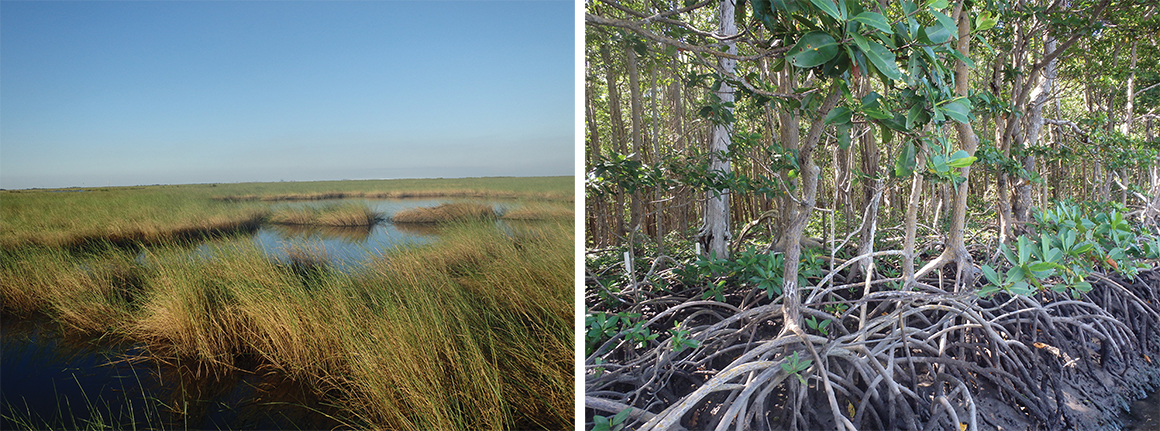
Ecosystems change along the Gulf of Mexico wetlands, as marsh grasses (left) are replaced by mangrove forests (right) due to warmer winters. Credit: LA-SAFE
Coral reefs are dying due to warming ocean temperatures.
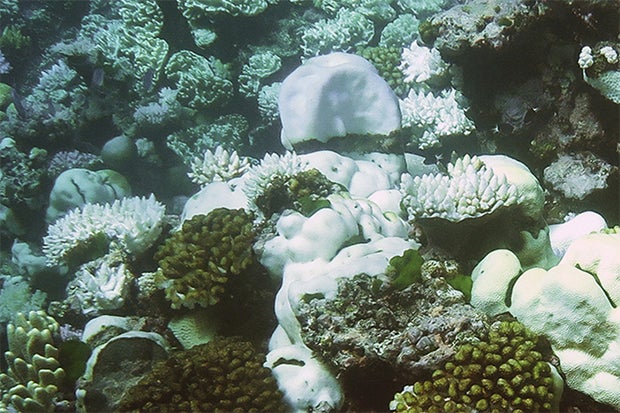
Healthy coral in Australia’s Great Barrier Reef are brown and green, while bleached coral that have been damaged due to warming ocean temperatures are white. Credit: NOAA
Studies estimate that one third to one half of the Earth’s corals have been lost, due in part to the warming ocean. When average ocean temperatures rise just 1°C, the coral become stressed and expel the symbiotic algae that make their food, resulting in the appearance of white coral (called coral bleaching). Though coral reefs cover less than one percent of the ocean floor, they support about 25% of all life in the ocean. The loss of coral reefs threatens marine ecosystems that depend on reefs as nurseries for fish and other marine species. Coastlines normally protected by coral reefs are also more vulnerable to erosion and storms.
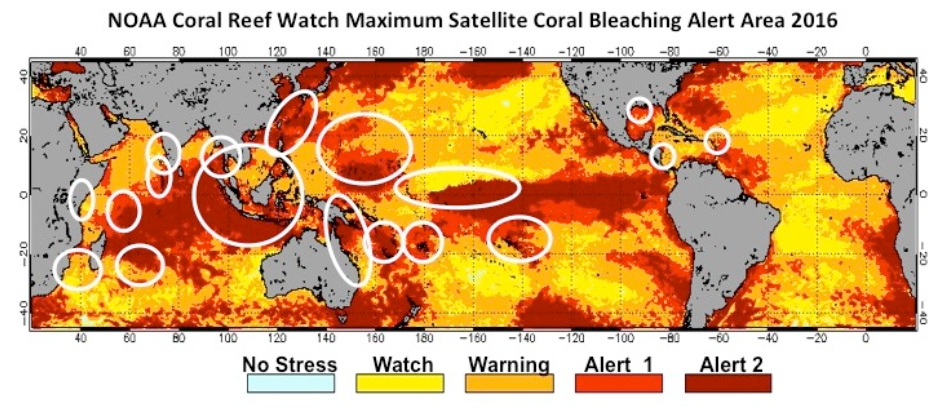
In this Coral Reef Watch Alert Area map for January-December 2016, severe bleaching was reported in areas circled in white. Note that there aren't any coral reefs in the "No Stress" category, a sign that warming temperatures due to climate change are endangering coral reefs on a global scale.
Melting ice is causing habitat loss in the polar regions.
Antarctica is losing three times as much ice today as it was 25 years ago, and trillions of tons of ice are melting from the Greenland Ice Sheet each year due to climate change. The warming of the polar regions creates fragmented habitats for species that depend on the ice for survival, such as caribou and polar bears in the Arctic . In the Antarctic, melting is changing the distribution of penguin colonies and allows new plant species to establish in areas once covered in snow and ice year-round.
- Impacts of Climate Change
- Potential Consequences of Climate Change
- Rising Sea Level
- Warming of the Polar Regions
- Studying Lions From Space as Climate Changes
- The Changing Carbon Cycle
- The Changing Nitrogen Cycle
- The Water Cycle and Climate Change
- Corals and Climate

Ecological Impacts of Climate Change (2008)
Chapter: 1 introduction.
Below is the uncorrected machine-read text of this chapter, intended to provide our own search engines and external engines with highly rich, chapter-representative searchable text of each book. Because it is UNCORRECTED material, please consider the following text as a useful but insufficient proxy for the authoritative book pages.
1 Introduction The worldâs climate is changing, and it will continue to change throughout the 21st century and beyond. Rising temperatures, new precipitation patterns, and other changes are already affecting many aspects of human society and the natural world. Climate change is transforming ecosystems at extraordinary rates and scales. As each species responds to its changing environment, its interactions with the physical world and the creatures around it changeâtriggering a cascade of impacts throughout the ecosystem, such as expansion into new areas, the intermingling of formerly non-overlapping species, and even species extinctions Climate change is happening on a global scale, but the ecological impacts are often local and vary from place to place. To illuminate how climate change has affected specific species and ecosystems, this document presents a series of examples of ecological impacts of climate change that have already been observed across the United States. Human actions have been a primary cause of the climate changes observed today, but humans are capable of changing our behavior in ways that reduce the rate of future climate change. Human actions are also needed to help wild species adapt to climate changes that cannot be avoided. Our approaches to energy, agriculture, water management, fishing, biological conservation, and many other activities will all affect the ways and extent to which climate change will alter the natural worldâand the ecosystems on which we depend. What are ecosystems and why are they important? Humans share Earth with a vast diversity of animals, plants, and microorganisms. Virtually every part of the planetââthe continents, the oceans, and the atmosphereââteems with life. Even the deepest parts of the ocean and rock formations hundreds of meters below the surface are populated with organisms adapted to cope with the unique challenges each environment presents. In our era organisms almost everywhere are facing a new set of challenges; specifically, the challenges presented by rapid climate change. How have plants, animals, and microorganisms coped with the climate changes that have already occurred, and how might they cope with future changes? To explore these questions we start with a discussion of how plants, animals, and microorganisms fit together in ecosystems and the role of climate in those relationships. Earth has a great diversity of habitats. These differ in climate, of course, but also in soils, day length, elevation, water sources, chemistry, and many other factors, and consequently, in the kinds of organisms that inhabit them. The animals, plants, and microorganisms that live in one place, along with the water, soils, and landforms, make an ecosystem. When we attempt to understand the impacts of climate change, thinking about ecosystemsââand not just individual speciesââcan be helpful because each ecosystem depends on a wide array of interactions among individuals. Some of these involve competition. For example, some plants shade others or several animals compete for the same scarce food. Some involve relationships between animals and their prey. Others involve decomposition, the process of decay that returns minerals and organic matter to the soil. And some interactions are beneficial to both partners, for example, bees that obtain food from flowers while pollinating them. Climate influences ecosystems and the species that inhabit them in many ways. In general, each type of ecosystem is consistently associated with a particular combination of climate characteristics (Walter 1968). Warm tropical lands with year-round rain typically support
2 Introduction tall forests with evergreen broadleaved trees. Midlatitude lands with cold winters and moist summers usually support deciduous forests, while drier areas are covered in grasslands, shrublands, or conifer forests. In a similar fashion shallow tropical-ocean waters harbor coral reefs on rocky bottoms and mangrove forests along muddy shores, whereas temperate shores are characterized by kelp forests on rocky bottoms and seagrasses or salt marshes on sediment- covered bottoms. These major vegetation types or biomes can cover vast areas. Within these areas a wide range of subtly different ecosystems utilize sites with different soils, topography, land-use history, ocean currents, or climate details. Humans are an important part of most ecosystems, and many ecosystems have been heavily modified by humans. A plot of intensively managed farmland, a fish pond, and a grazed grassland are just as much ecosystems as is a pristine tropical forest. All are influenced by climate, all depend on a wide variety of interactions, and all provide essential benefits to people. The lives of animals, plants, and microorganisms are strongly attuned to changes in climate, such as variation in temperatures; the amount, timing, or form of precipitation; or changes in ocean currents. Some are more sensitive and vulnerable to climate fluctuations than others. If the climate change is modest and slow, the majority of species will most likely adapt successfully. If the climate change is large or rapid, more and more species will face ecological changes to which they may not be able to adapt. But as we will see later, even modest impacts of climate change can cause a range of significant responses, even if the changes are not so harsh that the organism dies. Organisms may react to a shift in temperature or precipitation by altering the timing of an event like migration or leaf emergence, which in turn has effects that ripple out to other parts of the ecosystem. For example, such timing changes may alter the interactions between predator and prey, or plants (including many crops) and the insects that pollinate their flowers. Ultimately we want to understand how climate change alters the overall functioning of the ecosystem and in particular how it alters the ability of the ecosystem to provide valuable services for humans. Ecosystems play a central role in sustaining humans (Figure 1) (Daily 1997; Millennium Ecosystem Assessment 2005). Ecosystems provide products directly consumed by people. This includes food and fiber from agricultural, marine, and forest ecosystems, plus fuel, including wood, grass, and even waste from some agricultural crops, and medicines (from plants, animals and seaweeds). Our supply and quality of fresh water also depends on ecosystems, as they play a critical role in circulating, cleaning, and replenishing water supplies. Ecosystems also regulate our environment; for example, forests, floodplains, and streamside vegetation can be critically important in controlling risks from floods; likewise, mangroves, kelp forests, and coral reefs dampen the impact of storms on coastal communities. Ecosystems provide cultural services that improve our quality of life in ways that range from the sense of awe many feel when looking up at a towering sequoia tree to educational and recreational opportunities. Ecosystems also provide natureâs support structure; without ecosystems there would be no soil to support plants, nor all the microorganisms and animals that depend on plants. In the oceans, ecosystems sustain the nutrient cycling that supports marine plankton, which in turn supply food for the fish and other seafood humans eat. Algae in ocean ecosystems produce much of the oxygen that we breathe. In general, we do not pay for the services we get from ecosystems, even though we could not live without them and would have to pay a high price to provide artificially.
Introduction 3 FIGURE 1 Ecosystem services. SOURCE: Millennium Ecosystem Assessment (2005). Ecosystem services rely on complex interactions among many species, so in most environments it is critical that they contain a diverse array of organisms. Even those services that appear to depend on a single species, like the production of honey, actually depend on the interactions of many species, sometimes many hundreds or thousands. Honey comes from honeybees, but the bees depend on pollen and nectar from the plants they pollinate. These plants depend not only on the bees but also on the worms and other soil animals that aerate the soil, the microorganisms that release nutrients, and the predatory insects that limit populations of plant- eating insects. Scientists are still at the early stages of understanding exactly how diversity contributes to ecosystem resilienceâthe ability of an ecosystem to withstand stresses like pollution or a hurricane without it resulting in a major shift in the ecosystemâs type or the services it provides (Schulze and Mooney 1993; Chapin et al. 1997; Tilman et al. 2006; Worm et al. 2006). But we are already certain about one thing. Each species is a unique solution to a challenge posed by nature and each speciesâ DNA is a unique and complex blueprint. Once a species goes extinct, we canât get it back. Therefore, as we look at the impacts of climate change on ecosystems, it is critical to remember that some kinds of impactsâlosses of biological diversityâare irreversible. What do we know about current climate change? Over the last 20 years the worldâs governments have requested a series of authoritative assessments of scientific knowledge about climate change, its impacts, and possible approaches
4 Introduction for dealing with climate change. These assessments are conducted by a unique organization, the Intergovernmental Panel on Climate Change (IPCC). Every five to seven years, the IPCC uses volunteer input from thousands of scientists to synthesize available knowledge. The IPCC conclusions undergo intense additional review and evaluation by both the scientific community and the worldâs governments, resulting in final reports that all countries officially accept (Bolin 2007). The information in the IPCC reports has thus been through multiple reviews and is the most authoritative synthesis of the state of the science on climate change. Earthâs average temperature is increasing In 2007 the IPCC reported that Earthâs average temperature is unequivocally warming (IPCC 2007b). Multiple lines of scientific evidence show that Earthâs global average surface temperature has risen some 0.75°C (1.3°F) since 1850 (the starting point for a useful global network of thermometers). Not every part of the planetâs surface is warming at the same rate. Some parts are warming more rapidly, particularly over land, and a few parts (in Antarctica, for example) have cooled slightly (Figure 2). But vastly more areas are warming than cooling. In the United States average temperatures have risen overall, with the change in temperature generally much higher in the northwest, especially in Alaska, than in the south (Figure 3). The eight warmest years in the last 100 years, according to NASA's Goddard Institute for Space Studies, have all occurred since 1998 (http://www.giss.nasa.gov/research/news/20080116/). During the second half of the 20th century, oceans have also become warmer. Warmer ocean waters cause sea ice to melt, trigger bleaching of corals, result in many species shifting their geographic ranges, stress many other species that cannot move elsewhere, contribute to sea- level rise (see below), and hold less oxygen and carbon dioxide.
Introduction 5 FIGURE 2 Global trends in temperature. The upper map shows the average change in temperature per decade from 1870 to 2005. Areas in orange have seen temperatures rise between 0.1-0.2oC per decade, so that they average 1.35 to 2.7oC warmer in 2005 than in 1870. The lower map shows the average change in temperature per decade from 1950 to 2005. Areas in deep red have seen temperatures rise on average more than 0.4oC per decade, so that they average more than 2oC warmer in 2005 than in 1950. SOURCE: Joint Institute for the Study of the Atmosphere and Ocean, University of Washington.
6 Introduction FIGURE 3 Temperature trends in North America, 1955 to 2005. The darker areas have experienced greater changes in temperature. For example, the Pacific Northwest had average temperatures about 1oC higher in 2005 than in 1955, while Alaskaâs average temperature had risen by over 2oC. SOURCE: Created with data from Goddard Institute for Space Studies. Sea levels are rising Climate change also means that sea levels are rising. Not only do warmer temperatures cause glaciers and land ice to melt (adding more volume to oceans), but seawater also expands in volume as it warms. The global average sea level rose by just under 2 mm/yr (0.08in/yr) during the 20th century, but since satellite measurements began in 1992, the rate has been 3.1 mm/year (0.12in/yr)(IPCC 2007a). Along some parts of the U.S. coast, tide gauge records show that sea level rose even faster (up to 10 mm/yr, 0.39in/yr) because the land is also subsiding. As sea level rises, shoreline retreat has been taking place along most of the nationâs sandy or muddy shorelines, and substantial coastal wetlands have been lost due to the combined effects of sea- level rise and direct human activities. In Louisiana alone, 4900 km2 (1900 mi2) of wetlands have been lost since 1900 as a result of high rates of relative sea-level rise together with curtailment of the supply of riverborne sediments needed to build wetland soils. The loss of these wetlands has diminished the ability of that region to provide many ecosystem services, including commercial fisheries, recreational hunting and fishing, and habitats for rare, threatened, and migratory species, as well as weakening the regionâs capacity to absorb storm surges like those caused by Hurricane Katrina (Day et al. 2007). Higher sea levels can also change the salinity and water circulation patterns of coastal estuaries and bays, with varying consequences for the mix of species that can thrive there.
Introduction 7 Other effects are being seen Water Cycle Climate change is linked to a number of other changes that already can be seen around the world. These include earlier spring snowmelt and peak stream flow, melting mountain glaciers, a dramatic decrease in sea ice during the arctic summer, and increasing frequency of extreme weather events, including the most intense hurricanes (IPCC 2007b). Changes in average annual precipitation have varied from place to place in the United States (Figure 4). Climate dynamics and the cycling of water between land, rivers and lakes, and clouds and oceans are closely connected. Climate change to date has produced complicated effects on water balances, supply, demand, and quality. When winter precipitation falls as rain instead of snow and as mountain snowpacks melt earlier, less water is âstoredâ in the form of snow for slow release throughout the summer (Mote 2003), when it is needed by the wildlife in and around streams and rivers and for agriculture and domestic uses. Even if the amount of precipitation does not change, warmer temperatures mean that moisture evaporates more quickly, so that the amount of moisture available to plants declines. The complex interaction between temperature and water demand and availability means that climate change can have many different kinds of effects on ecosystems. FIGURE 4 Trends in precipitation from 1901 to 2006 in the United States. Areas in red are averaging some 30 percent less precipitation per year now than they received early in the 1900s. Dark blue areas are averaging 50 percent more precipitation per year. SOURCE: Backlund 2008. Created with data from the USGS and NOAA/NCDC. Extreme Events The character of extreme weather and climate events is also changing on a global scale. The number of frost days in midlatitude regions is decreasing, while the number of days with extreme warm temperatures is increasing. Many land regions have experienced an increase in days with very heavy rain, but the recent CCSP report on climate extremes concluded that âthere are recent
8 Introduction regional tendencies toward more severe droughts in the southwestern U.S., parts of Canada and Alaska, and Mexicoâ (Kunkel et al. 2008, Dai et al. 2004; Seager et al., 2007). These seemingly contradictory changes are consistent with a climate in which a greater input of heat energy is leading to a more active water cycle. In addition, warmer ocean temperatures are associated with the recent increase in the fraction of hurricanes that grow to the most destructive categories 4 and 5 (Emanuel 2005; Webster et al. 2005). Arctic Sea Ice Every year the area covered by sea ice in the Arctic Ocean expands in the winter and contracts in the summer. In the first half of the 20th century the annual minimum sea-ice area in the Arctic was usually in the range of 10 to 11 million km2 (3.86 to 4.25 million mi2) (ACIA 2005). In September 2007 sea-ice area hit a single-day minimum of 4.1 million km2 (1.64 million mi2), a loss of about half since the 1950s (Serreze et al. 2007). The decrease in area is matched by a dramatic decrease in thickness. From 1975 to 2000 the average thickness of Arctic sea ice decreased by 33 percent, from 3.7 to 2.5 m (12.3 to 8.3 ft) (Rothrock et al. 2008). Ocean Acidification About one-third of the carbon dioxide emitted by human activity has already been taken up by the oceans, thus moderating the increase of carbon dioxide concentration in the atmosphere and global warming. But, as the carbon dioxide dissolves in sea water, carbonic acid is formed, which has the effect of acidifying, or lowering the pH, of the ocean (Orr et al. 2005). Although not caused by warming, acidification is a result of the increase of carbon dioxide, the same major greenhouse gas that causes warming. Ocean acidification has many impacts on marine ecosystems. To date, laboratory experiments have shown that although ocean acidification may be beneficial to a few species, it will likely be highly detrimental to a substantial number of species ranging from corals to lobsters and from sea urchins to mollusks (Raven et al. 2005; Doney et al. 2008; Fabry et al. 2008). Causes of climate change Both natural variability and human activities are contributing to observed global and regional warming, and both will contribute to future climate trends. It is very likely that most of the observed warming for the last 50 years has been due to the increase in greenhouse gases related to human activities (in IPCC reports, âvery likelyâ specifically means that scientists believe the statement is at least 90 percent likely to be true; âlikelyâ specifically means about two-thirds to 90 percent likely to be true [IPCC 2007b]). While debate over details is an important part of the scientific process, the climate science community is virtually unanimous on this conclusion. The physical processes that cause climate change are scientifically well documented. The basic physics of the way greenhouse gases warm the climate were well established by Tyndall, Ahrrenius, and others in the 19th century (Bolin 2007). The conclusions that human actions have very likely caused most of the recent warming and will likely cause more in the future are based on the vast preponderance of accumulated scientific evidence from many different kinds of observations (IPCC 2007b). Since the beginning of the Industrial Revolution, human activities that clear land or burn fossil fuels have been injecting rapidly increasing amounts of greenhouse gases such as carbon dioxide (CO2) and methane (CH4) into the atmosphere. In 2006 emissions of CO2 were about 36 billion metric tons (39.6 billion English tons), or about 5.5 metric tons (6.0 English tons) for every human being (Raupach et al. 2007). In the United States average CO2
Introduction 9 emissions in 2006 were approximately 55 kg (120 lb) per person per day. As a consequence of these emissions, atmospheric CO2 has increased by about 35 percent since 1850. Scientists know that the increases in carbon dioxide in the atmosphere are due to human activities, not natural processes, because they can fingerprint carbon dioxide (for example, by the mix of carbon isotopes it contains, its spatial pattern, and trends in concentration over time) and identify the sources. Concentrations of other greenhouse gases have also increased, some even more than CO2 in percentage terms (Figure 5). Methane, which is 25 times more effective per molecule at trapping heat than CO2, has increased by 150 percent. Nitrous oxide (N2O), which is nearly 300 times more effective per molecule than CO2 at trapping heat, has increased by over 20 percent (Forster et al. 2007). Scientific knowledge of climate is far from complete. Much remains to be learned about the factors that control the sensitivity of climate to increases in greenhouse gases, rates of change, and the regional outcomes of the global changes. These uncertainties, however, concern the details and not the core mechanisms that give scientists high confidence in their basic conclusions.
10 Introduction Atmospheric concentrations of CO2, CH4 and N2O over the last 10,000 years (large panels) and since 1750 (inset panels). Measurements are shown from ice cores (symbols with different colors for different studies) and atmospheric samples (red lines). The corresponding radiative forcings (amount of energy trapped per unit area) relative to 1750 are shown on the right hand axes of the large panels. Source: IPCC 2007d. FIGURE 5: Historical concentrations of greenhouse gasses CO2, CH4, and N2O over the past 10,000 years. For each of these greenhouse gases, the characteristic âhockey stickâ shape of the
Introduction 11 curve is the result of large increases in the concentrations of these gases very recently, compared to their relatively stable levels over the past 10,000 years. SOURCE: IPCC 2007d. What do we expect from future climate change? Evidence of rising atmospheric and ocean temperatures, changing precipitation patterns, rising sea levels, and decreasing sea ice is already clear. Average temperatures will almost certainly be warmer in the future. The amount of future climate change depends on human actions. A large number of experiments with climate models indicate that if the world continues to emphasize rapid economic development powered by fossil fuels, it will probably experience dramatic warming during the 21st century. For this kind of âbusiness as usualâ future the IPCC (IPCC 2007b) projects a likely range of global warming over 1990 levels of 2.4-6.4ºC (4.3-11.5ºF) by 2100 (Figure 6, scenario A1F1). If greenhouse gas emissions grow more slowly, peak around the year 2050, and then fall, scientists project a likely warming over 1990 levels of 1.1-2.9ºC (2.0- 5.2ºF) by 2100 (Figure 6, scenario B1).5 Temperature increases at the high end of the range of possibilities are very likely to exceed many climate thresholds. Warming of 6°C (10.8°F) or more (the upper end of the projections that the 2007 IPCC rates as âlikelyâ) would probably have catastrophic consequences for lifestyles, ecosystems, agriculture, and other livelihoods, especially in the regions and populations with the least resources to invest in adaptationâthat is, the strategies and infrastructure for coping with the climate changes. Warming to the high end of the range would also entail a global average rate of temperature change that, for the next century or two, would dramatically exceed the average rates of the last 20,000 years, and possibly much further into the past. Mean seawater temperatures in some U.S. coastal regions have increased by as much as 1.1°C (2°F) during the last half of the 20th century and, based on IPCC model projections of air temperature, are likely to increase by as much as 2.2-4.4°C (4-8°F) during the present century. âBusiness as usualâ emissions through 2100 would likely lead to oceans with surface temperatures that are 2-4ºC (3.6-7.2ºF) higher than now and surface waters so acidified that only a few isolated locations would support the growth of corals (Cao et al. 2007). Most marine animals, especially sedentary ones, and plants are expected to be significantly stressed by these changes (Hoegh-Guldberg et al. 2007). Some may be able to cope with either increased temperatures or more acidic waters, but adjusting to both may not be feasible for many species. 5 Projections of warming are given as a range of temperatures for three reasons. First, gaps in the scientific understanding of climate limit the accuracy of projections for any specific concentration of greenhouse gases. Changes in wind and clouds can increase or decrease the warming that occurs in response to an increase in the concentration of greenhouse gases. Loss of ice on the sea or snow on land increases the amount of the incoming sunlight that is absorbed, amplifying the warming from greenhouse gases. Second, the pattern of future emissions and the mix of compounds released to the atmosphere cannot be predicted with high confidence. Some kinds of compounds that produce warming remain in the atmosphere only a few days (Ramanathan et al. 2007). Others, like CO2, remain for centuries and longer (Matthews and Caldeira 2008). Still other compounds tend to produce aerosols or tiny droplets or particles that reflect sunlight, cooling the climate. Third, there is substantial uncertainty about the future role of the oceans and ecosystems on land. In the past, oceans and land ecosystems have stored, at least temporarily, about half of the carbon emitted to the atmosphere by human actions. If the rate of storage increases, atmospheric CO2 will rise more slowly. If it decreases, then atmospheric CO2 will rise more rapidly (Field et al. 2007).
12 Introduction Continued emissions under the âbusiness as usualâ scenario could lead by 2100 to 0.6 m (2 ft) or more of sea-level rise. Continuation of recent increases in loss of the ice caps that cover Greenland and West Antarctica could eventually escalate the rate of sea-level rise by a factor of 2 (Overpeck et al. 2006; Meehl et al. 2007; Alley et al. 2005; Gregory and Huybrechts 2006; Rahmstorf 2007). There will also be hotter extreme temperatures and fewer extreme cold events. An increase in climate variability, projected in some models, will entail more frequent conditions of extreme heat, drought, and heavy precipitation. A warmer world will experience more precipitation at the global scale, but the changes will not be the same everywhere. In general, the projections indicate that dry areas, especially in the latitude band just outside the tropics (for example, the southwestern United States), will tend to get drier on average (IPCC 2007b; Kunkel et al. 2008). Areas that are already wet, especially in the tropics and closer to the poles, will tend to get wetter on average. Increased climate variability and increased evaporation in a warmer world could both increase the risk and likely intensity of future droughts. Changes in the frequency or intensity of El Niño events forecast by climate models are not consistent (IPCC 2007b). El Niños are important because they are often associated with large-scale drought and floods in the tropics and heavy rains just outside the tropics, but projecting how the interaction between climate change and El Niño events will affect precipitation patterns is difficult. Another example of inconsistent results from models is that model simulations indicate that future hurricane frequency and average intensity could either increase or decrease (Emanuel et al. 2008), but it is likely that rainfall and top wind speeds in general will increase in a world of warmed ocean temperatures. For all of these different factorsââtemperature, precipitation patterns, sea-level rise and extreme eventsââboth the magnitude and speed of change are important. For both ecosystems and human activities, a rapid rate of climate change presents challenges that are different from, but no less serious than, the challenges from a large amount of change (Schneider and Root 2001).
Introduction 13 Solid lines are multi-model global averages of surface warming for scenarios A2, A1B and B1, shown as continuations of the 20th-century simulations. These projections also take into account emissions of short-lived GHGs and aerosols. The pink line is not a scenario, but is for Atmosphere-Ocean General Circulation Model (AOGCM) simulations where atmospheric concentrations are held constant at year 2000 values. The bars at the right of the figure indicate the best estimate (solid line within each bar) and the likely range assessed for the six SRES marker scenarios at 2090-2099. All temperatures are relative to the period 1980-1999. SOURCE IPCC 2007b. FIGURE 6 Projected future temperatures. This figure shows projected trends of average global surface temperature, based on output from all of the major climate models, shown as continuations of the 20th century observations (with the average for 1980-1999 plotted as 0). The pink line represents what would happen if CO2 concentrations could be held constant at year 2000 levels. Scenarios B1, A1B and A2 represent alternative possible futures. A1B and B1 are futures with modest population growth, rapid economic growth, and a globally integrated economy, with A1B focusing on manufacturing and B1 focusing on service industries. A2 is a world with more rapid population growth but slower economic growth and less economic integration. The bars to the right of the graph represent the likely range of average global temperature from the same models in the years 2090-2099 for a wider range of possible futures, with the horizontal bar in the middle indicating the average across the models. As of 2006, actual CO2 emissions were higher than those in the A2 scenario, making the full range of scenarios look like underestimates, at least for the first years of the 21st century. (IPCC 2007b, Raupach et al. 2007).
14 Introduction Climate change can impact ecosystems in many ways Hundreds of studies have documented responses of ecosystems, plants, and animals to the climate changes that have already occurred (Parmesan 2006; Rosenzweig et al. 2007). These studies demonstrate many direct and indirect effects of climate change on ecosystems. Changes in temperature, for example, have been shown to affect ecosystems directly: the date when some plants bloom is occurring earlier in response to warmer temperatures and earlier springs. Extreme temperatures, both hot and cold, can be important causes of mortality, and small changes in extremes can sometimes determine whether a plant or animal survives and reproduces in a given location. Changes in temperature, especially when combined with changes in precipitation, can have indirect effects as well. For many plants and animals soil moisture is critically important for many life processes; changes in precipitation and in the rate of evaporation interact to determine whether moisture levels remain at a level suitable for various organisms. For fish and other aquatic organisms both water temperature and water flow are important and influenced by the combined effects of altered air temperatures and precipitation. For example, warmer, drier years in the northwestern United States, often associated with El Niño events and anticipated to be more common under many climate scenarios, have historically been associated with below- average snowpack, stream flow, and salmon survival (Mote 2003). Some salmon populations are especially sensitive to summer temperatures; others are sensitive to low stream-flow volumes in the fall (Crozier and Zabel 2006). The fact that climate change leads to rising seas means that organisms and ecosystems located in coastal zones between the ocean and terrestrial habitats are squeezed, especially when the coastal land is occupied by buildings or crops. The ecological impacts of climate change are not inherently beneficial or detrimental for an ecosystem. The concept that a change is beneficial or detrimental has meaning mainly from the human perspective. For an ecosystem, responses to climate change are simply shifts away from the state prior to human-caused climate change. Measured by particular ecosystem services, some changes could be beneficial; for example, warmer temperatures extend the growing season in some latitudes, and higher CO2 levels increase the growth of some land plants, with higher potential yields of food and forestry products (Nemani et al. 2003). Others are detrimental, for example, western mountain areas with a longer snow-free season are experiencing increased wildfires, reduced potential wood harvests, and loss of some recreational opportunities (Westerling et al. 2006). In some settings uncertainty about future ecosystem services may be a cost in itself, motivating investments that may not turn out to be necessary or that may be insufficient to effectively address changing needs. To date, many species have responded to the effects of climate change by extending their range boundaries both toward the poles (for example, northward in the U.S.) and up in elevation, and by shifting the timing of spring and autumn events. Plants and animals needing to move but prevented from doing so, for example, because appropriate habitat is not present at higher elevations, are at greater risk of extinction. Shifting species ranges, changes in the timing of biological events, and a greater risk of extinction all affect the ability of ecosystems to provide the critical servicesâproducts, regulation of the environment, enhanced human quality of life, and natural infrastructureâthey have been providing.
Introduction 15 Ecosystems can adjust to changeâover time Ecosystems are not static. They are collections of living organisms that grow and interact and die. Ecosystems encounter an ever changing landscape of weather conditions and various kinds of disturbances, both subtle and severe. Whatever conditions an ecosystem encounters, the individual organisms and species react to the changes in different ways. Ecosystems themselves do not move, individuals and species do; some species can move farther and faster than others, but some may not be able to move at all. For example, a long-lived tree species may take decades to spread to a new range, while an insect with many hatches per year could move quickly. A species that already lives on mountaintops may have nowhere else to retreat. Rapid and extreme disturbances can have major and long-lasting ecological impacts. For example, a severe drought, wildfire, or hurricane can fundamentally reshape an area, often for many decades. In one of the most dramatic examples the impact of an asteroid 65 million years ago is believed to have so radically changed conditions on Earth that the dominant animals, the dinosaurs, died off and were supplanted by mammals (Alvarez et al. 1990). On longer time scales, most places on Earth have experienced substantial climate changes. During the peak of the last ice age, approximately 21,000 years ago, most of Canada and the northern United States were under thousands of feet of ice (Jansen et al. 2007). Arctic vegetation thrived in Kentucky, and sea levels were about 120 m (400 ft) lower than at present. Over the past million years Earth has experienced a series of ice ages, separated by warmer conditions. Global average temperatures during these ice ages were about 4-7°C (7.2-12.6°F) cooler than present, with the cooling and warming occurring over many thousands of years (Jansen et al. 2007). These ice ages triggered extensive ecological responses, including large shifts in the distributions of plants and animals, as well as extinctions. The massive changes during past ice ages certainly pushed ecosystems off large swaths of Earthâs surface as ice- dominated landscapes advanced. However, these changes were generally slow enough that surviving species could move and reassemble into novel, as well as familiar-looking, ecosystems as the ice retreated (Pitelka et al. 1997; Overpeck et al. 2003). The 10,000 years since the last ice age have seen substantial regional and local climate variation, but on a global scale climate was relatively stable, and these regional climate changes did not drive species to extinction nor result in the scale of global ecosystem change seen during glacial-to-interglacial transitions. Even when the global climate is not changing noticeably, regional climate variability (droughts, storms, and heat waves) can have dramatic regional (often short-term) impacts. In a period of climate change it is important to remember that this climate variability will continue to occur on top of the more long-term human-caused climate changes. Data on ecosystem responses to disturbances in the distant past can provide valuable information about likely responses to current and future climate change. But it is important to recognize that the current rate of increase of CO2 in Earthâs atmosphere is faster than at any time measured in the past, indicating that human-caused global climate change in the current era is likely to be exceedingly rapid, many times faster than the long-term global changes associated with onset and termination of the ice ages (Jansen et al. 2007). One of the big concerns about the future is that climate changes in some places may be too fast for organisms to respond in the ways that have helped sustain ecosystem services in response to natural changes in the past. Understanding how quickly ecosystems can and cannot adjust is one of the key challenges in climate change research.
16 Introduction Climate change, other stresses, and the limits of ecosystem resilience Climate change is not the only way humans are affecting ecosystems. Humans have a large and pervasive influence on the planet. We use a substantial portion of the land for agriculture and the oceans for fishing (Worm et al. 2006; Ellis and Ramankutty 2008). Many rivers are dammed to provide water for crops or people, or they are polluted with fertilizer or other chemicals. Chemical residues and the by-products of industrial activity, from acid precipitation to ozone, affect plant growth. Human activities, especially land and ocean use, limit some opportunities for species migrations while opening routes for other species. Globally humans have moved many non-native species from one ecosystem to another. Ecosystems operate in a context of multiple human influences and interacting factors. Earthâs ecosystems are generally resilient to some range of changes in climate. A resilient ecosystem is one that can withstand a stress like pollution or rebuild after a major disturbance like a serious storm. A resilient ecosystem can cope with a drought or an unusually hot summer in ways that alter some aspects of ecosystem function but do not lead to a major shift in the type of ecosystem or the services it provides. Thus, a resilient ecosystem may not appear to be affected by modest or slow climate changes. But this resilience has limits. When a change exceeds those limits, or is coupled with other simultaneous changes that cause stress, the ecosystem undergoes a major change, often shifting to a fundamentally different ecosystem type. There is a threshold point when dramatic ecosystem transformations may occur (Gunderson and Pritchard 2002). These thresholds are like the top of a levee as the water level rises. As long as the water level is even slightly below the top of the levee, function is normal. But once it rises above the levee, there is a flood. This kind of threshold response is common in ecosystems, where extreme events like heat waves often serve as triggers for an irreversible transition of the ecosystem to a new state. Currently plants and animals are responding to rapid climate change while simultaneously coping with other human-created stresses such as habitat loss and fragmentation due to development, pollution, invasive species, and overharvesting. How do we know climate change itself is causing major changes in ecosystems? First, species changing their ranges in the Northern Hemisphere are almost uniformly moving their ranges northward and up in elevation in search of cooler temperatures (Parmesan and Yohe 2003; Parmesan 2006; Rosenzweig et al. 2007). If any or all of the other stressors were the major cause of ecosystem changes, plants and animals would move in many directions in addition to north, and to lower as well as higher elevations. Second, when we look at the association over time of changes between species ranges and temperatures modeled using only natural variation in climate, such as sunspots and volcanic dust in the stratosphere, the relationship is poor. When temperatures are modeled using natural variability as well as human-caused drivers, such as emission of CO2 and methane, the association is very strong. Consequently, humans are very likely causing changes in regional temperatures to which in turn the plants and animals are responding (Root et al. 2005).
The world's climate is changing, and it will continue to change throughout the 21st century and beyond. Rising temperatures, new precipitation patterns, and other changes are already affecting many aspects of human society and the natural world.
In this book, the National Research Council provides a broad overview of the ecological impacts of climate change, and a series of examples of impacts of different kinds. The book was written as a basis for a forthcoming illustrated booklet, designed to provide the public with accurate scientific information on this important subject.
READ FREE ONLINE
Welcome to OpenBook!
You're looking at OpenBook, NAP.edu's online reading room since 1999. Based on feedback from you, our users, we've made some improvements that make it easier than ever to read thousands of publications on our website.
Do you want to take a quick tour of the OpenBook's features?
Show this book's table of contents , where you can jump to any chapter by name.
...or use these buttons to go back to the previous chapter or skip to the next one.
Jump up to the previous page or down to the next one. Also, you can type in a page number and press Enter to go directly to that page in the book.
To search the entire text of this book, type in your search term here and press Enter .
Share a link to this book page on your preferred social network or via email.
View our suggested citation for this chapter.
Ready to take your reading offline? Click here to buy this book in print or download it as a free PDF, if available.
Get Email Updates
Do you enjoy reading reports from the Academies online for free ? Sign up for email notifications and we'll let you know about new publications in your areas of interest when they're released.
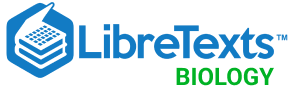
- school Campus Bookshelves
- menu_book Bookshelves
- perm_media Learning Objects
- login Login
- how_to_reg Request Instructor Account
- hub Instructor Commons
- Download Page (PDF)
- Download Full Book (PDF)
- Periodic Table
- Physics Constants
- Scientific Calculator
- Reference & Cite
- Tools expand_more
- Readability
selected template will load here
This action is not available.
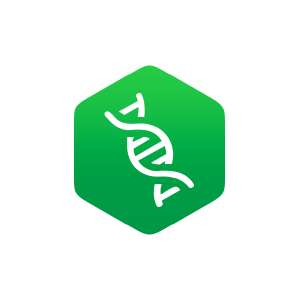
24.3: Current and Future Climate Change Impacts
- Last updated
- Save as PDF
- Page ID 118177
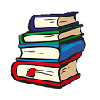
- Tara Jo Holmberg
- Northwestern Connecticut Community College
Current and Future Climate Change Impacts
- Please read and watch the following Learning Resources
- Reading the material for understanding, and taking notes during videos, will take approximately 1 hour.
- Optional Activities are embedded.
- If on a mobile device, use the Contents menu at the top of the page OR the links at the bottom of the page.
Learning Objectives
- Identify current global climate impacts and their confidence level
- Explain several projected abiotic climate impacts and their effects on living organisms
Introduction
Climate change has the potential to render Earth unrecognizable from what any human has ever experienced. These changes will have an immense impact on ecosystem services, global economies, and our own quality of life. Yet, while there is much talk about these risks, there is still too little action addressing its main causes.
Current Climate Change Impacts
Some of the lack of action may be attributed to “climate change” and “future” often being used in the same sentence, giving politicians and industries a false impression that we can deal with climate change once we achieved sufficient economic growth. The reality could however not be further from the truth, as we already see signs of the changes to come here today (Table \(\PageIndex{1}\)), including near-annual crop failures, record-high temperatures, and record-strength coastal storms.
Terms from Table \(\PageIndex{1}\)
Risk : provides a framework for understanding the increasingly severe, interconnected, and often irreversible impacts of climate change on ecosystems, biodiversity, and human systems; differing impacts across regions, sectors, and communities; and how to best reduce adverse consequences for current and future generations.
Adaptation : in human systems, the process of adjustment to actual or expected climate and its effects in order to moderate harm or take advantage of beneficial opportunities. In natural systems, adaptation is the process of adjustment to actual climate and its effects; human intervention may facilitate this.
Resilience : the capacity of social, economic, and ecosystems to cope with a hazardous event, trend, or disturbance, responding, or reorganizing in ways that maintain their essential function, identity, and structure as well as biodiversity in case of ecosystems while also maintaining the capacity for adaptation, learning, and transformation.
Mitigation: reducing emissions, and stabilizing the levels, of heat-trapping greenhouse gases in the atmosphere.
Confidence : each finding is grounded in an evaluation of underlying evidence and agreement. A level of confidence is expressed using five qualifiers: very low, low, medium, high and very high.
Between June 25 and July 1, 2021, British Columbia experienced a massive heat dome with temperatures reaching 49.6 o C in some areas. Scientists have stated that climate change was a contributing factor. More than 500 deaths were attributed to the heat. Glaciers throughout British Columbia are in retreat, with losses ranging from 6% to 34% from 1985-2005, and their melting is increasing. See more from the British Columbia Provincial Government: Change in Size of B.C. Glaciers .
A summary of the impacts of climate change on natural disasters is given in Figure \(\PageIndex{1}\). The major types of disasters related to climate are floods and storms, but the health implications of extreme temperatures are also becoming a great concern. In the decade 1971 to 1980, extreme temperatures were the fifth most common natural disasters; by 2001 to 2010, they were the third most common.
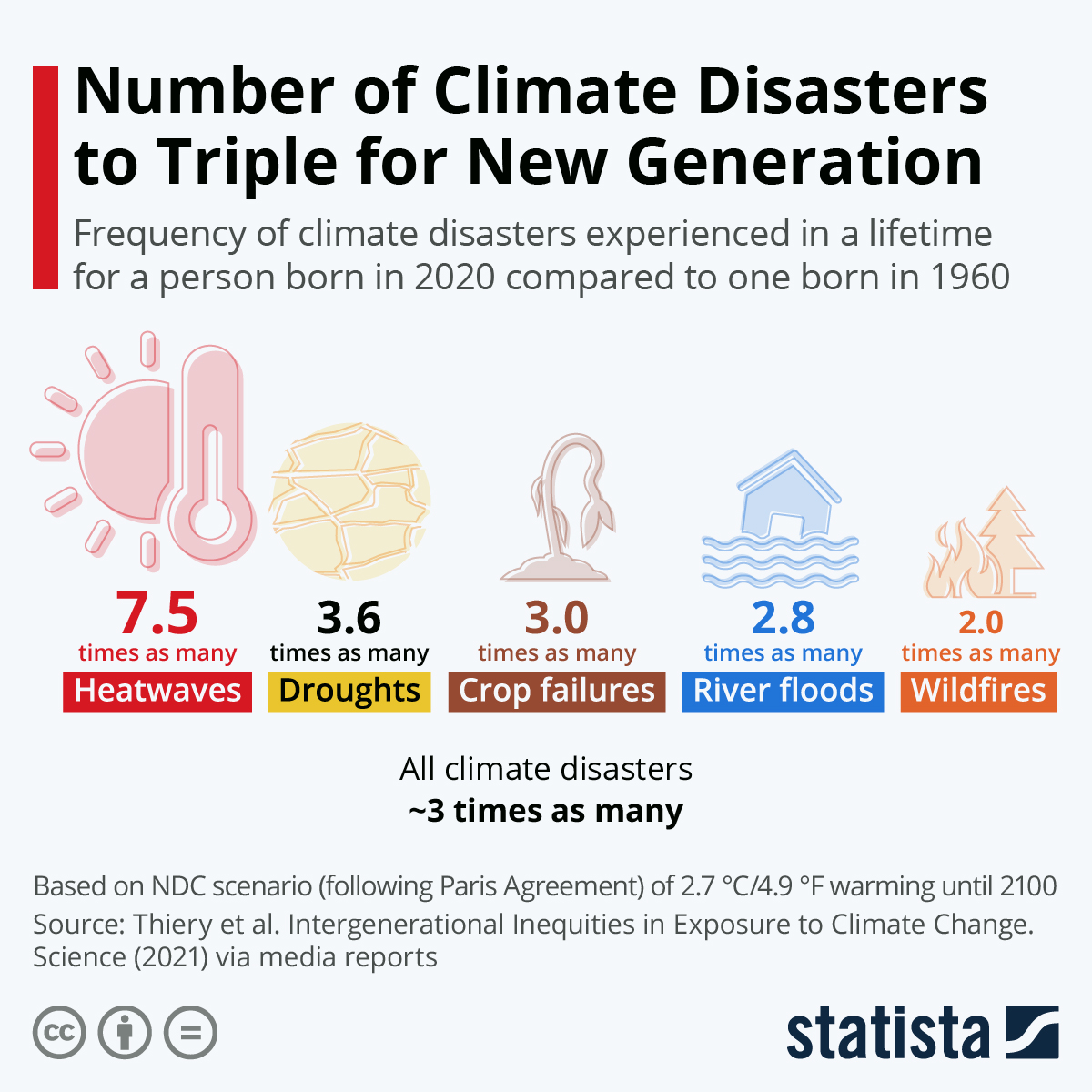
Canadian Perspective - The Mountain Pine Beetle
"The mountain pine beetle ( Dendroctonus ponderosae Hopkins) is native to western North America, from northern Mexico to northern British Columbia. It is also present in an isolated population of pine that is surrounded by prairie in the Cypress Hills area of southwestern Saskatchewan, which was likely infested in the early 1980s. In the 2000s, the beetle significantly expanded its range in Canada, invading new habitat east of the Rocky Mountains in northeastern British Columbia and northern Alberta (Figure \(\PageIndex{2}\) left)." 1
"Most species of pine that grow in the beetle’s range are readily attacked, with the exception of Jeffrey pine. In Canada, these hosts include ponderosa, western white, whitebark, limber pines, and, very recently, jack pine in the expanded range. Lodgepole pine is the most common host in the mountain pine beetle’s range in British Columbia." 1
"This small beetle attacks and kills mature trees by boring through the bark and mining the phloem (living tissue in vascular plants that carries organic nutrients). Its eggs hatch into larvae that consume the phloem, killing the tree. The beetles are killed by very cold winter weather, which historically has kept their numbers in check." 2
"Trees will attempt to repel the beetles by releasing quantities of resin, which mixes with the boring dust and forms a soft, white or reddish pitch tube around each borehole. These pitch tubes usually appear from the duff line to a top diameter of 15cm. Infected trees' foliage turns yellow and then red by the spring of the year following the initial beetle attack. Most of the needles drop from the tree after two years, leaving a dead grey snag." 2
"In British Columbia, the northern limit of the beetle’s range has been limited by cold winter temperatures (−40°C) and cool summers. Outbreaks have been linked to favorable weather in both summer and winter. Warm, dry summers are good for beetle development and dispersal, and drought stress reduces tree defenses. In the late 1990s, after several relatively warm winters, a massive outbreak resulted in the loss of millions of hectares of pine forest in British Columbia over the next 15 years." 1,2
1. Government of Canada. 2021. Mountain pine beetle (factsheet) . Natural Resources Canada [Online]. Available from: https://www.nrcan.gc.ca/forests/fire-insects-disturbances/top-insects/13397
2. Government of British Columbia. 2022. Mountain pine beetle. Forest Health [Online]. Available from: https://www2.gov.bc.ca/gov/content/industry/forestry/managing-our-forest-resources/forest-health/forest-pests/bark-beetles/mountain-pine-beetle
Future Climate Change
Many greenhouse gases stay in the atmosphere for long periods of time. As a result, even if emissions stopped increasing, atmospheric greenhouse gas concentrations would continue to remain elevated for hundreds of years. Moreover, if we stabilized concentrations and the composition of today’s atmosphere remained steady (which would require a dramatic reduction in current greenhouse gas emissions), surface air temperatures would continue to warm (Figure \(\PageIndex{3}\)). This is because the oceans, which store heat, take many decades to fully respond to higher greenhouse gas concentrations. The ocean’s response to higher greenhouse gas concentrations and higher temperatures will continue to impact climate over the next several decades to hundreds of years. Thus, past and present GHG emissions will affect climate far into the future.
Figure \(\PageIndex{3}\): Temperature increases have been most pronounced in northern latitudes and over land masses. The colors represent the temperature difference between the 2011-2020 average and the 1951-1980 baseline, with warmer colors (yellow, orange, red) representing increases, and cool colors (green, blue) representing decreases. The image uses longer term averages of at least a decade to smooth out climate variability due to factors such as El Niño. Grey areas in the image have insufficient data for rendering. Image and caption (modified) from NASA’s Scientific Visualization Studio/Eric Fisk (public domain).
Temperature Changes
Climate models project the following key temperature-related changes:
- Average global temperatures are expected to increase by 1.1°C to 6.4°C by 2100, depending on the level of future greenhouse gas emissions, and the outcomes from various climate models.
- By 2100, global average temperature is expected to warm at least twice as much as it has during the last 100 years.
- Ground-level air temperatures are expected to continue to warm more rapidly over land than oceans.
- Some parts of the world are projected to see larger temperature increases than the global average.
These changes will impact our food supply, water resources, infrastructure, ecosystems, and even our own health. The magnitude and rate of future climate change will primarily depend on the following factors:
- The rate at which levels of greenhouse gas concentrations in our atmosphere continue to increase, often known as "climate scenarios" (Figure \(\PageIndex{4}\));
- How strongly features of the climate (e.g., temperature, precipitation, and sea level) respond to the expected increase in greenhouse gas concentrations; and
- Natural influences on climate (e.g., from volcanic activity and changes in the sun’s intensity) and natural processes within the climate system (e.g., changes in ocean circulation patterns).

Species that are highly mobile are not entirely spared from the negative impacts of climate change. Consider that migratory species rely on environmental cues, such as day length and temperature, to determine when to start moving from one area to the next. But because different species rely on different environmental cues to time their life cycles (e.g. breeding), not all species will adjust to climate change at the same rate. There is consequently a high likelihood that climate change will disrupt these synchronous movements that the animals in particular have developed over thousands to millions of years. This disruption of timed aspects of a species’ life cycle, such as migration and breeding, is called phenological mismatch . Researchers have already seen signs of phenological mismatch : some migratory birds that overwinter in the tropics have started to migrate to their breeding grounds at earlier dates than before. If these trends hold, they may soon start breeding before peak food availability, which could lead to lower fitness of offspring.
Resident species are also vulnerable to phenological mismatch. While these species might not be known for large-scale movements around the globe, they may still have to adjust their ranges to keep track of their climatic niches. Considering the improbability that different species will all adapt at the same pace, there is thus a danger that important mutualistic relationships might be pulled apart during these range adaptations. This is of concern for species with specialized feeding niches, as seen in some pollinators. Extinctions arising from this decoupling of mutualistic relationships are referred to as coextinction .
Freshwater ecosystems will generally experience temperature increases under climate change. Like their terrestrial counterparts, many freshwater species are sensitive to temperature shifts as warmer water also holds less dissolved oxygen. In addition, longer growing seasons and higher water temperatures will lead to increased nutrient loads, algae blooms, and eutrophication. All these factors will force many freshwater species—even those not sensitive to temperature shifts—to adjust their ranges to keep track of suitable conditions. For some freshwater species, the impediments to adjusting their ranges as necessary may be insurmountable.
Not all species will be impacted negatively. A variety of species are expected to benefit from climate change. These include generalist species currently limited by interactions with localized specialists that are—at least at present—better competitors for limiting resources. Some tropical species may thrive as their habitats become hotter and wetter. Species with high genetic diversity that reproduce quickly (allowing for rapid adaption to environmental changes) are also likely to benefit. Unfortunately, many species that exhibit these traits carry diseases and/or are agricultural pests. For example, populations of the coffee berry borer ( Hypothenemus hampei )—Africa’s most notorious coffee pest—are expected to greatly increase in a warmer world. This growing threat is particularly worrying given that higher temperatures have already reduced coffee harvests in countries such as Tanzania by as much as 50%.
The range of disease-carrying insects and the viruses and pathogenic parasites they harbor will increase. This spread has already been documented in North America with several different diseases including West Nile and Lyme disease. Colder temperatures typically limit the distribution of certain species, such as the mosquitoes that transmit malaria, because freezing temperatures destroy their eggs. Not only will the range of some disease-causing insects expand, but increasing temperatures will also accelerate the host lifecycles, allowing them to breed and multiply more quickly. They may even evolve pesticide resistance faster. In addition to those listed above, other diseases such as dengue fever, malaria, yellow fever, zika virus, and chikungunya are expected to spread to new regions as the global climate warms.
Climate change does not only increase the spread of diseases in humans. Rising temperatures are associated with greater amphibian mortality due to chytridiomycosis (see Unit 5.2.3 ). Similarly, warmer temperatures have exacerbated bark beetle infestations of coniferous trees, such as pine and spruce.
Optional Activity \(\PageIndex{1}\)
How do climate factors (temperature, precipitation, & humidity) relate to vector-borne disease?
- Heavy rain increases the risk of animals transmitting pathogens.
- Milder winters increase the vector density & risk of disease
- Dry summers & drought increase the risk of vectors expanding their range
- More intense snowstorms Snow increase vector density & risk of disease
B. Milder winters increase the vector density & risk of disease.
Precipitation and Storm Events
Patterns of precipitation and storm events, including both rain and snowfall are likely to change. However, some of these changes are less certain than the changes associated with temperature. Because warm air is able to hold more water than cold air, the general global trend over the past century has been one of increasing precipitation (Figure \(\PageIndex{5}\)).
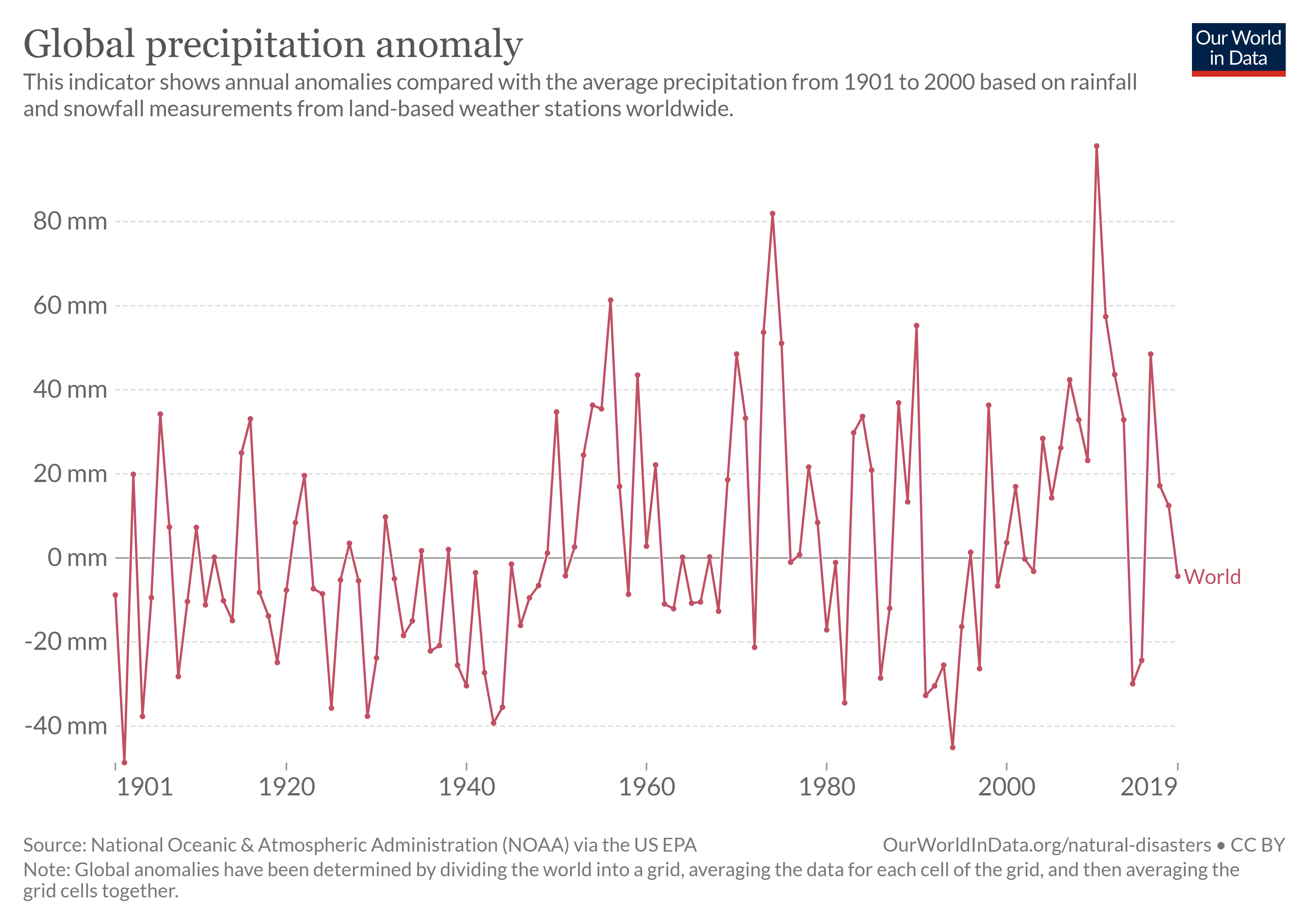
Figure \(\PageIndex{5}\): Data shows global precipitation patterns, based on rainfall and snowfall measurements from land-based weather stations worldwide. This indicator shows annual anomalies, or differences, compared with the average precipitation from 1901 to 2000. CC-BY United States Environmental Protection Agency (EPA)
Projections show that future precipitation and storm changes will vary by season and region. Some regions may have less precipitation, some may have more precipitation, and some may have little or no change. The amount of rain falling in heavy precipitation events is likely to increase in most regions, while storm tracks are projected to shift toward the poles. Climate models project the following precipitation and storm changes:
- Global average annual precipitation through the end of the century is expected to increase, although changes in the amount and intensity of precipitation will vary by region.
- The intensity of precipitation events will likely increase on average. This will be particularly pronounced in tropical and high-latitude regions, which are also expected to experience overall increases in precipitation.
- The strength of the winds associated with tropical storms is likely to increase. The amount of precipitation falling in tropical storms is also likely to increase.
- Annual average precipitation is projected to increase in some areas and decrease in others.
One of the other risks for coastal populations, besides sea-level rise, is that climate warming is also associated with an increase in the intensity of coastal storms (e.g., atmospheric rivers, hurricanes, cyclones, or typhoons), which almost always bring serious flooding from intense rain and storm surges. Some recent examples are the Vancouver floods of 2021 and the impact of Hurricane Fiona on Nova Scotia in 2022 (Figure \(\PageIndex{6}\)).
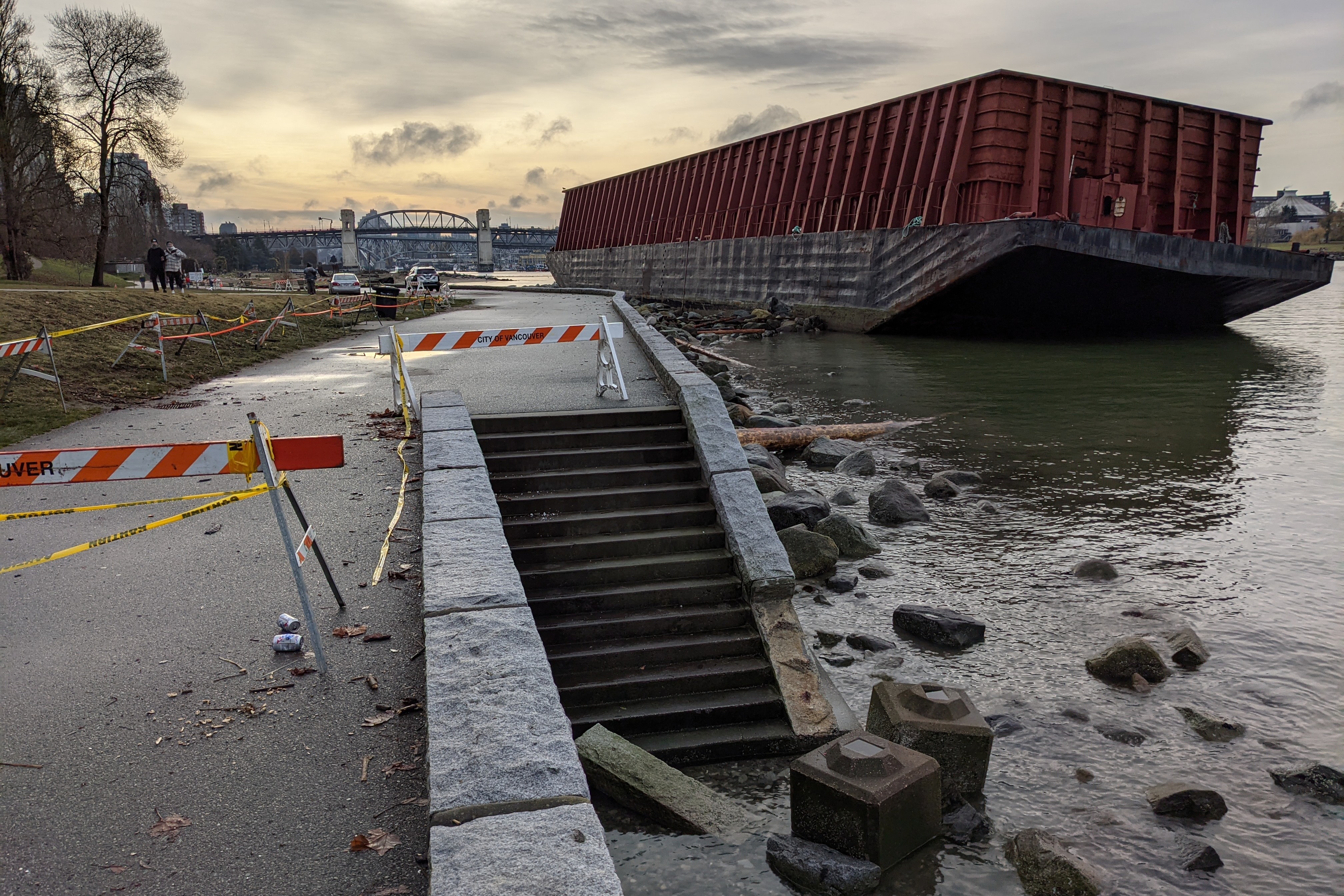
Changing precipitation levels will have several impacts on freshwater ecosystems, particularly as it relates to changes in their flow regimes. For example, areas that are undergoing decreased precipitation will experience decreased runoff and increased drying of wetlands and small streams, while areas with increased precipitation will experience increased storm surges and flushing. Given these multiple stressors, there is a reasonable expectation that many freshwater species will go extinct or face significant population declines and range shifts over the next decades. These changes are of major concern in countries that are currently majority subsistence, where so many people depend on fish and related natural resources for their livelihoods. Many communities around the world are already finding it more difficult to meet their nutritional needs due to climate-induced freshwater fish declines. Also, at some large lakes, which may supply 20–40% of the surrounding countries’ dietary protein—fish yields have decreased by 30% in recent years, also attributed to climate change.
Ice Melt and Sea Level Rise
Arctic sea ice is already declining. Sea ice is ice floating in the ocean (not on land like a glacier). Most sea ice is in the Arctic Ocean and the extent of sea ice is shrinking. Below, the NOAA animation shows how perennial sea ice has declined from 1990 to 2015. The oldest ice is white and the youngest (seasonal) ice is dark blue. The amount of old ice has declined from 20% in 1985 to 3% in 2015. For every 1°C of warming, models project about a 15% decrease in the extent of annually averaged sea ice (the total ice extent and concentration averaged over a year) and a 25% decrease in September Arctic sea ice.
This 2-minute video from the US's National Oceanic and Atmospheric Association illustrates the loss of Arctic sea ice from 1990-2015. Question after watching: What seasonal patterns do you notice? What do you notice regarding the oldest ice over the 25 years portrayed in the video?
The land-based, coastal sections of the Greenland and Antarctic ice sheets have been melting significantly and are expected to continue to do so (Figure \(\PageIndex{7}\)). If the rate of this ice melting increases in the 21st century, these ice sheets could add significantly to global sea level rise and disrupt global ocean currents. Before anthropogenic warming, glacial activity was variable with some retreating and some advancing. Now, nearly all glaciers globally a retreating and expected to continue to melt, leading to freshwater shortages globally.
On average, the sea is rising at a rate of 1.8 mm per year. However, between 1993 and 2010 the rate of sea level increase ranged between 2.9 and 3.4 mm per year (Figure \(\PageIndex{8}\)). This is thought largely to be from both the melting of glaciers and thermal expansion. Thermal expansion means that as objects such as solids, liquids, and gases heat up, they expand in volume. Since 1970, the melting of glaciers and thermal expansion account for 75% of the sea-level rise (scientists are still researching the other 25%). As the oceans creep further inland, the extent of low-lying coastal ecosystems such as rocky shores or sandy beaches will shrink, and so also the sizes of the wildlife populations living in those areas.
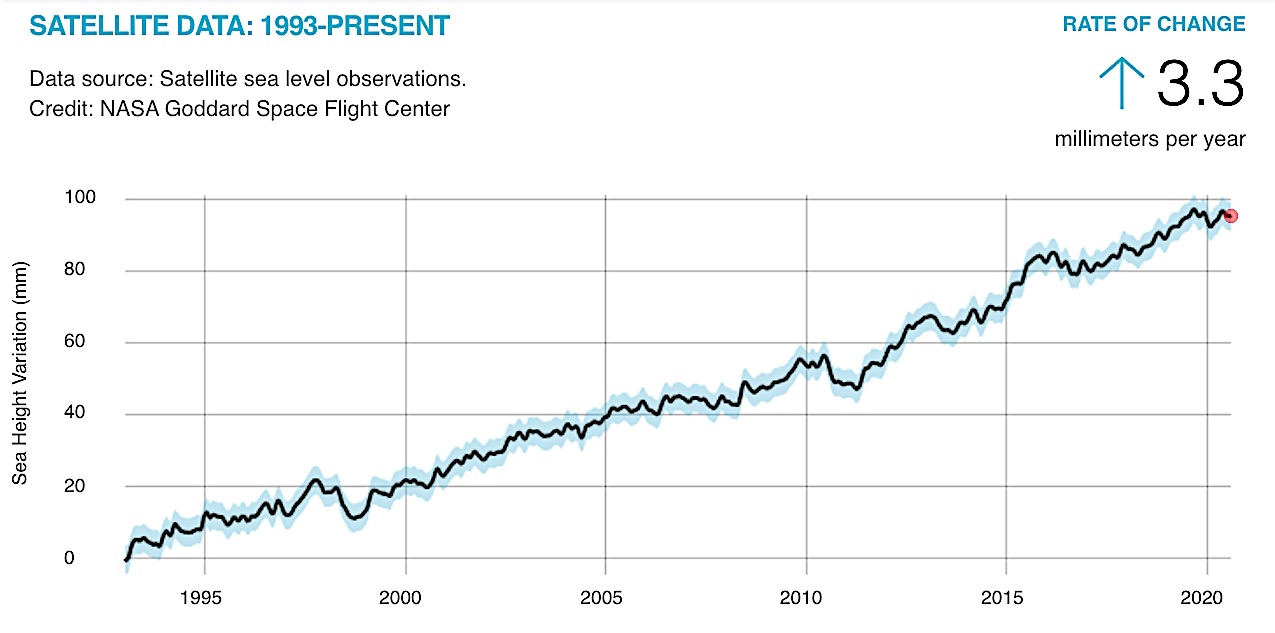
Projections for sea level rise to the end of this century vary widely. This is in large part because we do not know which of the above climate change scenarios (Figure \(\PageIndex{3}\)) we will most closely follow, but many are in the range from 0.5 m to 2.0 m. One of the problems in predicting sea level rise is that we do not have a strong understanding of how large ice sheets, such as Greenland and Antarctica, will respond to future warming. Another issue is that the oceans do not respond immediately to warming. For example, with the current amount of warming, we are already committed to a future sea level rise of between 1.3 m and 1.9 m, even if we could stop climate change today.
Optional Activity \(\PageIndex{2}\)
What are the major causes of sea level rise?
- Melting sea ice
- Melting glaciers and ice sheets
- Seawater expanding as it gets warmer
B and C. Melting glaciers and ice sheets and seawater expanding as it gets warmer.
Ocean Acidification and Deoxygenation
Since 1750, the ocean has absorbed about 30% of all anthropogenic carbon dioxide sources. When carbon dioxide gets absorbed in the ocean, it creates carbonic acid which makes the ocean more acidic. Ocean acidification is the process of ocean waters' decreasing pH (lower values are more acidic on the pH scale). The pH level of the oceans has decreased by approximately 0.1 pH units since pre-industrial times, which is equivalent to a 25% increase in acidity. The pH level of the oceans is projected to decrease even more by the end of the century as CO 2 concentrations are expected to increase for the foreseeable future.
Ocean acidification adversely affects many marine species, including plankton, mollusks, shellfish, and corals. As ocean acidification increases, the availability of calcium carbonate will decline. Calcium carbonate is a key building block for the shells and skeletons of many marine organisms. Most tropical oceans have experienced extensive coral bleaching events in recent years due to increased sea surface temperatures and ocean acidification. Ocean acidification also disturbs predator-prey dynamics by impairing the senses of prey species and compromising the ability of marine creatures to communicate with those within their same species.
Marine fish and invertebrates rely on dissolved oxygen that enters the water either through the atmosphere or by photosynthetic plankton. But because warmer water absorbs less oxygen, scientists predict that some areas of the ocean will see a 3–6% drop in dissolved oxygen concentrations under climate change. This process, known as ocean deoxygenation , will leave parts of the ocean unsuitable for marine fishes and invertebrates, leading to dead zones. The impact of ocean deoxygenation will also be felt by economically important fisheries where climate change is predicted to lead to fisheries-related economic losses upwards of $415 million each year.
Acidification combined with warmer temperatures and lower oxygen levels is expected to have severe impacts on marine ecosystems, especially coral reefs, and associated fisheries possibly affecting our ocean-derived food sources.
Optional Activity \(\PageIndex{3}\)
What causes ocean acidification?
- Deoxygenated ocean water.
- Ocean pollution.
- Warm freshwater from land.
- CO 2 dissolved in ocean water.
D. CO 2 dissolved in ocean water.
Climate Change Affects Everyone
We have all experienced the effects of climate change over the past decade. However, it is not straightforward for climatologists to make the connection between a warming climate and specific weather events, and most are justifiably reluctant to ascribe any specific event to climate change. In this respect, the best measures of climate change are those that we can detect over several decades, such as the temperature changes shown in Figure \(\PageIndex{2}\), or the sea level rise shown in Figure \(\PageIndex{7}\).
Our lives are connected to the climate . Human societies have adapted to the relatively stable climate we have enjoyed since the last ice age which ended several thousand years ago. A warming climate will bring changes that can affect our water supplies, agriculture, power and transportation systems, the natural environment, and even our own health and safety. Carbon dioxide can stay in the atmosphere for nearly a century, on average, so Earth will continue to warm in the coming decades. The warmer it gets, the greater the risk for more severe changes to the climate and Earth’s system. Although it is difficult to predict the exact impacts of climate change, what is clear is that the climate we are accustomed to is no longer a reliable guide for what to expect in the future.
Indigenous Connections: Climate Change Vulnerabilities
In 2019, the Tsleil-Waututh Nation published a report entitled, "Understanding Our Community’s Climate Change Vulnerabilities: Community Climate Change Resilience Planning | PHASE 1 Summary". As noted in the report, "Climate change has the potential to profoundly impact the Tsleil-Waututh Nation (TWN) and many key aspects of community life. It influences the ability of our community to harvest some wild foods and medicinal plants, how we access the shoreline and marine waters, and how we connect with Nature. It poses community health risks, influence our ability to practice spiritual and cultural ceremonies, and share cultural teachings...This report serves to build an understanding of community vulnerability to climate change and begins to explore potential climate adaptation actions" (Tsleil-Waututh Nation, 2019).
The project focused on "climate change impacts to Tsleil-Waututh, also known as Burrard Inlet IR#3, which is the current village site for the Nation. In the future, TWN hopes to expand the assessment to consider the vulnerability of other areas within its traditional territory" (Tsleil-Waututh Nation, 2019).
Below is a summary of some of what this report details as possible impacts of climate change on the Tsleil-Waututh Nation:
- Coastal flood hazard modeling results showed that most waterfront properties may experience flooding during an extreme flood under a 2 m sea level rise scenario.
- Coastal erosion may affect waterfront properties and natural habitats, and threaten buildings, archeological, and other cultural sites.
- Ocean acidification from climate change poses an acute threat to species (particularly shellfish) along the mudflats and beaches of Burrard Inlet IR#3.
- [Loss of intertidal areas] will have significant impacts on species that live in the intertidal zone (e.g., shellfish) or that forage for food in these areas (e.g., marine birds and salmon). Many of these species are critically important as sources of food for TWN community and cultural health and serve as important opportunities to share traditional teachings (Figure \(\PageIndex{9}\)).
- The waters of Burrard Inlet will be increasingly vulnerable to harmful algal blooms as water temperatures rise and summer precipitation decreases.
- Climate change has the potential to make local waters more habitable for invasive non-native species, particularly those from warmer waters.
- Extended periods of extreme heat can cause heat exhaustion or heat stroke, particularly among vulnerable groups.
- Community member health and the opportunity for recreation, cultural practices, and job productivity is also impacted by poor air quality and smoke from wildfires across BC.
- Ongoing changes to air temperatures and precipitation patterns from climate change could increase the success and abundance of some invasive species.
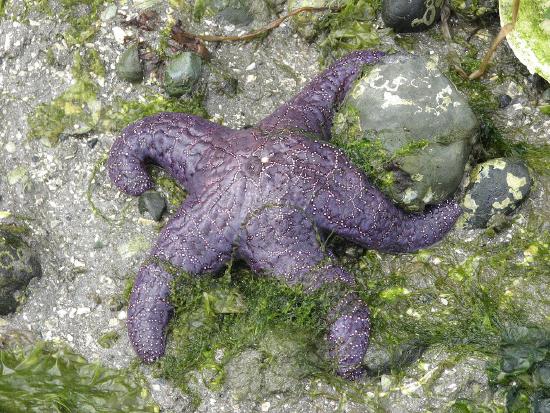
Tsleil-Waututh Nation. 2019. "Understanding Our Community’s Climate Change Vulnerabilities: Community Climate Change Resilience Planning | PHASE 1 Summary" British Columbia, Canada. Available from: https://twnation.ca/wp-content/uploa...e-Jan-2020.pdf
Learn how climate change will transform British Columbia by 2050 in this 3.5-minute video by CBC's Johanna Wagstaffe. Question after watching: Which of the changes will affect you directly? Which of the changes will affect you indirectly?
Suggested Supplementary Reading
Intergovernmental Panel on Climate Change. 2021. 6th Assessment: Summary for Policymakers.
NASA. 2022. Global Climate Change: Vital Signs of the Planet . This website by NASA provides a multi-media smorgasbord of engaging content. Learn about climate change using data collected by NASA satellites and more.
- Reference Manager
- Simple TEXT file
People also looked at
Policy brief article, in the climate emergency, conservation must become survival ecology.
- 1 Durrell Institute of Conservation and Ecology, University of Kent, Canterbury, United Kingdom
- 2 Faculty of Science and Technology, Bournemouth University, Poole, United Kingdom
Earth faces a climate emergency which renders conservation goals largely obsolete. Current conservation actions are inadequate because they (i) underplay biodiversity's role in maintaining human civilisation, which contributes to its marginalisation, and (ii) rely on false assumptions of how to catalyse transformative change. We suggest a paradigm shift from biodiversity conservation to survival ecology, refocusing the field on safeguarding a planetary system in which humans and other species can thrive. Rather than seeking to maintain a world which will no longer exist, survival ecology acknowledges unavoidable change and seeks to shape the world that will: it looks to the future, not the past. Since conservation science and advocacy have not been sufficient to achieve the required change, survival ecologists should additionally embrace non-violent civil disobedience.
Introduction
Climate change threatens to end human civilisation ( Richards et al., 2021 ), and almost 14,000 scientists have declared “clearly and unequivocally that planet Earth is facing a climate emergency” ( Ripple et al., 2021 ). An emergency is a critical situation requiring our immediate attention; in an emergency, we stop what we were doing and refocus on the urgent task at hand. However, like most of society, conservation stakeholders (including scientists, practitioners, policy-makers, and funders) have collectively failed to respond to the urgency of the emergency and adapt our objectives and approaches. Here, we argue that climate risks are so great as to render largely obsolete the customary conservation focus on preventing species extinctions and maintaining the current benefits people obtain from nature. Instead, we suggest that conservation aims may best be achieved by refocusing and reframing conservation around the safeguarding of an Earth system that supports both human civilisation and other life into the future, a paradigm we term “survival ecology.” We first make the case that near-future impacts of climate change on biodiversity will be so severe that the conservation movement needs to re-examine its fundamental objectives. We then argue that reframing conservation around a survival ecology agenda may be required to transition the field from its currently marginal status to the heart of economic and political decision-making. However, while our argument largely concerns conservation's framing, the transition to survival ecology also requires fundamental changes to conservation philosophies, objectives, practise, and theories of change, which we briefly outline in the following two sections. We do so in the hope of stimulating an urgently-needed debate, because without rapid adaptation to the climate emergency and reflection on its continued inability to catalyse the required changes in our economies and societies, conservation will fail to conserve global biodiversity.
Biodiversity in the Climate Emergency
Conservation is a diverse field encompassing a range of rationales and approaches ( Sandbrook et al., 2019 ). While rationales for conservation have become increasingly anthropocentric over recent decades, it remains largely centred (and, in particular, marketed) on the goal of maintaining biodiversity through the prevention of species extinctions (e.g., Bolam et al., 2020 ; Rounsevell et al., 2020 ). However, existing efforts have failed to prevent extensive anthropogenic biodiversity loss driven by habitat change, overharvesting, species introductions and cascading effects ( Secretariat of the Convention on Biological Diversity, 2020 ; Bradshaw et al., 2021 ), and an estimated one million species now face extinction ( Díaz et al., 2019 ). Moreover, the prospects for preventing further extinctions become ever more remote in the climate emergency, as an increasing body of evidence highlights the negative impacts of climate change on species and ecosystems in all biomes. For example, temperature increases and other climatic changes will render large portions of the current ranges of many species unsuitable within decades (e.g., Román-Palacios and Wiens, 2020 ; Trisos et al., 2020 ), but many species will be unable to adapt sufficiently ( Radchuck et al., 2019 ) or shift their ranges within these timeframes ( Román-Palacios and Wiens, 2020 ). This of course supposes the existence and accessibility of suitable niche space elsewhere, though this will be particularly lacking for many montane and polar species, and island endemics ( La Sorte and Jetz, 2010 ; Post et al., 2019 ; Veron et al., 2019 ). Further, temporal adaptations will cause increasing phenological mismatches between currently interacting species ( Kharouba et al., 2018 ), hastening the breakdown of biotic communities and amplifying extinction risks. All major ecosystems will undergo major transformation ( Nolan et al., 2018 ), though tropical biomes such as humid forests and coral reefs are particularly threatened ( Trisos et al., 2020 ). While these direct impacts are likely to affect most if not all species and ecosystems, they will also serve as a multiplier of existing threats (such as habitat change and overharvesting), as human societies take actions to mitigate and adapt to changes already underway ( Watson, 2014 ). Thus, climate change is expected to drive rapid increases in species extinction risk ( Urban, 2015 ; Román-Palacios and Wiens, 2020 ).
While conservation was failing to arrest biodiversity loss even before climate impacts started to become apparent, it is now clear that climate change will be so severe as to threaten the survival of entire biomes and condemn many species to extinction regardless of any future conservation efforts. The principal conservation objective of preventing extinctions is built on the assumption that all species can be saved from extinction, yet climate change renders this largely obsolete. Given that existing conservation efforts, which were already failing, will be inadequate in the face of climate impacts, the continuation of the current conservation paradigm is not an option and the field must rapidly adapt its objectives and strategies. But while the climate emergency necessitates an evolution in conservation, it also presents an opportunity to mainstream conservation by reframing the field as critical to the maintenance of human civilisation, as well as other species.
From Biodiversity Conservation to Survival Ecology
According to the lead authors of the Intergovernmental Science-Policy Platform on Biodiversity and Ecosystem Services (IPBES), preventing catastrophic climate change and reversing other drivers of biodiversity loss will require “transformative change, namely a fundamental, system-wide reorganisation across technological, economic, and social factors, making sustainability the norm rather than the altruistic exception” ( Díaz et al., 2019 ). However, conservation largely continues to portray and market itself as an “altruistic exception” by framing the field as the endeavour to prevent extinctions of other species. This is problematic because, while many decision-makers may agree that conserving species is a noble goal, few behave as if it is essential. As a result, conservation remains an economically and socially marginal activity; for example, protected areas are our principal conservation strategy but global protected area spending is estimated at US$ 24.3 billion/year ( Waldron et al., 2020 ), the size of the global beard grooming industry ( Thomas and Deshmukh, 2019 ). Simply put, conservation is not a priority for our societies, and neither the public nor political leaders understand the gravity of the threat biodiversity loss poses for humanity ( Bradshaw et al., 2021 ).
Conservation should be ultimately about much more than preventing the extinction of threatened species, because the promotion of complex, well-functioning and resilient ecosystems ensures the generation of ecosystem services that underpin human wellbeing in the present ( Díaz et al., 2019 ), and is a key strategy for both mitigating and adapting to ( Malhi et al., 2020 ) the catastrophic climate change that threatens the survival of human civilisation. If we are to persuade society to take conservation seriously, we must stop framing it as the altruistic quest to save other species from extinction, and instead present it as the selfish, pragmatic goal of sustaining the conditions for human civilisation and other life on Earth. Given that climate change renders the objective of preventing all species extinctions impossible, the goal of conservation should be to retain, into the future, an Earth system in which life (including human life) can flourish. In other words, we must dynamically maintain, restore and create ecosystems to allow the biosphere to evolve, adapt and change such that it maintains itself in all its complexity during a time of rapid biotic and abiotic change. We must move from biodiversity conservation to survival ecology.
While the survival ecology framing has some echoes of other approaches which centre conservation around explicitly anthropocentric concerns, including ecosystem services (“ES,” Millennium Ecosystem Assessment, 2005 ), nature's contributions to people ( “NCP,” Díaz et al., 2018 ), and natural climate solutions (“NCS,” Griscom et al., 2017 ), it differs fundamentally in three respects. Firstly, while the ES, NCP and NCS frameworks focus on the specific components of biodiversity that generate desired services, survival ecology instead focuses on the conditions that allow them (or any other, less service-generating components of biodiversity) to thrive, and strives for resilient and adaptable ecosystems that benefit all species, including humans. Rather than focusing on elements of the Earth system that are of value to people, survival ecology requires managing the system as a whole. Secondly, survival ecology recognises that a focus on present values (which is the predominant focus of ES and NCP) is inappropriate in a time of rapid global change, and is explicitly forward-looking. Thirdly, existing frameworks do not address the question of how conservation itself needs to change, while survival ecology recognises the need for new philosophies and approaches (which we discuss in the following sections).
Some authors have argued that utilitarian rationales for conservation have partly contributed to to the ecological crisis, and thus that anthropocentric framings carry risks ( Taylor et al., 2020 ). However, the ecocentric arguments central to conservation have been equally unsucessful in persuading society of the importance of the living world. In this regard, nothing conservationists have tried so far has worked, but it would be foolish to stop trying new approaches in an emergency context. Moreover, while survival ecology is somewhat anthropocentric in framing, it is neither anthropocentric nor ecocentric in practise because, as the IPBES lead authors state, “maintaining a life-sustaining and life-fulfilling planet for humans and other species are […] one and the same challenge” ( Díaz et al., 2019 ). Nevertheless, survival ecology is more than just a reframing of conservation for marketing purposes or asking people to ascribe monetary value to biodiversity. It also requires a fundamental shift in conservation philosophies and approaches, and in particular a re-examination of our atttutide to biotic change ( Figure 1 ).
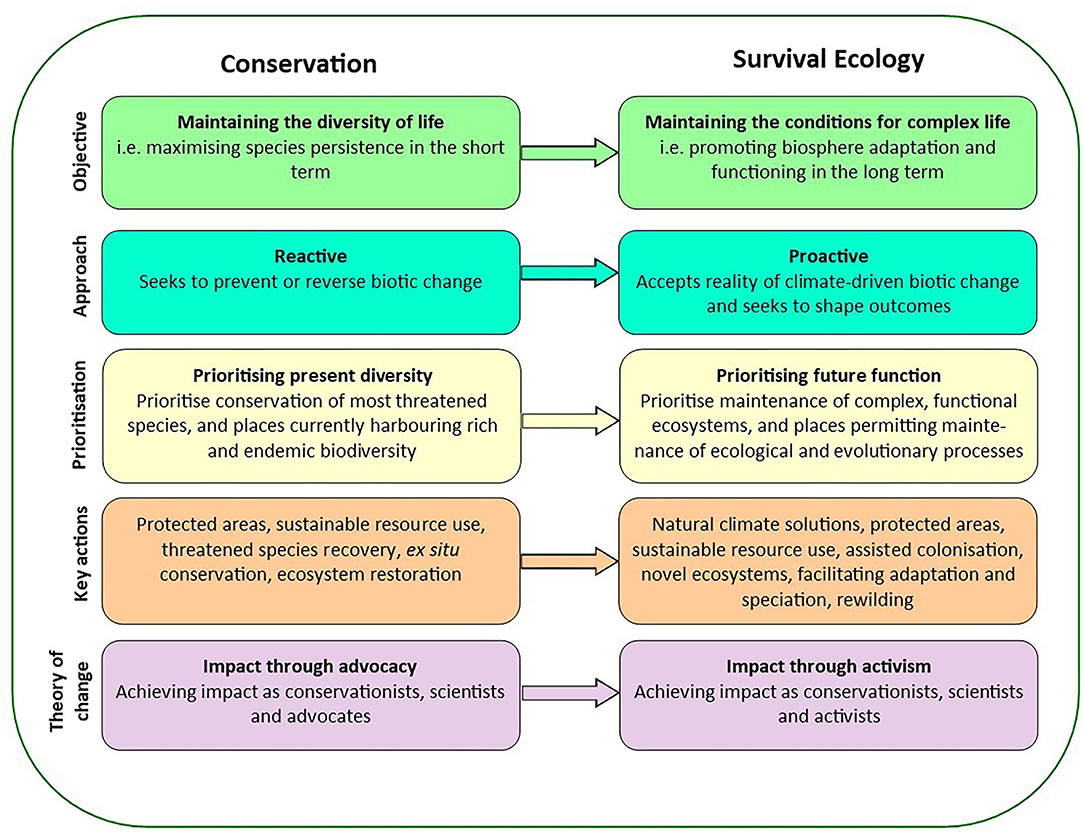
Figure 1 . The transition from conservation to survival ecology, which requires a shift in objectives and approach, as well as prioritisation, key actions, and theory of change.
From Reactive to Proactive
Conservation is a dynamic, evolving field and some efforts have been made to adapt it to the rapidly changing climate. These include the NCS approach to maximise conservation's contribution to climate mitigation, and efforts to enhance the resilience of approaches such as protected areas. However, rapid adaptation to the climate emergency remains hampered the field's prevailing attitude to change. Conservationists have generally sought either to prevent change (by combatting anthropogenic threats to maintain species and ecosystems as they are now), or to reverse it (by restoring species populations and ecosystems to some desired past state). However, given the inevitability of widespread climate-driven abiotic change, including considerable extinctions, we must cease looking to the past for our benchmarks, and instead think to the future ( Thomas, 2020 ). Conservation time and resources spent on trying to maintain the world as it currently is, or recently was, risk being wasted because that world will soon no longer exist, if indeed it still does. While it is of course desirable to maintain as much of current biodiversity as possible, and a comprehension of current and past ecosystems may inform future actions, the goal of maintaining ecosystems as they are, or restoring them to how they used to be, is simply not possible in a rapidly changing climate. Failure to acknowledge that, and refocus conservation objectives in response, risks wasting conservation time and resources on quixotic quests. Conversely, letting go of the past must not lead to a laissez faire attitude to future change. We need to plan proactively, by defining the future we want, researching what is needed to get there, and starting to actively manage both species and ecological and evolutionary processes to influence the outcomes of unavoidable environmental change. We must stop working to conserve a world that will no longer exist, and start trying to shape the one that will.
One key way in which this proactive approach will manifest itself is in our attitudes to the distribution of life on Earth, and our concepts of native and non-native species. The introduction of invasive non-native species has been a major driver of extinctions and other biodiversity impacts ( Pyšek et al., 2020 ), so the deliberate translocation of species is risky and controversial. However, given the mass of extinctions projected this century as a result of species' inabilities to track or adapt to climate change ( Román-Palacios and Wiens, 2020 ), assisted colonisation on a massive scale ( Butt et al., 2020 ) will likely be required within decades. This may even require the active establishment of entire ecosystems in novel places—if coral reefs and tropical humid forests will no longer occur in their current locations, should we not make efforts to ensure they exist somewhere? These activities could also promote evolution and speciation in the new environments, potentially leading to the maintenance of biological complexity ( Hendry et al., 2017 ). Given that this will necessarily entail the creation of novel ecosystems, a regulatory framework and enhanced research agenda will be required.
Of course, for many ecosystems we lack the ecological knowledge to be able to intervene in this way and be confident of the outcomes, though restoration ecology can give pointers as to how to assemble new ecosystems, and evidence for novel ecosystems shows that new combinations of species can assemble communities successfully ( Kennedy et al., 2018 ). While such “experimentation” does represent a leap in the dark to some extent, when one is in a burning building the only choice is to leap. Climate change is already a huge experiment with the future of life on Earth, and no non-radical choices remain. The only options are to continue as normal with conservation mindsets developed in the climatically-stable world we have left behind, or to adapt our approaches and develop solutions explicitly designed to overcome the problems at hand. Nevertheless, even such radical changes in conservation approaches will be insufficient unless we also reconsider how conservation seeks to influence political and economic decision-making, and wider society.
From Advocates to Activists
Conservation science largely operates according to an unspoken “theory of change” (ToC) that if we generate scientific information about biodiversity loss and its implications, and develop solutions, society's leaders will use that information to make wise policy decisions, and fund the implementation of those solutions. However, this ToC is based on false assumptions, and conservation is failing (just as climate change is occurring) because scientists have not adequately considered how social and political change occurs in the real world ( Gardner et al., 2021 ). Conservation science alone cannot determine governmental policy-making and funding decisions because these are additionally influenced by the lobbying, donations and public relations campaigns of billion-dollar industries including agrochemicals, industrial meat production, fisheries, logging, plastics, mining, construction, and so on. Since the science and practise of conservation have not and cannot be enough to bring about the required transformative changes in our economic systems, survival ecology (or any appropriate response to an emergency context) requires that we should additionally engage in efforts with a higher chance of success.
Direct activism such as non-violent civil disobedience has historically been effective in bringing about political change ( Chenoweth and Stephan, 2012 ), and played an important role in the successful struggles for universal suffrage in the UK, civil rights in the USA, and independence from colonialism in many countries. Since 2018 it has also succeeded in raising climate change up the media, public and political agenda, through the actions of movements such as Fridays for Future, Extinction Rebellion and others. For example, numerous countries have declared a climate emergency, and in 2019 the environment grew to become the third largest concern for British voters ( Gardner and Wordley, 2019 ). However, the increase in public, media and political concern has been heavily weighted towards climate, and the parallel issue of biodiversity loss has been overshadowed ( Gardner et al., 2020 ). Similar efforts are required for the living world, and these may be lent important credibility and authority should scientists participate publicly as scientists, rather than simply as citizens ( Gardner et al., 2021 ).
Such is the primacy of the science-to-policy change ToC in conservation that some suggest conservation scientists should refrain even from advocacy for fear of reducing their scientific legitimacy or authority (see e.g., Lackey, 2007 ; Chan, 2008 ). However, conservation science is an explicitly normative and value-laden discipline: it sees biodiversity as good, and strives to maintain it ( Noss, 2007 ). To achieve this objective, conservationists have a long history of extending beyond research to engage in both conservation practise and advocacy. Indeed, it is common for conservation scientists to work for, collaborate with, or receive funding from non-governmental and campaigning organisations adopting the explicit political position that biodiversity should be prioritised in decision-making, or advocating for one species (or site, or conservation method) over others. Values and advocacy are thus integral to conservation, and to claim scientists and the science they do are value-neutral risks undermining the public's trust and acceptance of that science ( Oreskes, 2019 ). However, while conservation scientists have long engaged in advocacy, neither that nor our research and practise have been sufficient to slow the destruction of nature appreciably ( Secretariat of the Convention on Biological Diversity, 2020 ; Bradshaw et al., 2021 ) and they will prove increasingly inadequate as the climate emergency deepens. In this emergency, we must think beyond approaches that have proved inadequate to date. If we are to move conservation issues to the mainstream of public and political thought, and trigger the transformations required to maintain most life on Earth, we will need to become more influential. If conservation was content with advocacy, the twin climate and ecological emergencies require survival ecologists to go one step further, and become activists.
Discussion and Conclusions
Conservation, we believe, is in need of radical reform because it is unable to achieve its goals in the present, and will be increasingly unable to do so in a rapidly changing future. By largely focusing on and marketing itself as the conservation of other species it has constrained itself to being only peripherally relevant to human society, and in seeking to prevent biotic change it has burdened itself with a goal rendered impossible by climate change. By reframing goals around the maintenance of an Earth system in which both humans and other species can survive (and hopefully thrive), we believe that the field will be better able to mainstream itself into political and economic decision-making, and avoid wasting effort and resources on endeavours that will ultimately prove futile.
Some of our suggestions may be controversial, not least the notion that preventing species extinctions is no longer necessarily an appropriate goal. However, it has long been recognised that not all species can be saved, and discussions over conservation triage were underway long before the extent of climate impacts on biodiversity became apparent ( Bottrill et al., 2008 ). The ES, NCP, and NCS frameworks imply that species not contributing to the delivery of particular services should be deprioritised, though survival ecology makes no such value judgements and recognises the role of all species in maintaining complex and resilient ecosystems. It does, however, acknowledge the inevitability of extinctions, and emphasises the maintenance of an Earth system in which species can adapt and new species can evolve, to counter that loss.
We seek only to stimulate an urgently-needed debate on how to conserve biodiversity in a rapidly-changing world, and it is beyond this essay to propose any plan of implementation. Indeed survival ecology cannot necessarily even be “implemented,” for it is a way of thinking about conservation rather than a plan or toolbox. Many of the tools and techniques developed by conservationists will clearly remain essential to the survival ecology agenda. For example, we will still need to manage protected areas, restore ecosystems, and empower local communities to manage natural resources sustainably. However, these and other traditional approaches will never be sufficient while they are implemented in pursuit of an objective that is both impossible to achieve and considered trivial by society. In the climate emergency, we can no longer conserve all biodiversity. Instead we must aim to maintain the planetary conditions that will allow both humans and other species to thrive.
Author Contributions
CG conceived the paper. CG and JB wrote the paper. Both authors contributed to the article and approved the submitted version.
Conflict of Interest
The authors declare that the research was conducted in the absence of any commercial or financial relationships that could be construed as a potential conflict of interest.
Publisher's Note
All claims expressed in this article are solely those of the authors and do not necessarily represent those of their affiliated organizations, or those of the publisher, the editors and the reviewers. Any product that may be evaluated in this article, or claim that may be made by its manufacturer, is not guaranteed or endorsed by the publisher.
Acknowledgments
CG thanks Sophus zu Ermgassen for stimulating discussion of some of the ideas in this paper.
Bolam, F. C., Mair, L., Angelico, M., Brooks, T. M., Burgman, M., Hermes, C., et al. (2020). How many bird and mammal extinctions has recent conservation action prevented? Conserv. Lett. 14:e12762. doi: 10.1111/conl.12762
CrossRef Full Text | Google Scholar
Bottrill, M. C., Joseph, L. N., Cawardine, J., Bode, M., Cook, C., Game, E. T., et al. (2008). Is conservation triage just smart decision making? Trends Ecol. Evol . 23, 649–654. doi: 10.1016/j.tree.2008.07.007
PubMed Abstract | CrossRef Full Text | Google Scholar
Bradshaw, C. J. A., Ehrlich, P. R., Beattie, A., Ceballos, G., Crist, E., Diamond, J., et al. (2021). Underestimating the challenges of avoiding a ghastly future. Front. Conserv. Sci. 1:615419. doi: 10.3389/fcosc.2020.615419
Butt, N., Chauvenet, A. L. M., Adams, V. M., Beger, M., Gallagher, R. V., Shanahan, D. F., et al. (2020). Importance of species translocations under rapid climate change. Conserv. Biol. 35, 775–783. doi: 10.1111/cobi.13643
Chan, K. M. A. (2008). Value and advocacy in conservation biology: crisis discipline or discipline in crisis? Conserv. Biol . 22, 1–3. doi: 10.1111/j.1523-1739.2007.00869.x
Chenoweth, E., and Stephan, M. J. (2012). Why Civil Resistance Works: The Strategic Logic of Nonviolent Conflict . New York, NY: Columbia University Press.
Google Scholar
Díaz, S., Pascual, U., Stenseke, M., Martín-López, B., Watson, R. T., Molnár, Z., et al. (2018). Assessing nature's contributions to people. Science 359, 270–272. doi: 10.1126/science.aap8826
Díaz, S., Settele, J., Brondízio, E. S., Ngo, H. T., Agard, J., Arneth, A., et al. (2019). Pervasive human-driven decline of life on Earth points to need for transformative change. Science 366:eaax3100. doi: 10.1126/science.aax3100
Gardner, C. J., Struebig, M. J., and Davies, Z. G. (2020). Conservation must capitalise on climate's moment. Nat. Commun. 11:109. doi: 10.1038/s41467-019-13964-y
Gardner, C. J., Thierry, A., Rowlandson, W., and Steinberger, J. K. (2021). From publications to public actions: the role of universities in facilitating academic advocacy and activism in the climate and ecological emergency. Front. Sustain . 2:679019. doi: 10.3389/frsus.2021.679019
Gardner, C. J., and Wordley, C. F. R. (2019). Scientists must act on our own warnings to humanity. Nat. Ecol. Evol. 3, 1271–1272. doi: 10.1038/s41559-019-0979-y
Griscom, B. W., Adams, J., Ellis, P. W., Houghton, R. A., Lomax, G., Miteva, D. A., et al. (2017). Natural climate solutions. Proc. Natl. Acad. Sci. U.S.A. 114, 11645–11650. doi: 10.1073/pnas.1710465114
Hendry, A. P., Gotanda, K. M., and Svensson, E. I. (2017). Human influences on evolution, and the ecological and societal consequences. Phil. Trans. R. Soc. B 372:20160028. doi: 10.1098/rstb.2016.0028
Kennedy, P. L., Fontaine, J. B., Hobbs, R. J., Johnson, T. N., Boyle, R., and Lueders, A. S. (2018). Do novel ecosystems provide habitat for wildlife? Revisiting the physiognomy vs. floristics debate. Ecosphere 9:e02172. doi: 10.1002/ecs2.2172
Kharouba, H. M., Ehrlén, J., Gelman, A., Bolmgren, K., Allen, J. M., Travers, S. E., et al. (2018). Global shifts in the phenological synchrony of species interactions over recent decades. Proc. Natl. Acad. Sci. U.S.A. 115, 5211–5216. doi: 10.1073/pnas.1714511115
La Sorte, F. A., and Jetz, W. (2010). Projected range contractions of montane biodiversity under global warming. Proc. R Soc. B 277, 3401–3410. doi: 10.1098/rspb.2010.0612
Lackey, R. T. (2007). Science, scientists, and policy advocacy. Conserv. Biol. 21, 12–17. doi: 10.1111/j.1523-1739.2006.00639.x
Malhi, Y., Franklin, J., Seddon, N., Solan, M., Turner, M. G., Field, C. B., et al. (2020). Climate change and ecosystems: threats, opportunities and solutions. Phil. Trans. R Soc. B 375:20190104. doi: 10.1098/rstb.2019.0104
Millennium Ecosystem Assessment (2005). Ecosystems and Human Well-Being: Synthesis . Washington, DC: Island Press.
Nolan, C., Overpeck, J. T., Allen, J. R. M., Anderson, P. M., Betancourt, J. L., Binney, H. A., et al. (2018). Past and future global transformation of terrestrial ecosystems under climate change. Science 361, 920–923. doi: 10.1126/science.aan5360
Noss, R. F. (2007) Values are a good thing in conservation biology. Conserv. Biol. 21:18–20.
Oreskes, N. (2019). Why Trust Science? Princeton, NJ: Princeton University Press.
Post, E., Alley, R. B., Christensen, T. R., Macias-Fauria, M., Forbes, B. C., Gooseff, M. N., et al. (2019). The polar regions in a 2°C warmer world. Sci. Adv. 5:eaaw9883. doi: 10.1126/sciadv.aaw9883
Pyšek, P., Hulme, P. E., Simberloff, D., Bacher, S., Blackburn, T. M., Carlton, J. T., et al. (2020). Scientists' warning on invasive alien species. Biol. Rev. 96, 1511–1534. doi: 10.1111/brv.12627
Radchuck, V., Reed, T., Teplitsky, C., van de Pol, M., Charmantier, A., Hassall, C., et al. (2019). Adaptive responses of animals to climate change are most likely insufficient. Nat. Commun. 10:3109. doi: 10.1038/s41467-019-10924-4
Richards, C. E., Lupton, R. C., and Allwood, J. M. (2021). Re-framing the threat of global warming: an empirical causal loop diagram of climate change, food insecurity and societal collapse. Clim Change 164:49. doi: 10.1007/s10584-021-02957-w
Ripple, W. J., Wolf, C., Newsome, T. M., Gregg, J. W., Lenton, T. M., Palomo, I., et al. (2021). World scientists' warning of a climate emergency 2021. BioScience 71, 894–898. doi: 10.1093/biosci/biab079
Román-Palacios, C., and Wiens, J. J. (2020). Recent responses to climate change reveal the drivers of species extinction and survival. Proc. Natl. Acad. Sci. U.S.A. 117, 4211–4217. doi: 10.1073/pnas.1913007117
Rounsevell, M. D. A., Harfoot, M., Harrison, P. A., Newbold, T., Gregory, R. D., and Mace, G. D. (2020). A biodiversity target based on species extinctions. Science 368, 1193–1195. doi: 10.1126/science.aba6592
Sandbrook, C., Fisher, J. A., Holmes, G., Luque-Lora, R., and Keane, A. (2019). The global conservation movement is diverse but not divided. Nat. Sustain. 2, 316–323. doi: 10.1038/s41893-019-0267-5
Secretariat of the Convention on Biological Diversity (2020). Global Biodiversity Outlook 5 . Montreal, QC: Secretariat of the Convention on Biological Diversity.
Taylor, B., Chapron, G., Kopnina, H., Orlikowska, E., Gray, J., and Piccolo, J. J. (2020). The need for ecocentrism in biodiversity conservation. Conserv. Biol. 34, 1089–1096. doi: 10.1111/cobi.13541
Thomas, A., and Deshmukh, R. (2019). Beard Grooming Market: Global Opportunity Analysis and Industry Forecast, 2019–2026. Pune: Allied Market Research . Available online at: https://www.alliedmarketresearch.com/beard-grooming-market (accessed August 6, 2021).
Thomas, C. D. (2020). The development of Anthropocene biotas. Philos. Trans. R Soc. B 375:20190113. doi: 10.1098/rstb.2019.0113
Trisos, C. H., Merow, C., and Pigot, A. L. (2020). The projected timing of abrupt ecological disruption from climate change. Nature 580, 496–501. doi: 10.1038/s41586-020-2189-9
Urban, M. (2015). Accelerating extinction risk from climate change. Science 348, 571–573. doi: 10.1126/science.aaa4984
Veron, S., Mouchet, M., Govaerts, R., Haevermans, T., and Pellens, R. (2019). Vulnerability to climate change of islands worldwide and its impact on the tree of life. Sci. Rep. 9:14471. doi: 10.1038/s41598-019-51107-x
Waldron, A., Adams, V., Allan, J., Arnell, A., Asner, G., Atkinson, S., et al. (2020). Protecting 30% of the Planet for Nature: Costs, Benefits and Economic Implications . Washington, DC: Campaign for Nature. Available online at: https://www.conservation.cam.ac.uk/files/waldron_report_30_by_30_publish.pdf (accessed August 6, 2021).
Watson, J. E. M. (2014). Human responses to climate change will seriously impact biodiversity conservation: it's time we start planning for them. Conserv. Lett . 7, 1–2. doi: 10.1111/conl.12083
Keywords: biodiversity, climate, civil disobedience, collapse, conservation, extinction, social movement
Citation: Gardner CJ and Bullock JM (2021) In the Climate Emergency, Conservation Must Become Survival Ecology. Front. Conserv. Sci. 2:659912. doi: 10.3389/fcosc.2021.659912
Received: 28 January 2021; Accepted: 29 September 2021; Published: 28 October 2021.
Reviewed by:
Copyright © 2021 Gardner and Bullock. This is an open-access article distributed under the terms of the Creative Commons Attribution License (CC BY) . The use, distribution or reproduction in other forums is permitted, provided the original author(s) and the copyright owner(s) are credited and that the original publication in this journal is cited, in accordance with accepted academic practice. No use, distribution or reproduction is permitted which does not comply with these terms.
*Correspondence: Charlie J. Gardner, cjamgardner@yahoo.co.uk

An official website of the United States government
Here’s how you know
Official websites use .gov A .gov website belongs to an official government organization in the United States.
Secure .gov websites use HTTPS A lock ( Lock A locked padlock ) or https:// means you’ve safely connected to the .gov website. Share sensitive information only on official, secure websites.
JavaScript appears to be disabled on this computer. Please click here to see any active alerts .
Climate Change Impacts on Ecosystems
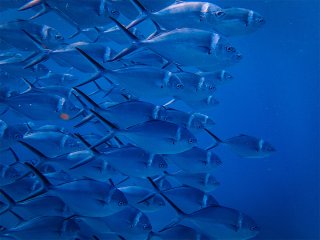
Ecosystems are communities of living things, including plants, animals, and microorganisms, that interact with each other and the physical world. 1 People depend on ecosystems for many benefits, such as food, water, clean air, building materials, and recreation. Ecosystems can be big, like the one that surrounds Yellowstone National Park, 2 or as small as a single fallen tree. They can also overlap with one another or be part of larger ecosystems. These connections between ecosystems also make them dependent on one another, and not simply dependent on the organisms within them.
Climate change affects ecosystems in many ways. Climate controls how plants grow, how animals behave, which organisms thrive, and how they all interact with the physical environment. 3 , 4 As habitats experience different temperatures, precipitation patterns, and other changes, the organisms that make up ecosystems feel the effects. All U.S. regions are experiencing the impacts of climate change but impacts vary by area and ecosystem. 5
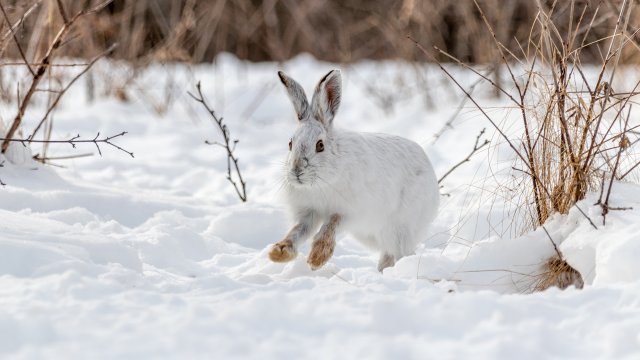
Impacts on populations' ability to survive. The white coat of the snowshoe hare makes it less visible to predators in the winter. But earlier snowmelts are making the hare stand out more against the brown forest floor, increasing its vulnerability.
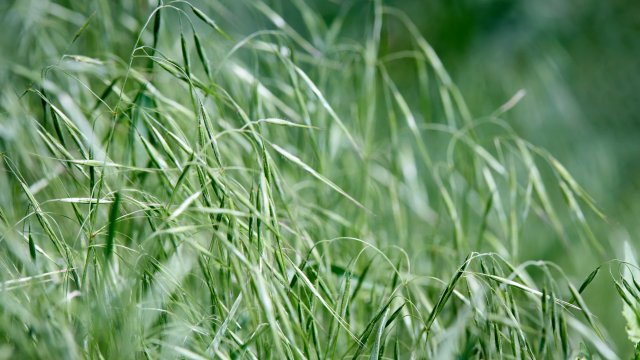
Increased spread of invasive species. Cheatgrass is an invasive species that threatens ranches in the western United States. It degrades soil nutrients and can lead to a loss of biodiversity.
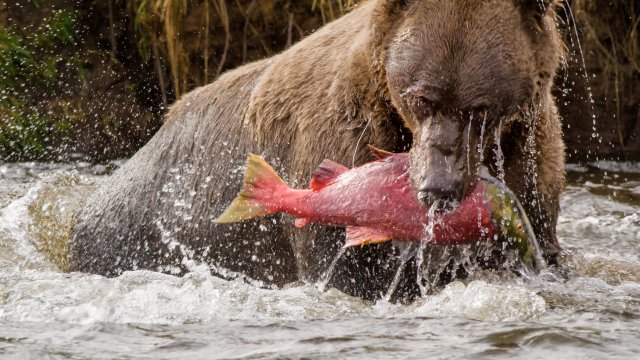
Changes in the timing of natural events. In Alaska, brown bears are switching their diet from salmon to elderberries as the berries ripen earlier due to a warming climate. 30 When bears eat less salmon, it also affects other animals in the food web, like birds that feed on the salmon carcasses bears leave behind. 31
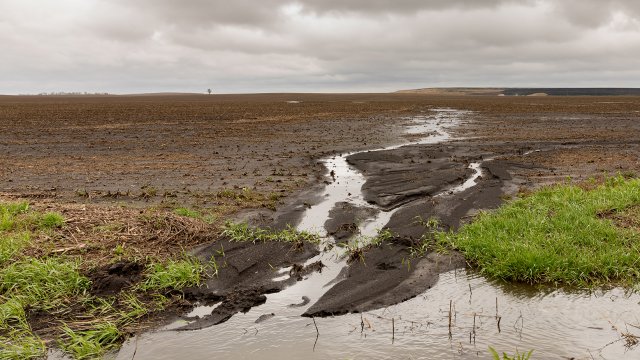
Reduced ecosystem services: erosion control. Salt marsh grasses help hold soil in place. In some areas, a change to the species balance, possibly due to changing temperatures, has allowed a grass-eating marsh crab to dominate, resulting in less grass to control erosion. 32
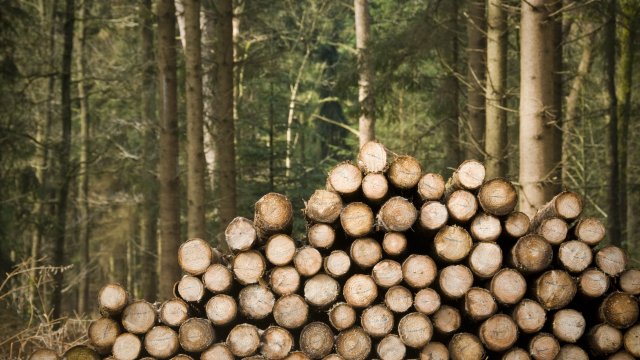
Reduced ecosystem services: lumber. Ecosystems provide Americans with needed materials such as wood. Changing precipitation patterns can influence the frequency and severity of wildfires, threatening this ecosystem service and important economic sector.
People are taking many actions to help ecosystems adapt to climate change impacts or minimize the effects. For example, federal agencies that manage the nation’s natural resources are now considering climate change in polices and planning. At the local level, many groups are preserving habitats and restoring ecosystems that have been damaged or disturbed in the past.
Explore the sections on this page to learn more about climate impacts on ecosystems:
Top Climate Impacts on Ecosystems
- Ecosystems and the Economy
Environmental Justice and Equity
What we can do, related resources, ecosystem services.
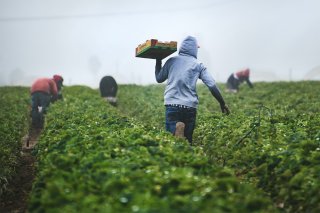
Ecosystems provide many benefits to people. These are called ecosystem services . Ecosystem services include goods from plants and animals, such as the food and wood provided by trees. They also include parts of the physical world that keep the planet safe and healthy. For example, clean lakes and streams are considered ecosystem services because we use them for drinking water and recreation.
Climate change affects ecosystems at multiple levels, from the populations that make up ecosystems to the services they provide to communities, economies, and people. Four key impacts are described in this section.
1. Changes in Species and Populations
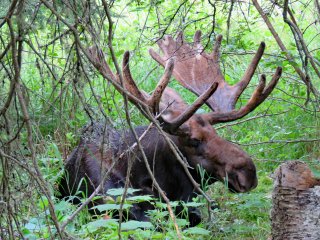
As the climate changes, some species will adapt by changing their behavior, physical characteristics, or how their bodies function. Others will not be able to adapt. As a result, climate change could lead to expansions, reductions, or extinctions of some populations. These changes, in turn, can affect the overall biodiversity of a region.
Plants and animals may also change the geographic range they inhabit in response to changing climatic conditions. Changing temperature and water conditions have already altered the ranges of many plants and animals. 6 As temperatures have warmed in the United States, some land animals have moved to the (typically cooler) north by an average of 3.8 miles per decade. 7 Some marine species have also shifted north by more than 17 miles per decade. 8
2. Changes in the Timing of Natural Events and Cycles
Many plants and animals rely on cues in nature, including temperature and water conditions, to trigger certain stages of their life cycles. As the climate changes, these cues can change at different rates, or potentially not all. As a result, species that depend on one another at certain times of the year may no longer be in sync.
For example, plankton are an important food source for young fish, but they tend to react more quickly to changes in temperature than the fish. This means the plankton might not be as available when growing fish need it most. Also, if a bird migrates at the same time each year, it could reach its destination to find that, due to shifting temperatures, its main food source grew too early and is no longer available. 10
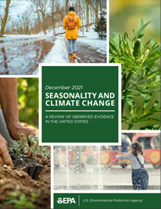
3. Changes in Ecosystem Interactions
Climate change is also changing the way species and populations interact with the environment and one another. These impacts can be felt throughout an entire ecosystem.
For example, climate change is increasing the spread of invasive species in some areas. 11 An invasive species is one that is not native to an area. Invasive species can outcompete native plants and animals, bring in new diseases, and cause other problems. These changes can create significant environmental and economic harm.
As ocean water warms, invasive fish species, such as the tropical lionfish , are expected to move north along the Atlantic coast, threatening native species. 12 This can also hurt humans, as lionfish are venomous and can sting people.
Climate change can also affect food webs. A food web is the whole set of feeding relationships among different organisms in an ecosystem. At the bottom of a food web are organisms like plants and plankton. Other animals, higher in the web, rely on them as food sources. Climate impacts on any part of a food web can affect the whole system, and even other ecosystems altogether. From the example above, if young fish cannot find enough food in the estuaries, their predators in the ocean could feel the effects as well.
4. Altered or Reduced Ecosystem Services
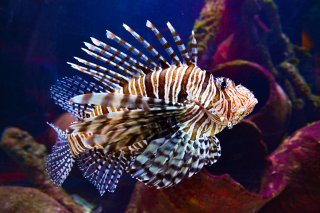
Climate change is affecting some of the critical services that ecosystems provide to society. 13 For example, ecosystems provide a bounty of food to people. Climate changes, like drought and heat, could affect the availability and quality of some foods , as well as farmers’ ability to grow certain crops. 14
Climate change can also affect ecosystem services such as carbon capture and storage. Forest ecosystems play a critical role in the carbon cycle, helping to absorb carbon dioxide from the atmosphere and store it in roots, soil, and the forest floor. 15 But climate-driven increases in wildfires, flooding, pests, and diseases can limit the ability of an ecosystem to provide this important service. 16
For more specific examples of climate change impacts in your region, please see the National Climate Assessment .
Ecosystems and the Economy
Ecosystems and their benefits—often referred to as ecosystem services—support key aspects of human existence. As a result, they are the foundation to large parts of the economy. Globally, ecosystem services are worth an estimated $125 to $145 trillion per year. 17
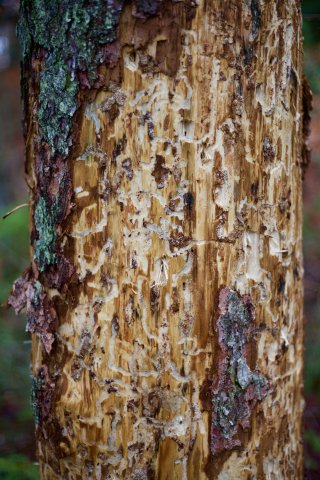
Climate change impacts affect the livelihoods of millions of Americans, including fishers, loggers, ranchers, and farmers. For example, the stress caused by rising water temperatures and ocean acidification could cost the shellfish industry hundreds of millions of dollars. 18 Shifting ranges for certain fish mean that fishers may need to travel farther to catch them or purchase new equipment to reach those areas. 19 These shifts are projected to result in losses of hundreds of millions of dollars each year by the year 2100. 20 U.S. agriculture will also experience economic impacts, though they could be both negative and positive. Climate change threatens to bring increased droughts, pests, and extreme weather events, which would take an economic toll. 21 However, earlier springs and milder winter weather can prolong growing seasons in many regions, increasing crop yields and revenues. 22
Climate change also affects the tourism and recreation industries. Harmful algal blooms already cost the country nearly $1 billion each year from the loss of recreational fishing and boating revenue. 24 Damage to coral reefs due to climate change is projected to result in $140 billion in total lost revenues to the U.S. recreation industry by 2100. 25 Communities that depend on tourism will feel these economic impacts the most.
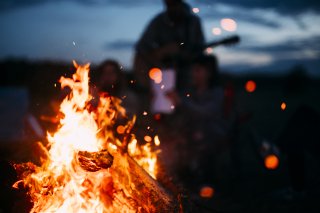
Some communities depend on ecosystems and their services more than others, including some Indigenous and rural communities. As ecosystems change, some groups also may be able to adapt better than others due to geographic, social, or economic factors.
Indigenous communities have a unique relationship with the environment. Climate change impacts on ecosystems can harm Indigenous peoples’ ability to preserve and sustain their cultural heritage. 26 , 27 Shifting animal ranges can disrupt Indigenous peoples’ ability to hunt and fish as the animals move beyond their tribal lands. 28
Many rural communities depend on ecosystem-related livelihoods, including farming, fishing, and raising livestock. People in these areas are more likely to see economic impacts when faced with ecosystem challenges, such as invasive species and decreased soil quality. 29
We can limit climate change’s impacts on ecosystems in several ways, including the following:
- Prevent the spread of aquatic invasive species. Inspect and wash your boat before moving it to a new body of water to remove “hitchhiking” plants and animals , like zebra and quagga mussels.
- Share information. Engage in citizen science efforts to provide accurate, up-to-date information about climate change in the United States.
- Report invasive species. Reporting invasive species to the proper authorities can help them manage plants and animals that threaten native populations.
- Treat ecosystems responsibly. Practice management techniques that improve ecosystems’ health and help them be more resilient to a changing climate. For example, plant native vegetation and conserve water. In arid places planting native or drought resistant species conserves water.
- Conserve lands and grow prudently. Development can break up lands into smaller segments that can isolate species, making it harder for them to migrate or adapt to climate change. Smart conservation planning can help preserve lands and help populations thrive.
Related Climate Indicators
Learn more about some of the key indicators of climate change related to this sector from EPA’s Climate Change Indicators in the United States :
- Ecosystems Summary
- U.S. and Global Temperature
- Leaf and Bloom Dates
- Length of Growing Season
- Weather and Climate Summary
- Fifth National Climate Assessment, Chapter 8: “Ecosystems, Ecosystem Services, and Biodiversity.”
- U.S. Climate Resilience Toolkit: Ecosystems . Provides information on how ecosystems affect air and water.
- Ecosystem Services . Provides information and decision support tools around how to manage ecosystem services.
- U.S. Department of Agriculture: Climate Change Effects on Forests and Grasslands . Provides an interactive learning module explaining the main effects of climate changes on two important ecosystems.
- U.S. Geological Survey (USGS): Ecosystems . Presents the latest USGS-generated science on ways to sustainably manage ecosystems.
- U.S. Fish and Wildlife Service: Climate Change . Summarizes climate change impacts, adaptation strategies, and mitigation strategies with a focus on ecosystems.
- EPA’s Report on Seasonality and Climate Change . Summarizes the state of the science on observed changes relating to seasonality in the United States.
1 Lipton, D., et al. (2018). Ch. 7: Ecosystems, ecosystem services, and biodiversity . In: Impacts, risks, and adaptation in the United States: Fourth national climate assessment, volume II . U.S. Global Change Research Program, Washington, DC, p. 270.
2 U.S. Department of the Interior, National Park Service. (2022). Greater Yellowstone ecosystem. Retrieved 01/29/2022.
3 Lipton, D., et al. (2018). Ch. 7: Ecosystems, ecosystem services, and biodiversity . In: Impacts, risks, and adaptation in the United States: Fourth national climate assessment, volume II . U.S. Global Change Research Program, Washington, DC, p. 276.
4 Lipton, D., et al. (2018). Ch. 7: Ecosystems, ecosystem services, and biodiversity . In: Impacts, risks, and adaptation in the United States: Fourth national climate assessment, volume II . U.S. Global Change Research Program, Washington, DC, p. 273.
5 Lipton, D., et al. (2018). Ch. 7: Ecosystems, ecosystem services, and biodiversity . In: Impacts, risks, and adaptation in the United States: Fourth national climate assessment, volume II . U.S. Global Change Research Program, Washington, DC, p. 279.
6 Lipton, D., et al. (2018). Ch. 7: Ecosystems, ecosystem services, and biodiversity . In: Impacts, risks, and adaptation in the United States: Fourth national climate assessment, volume II . U.S. Global Change Research Program, Washington, DC, p. 291.
7 Lipton, D., et al. (2018). Ch. 7: Ecosystems, ecosystem services, and biodiversity . In: Impacts, risks, and adaptation in the United States: Fourth national climate assessment, volume II . U.S. Global Change Research Program, Washington, DC, p. 281.
8 Lipton, D., et al. (2018). Ch. 7: Ecosystems, ecosystem services, and biodiversity . In: Impacts, risks, and adaptation in the United States: Fourth national climate assessment, volume II . U.S. Global Change Research Program, Washington, DC, p. 281.
9 Lipton, D., et al. (2018). Ch. 7: Ecosystems, ecosystem services, and biodiversity . In: Impacts, risks, and adaptation in the United States: Fourth national climate assessment, volume II . U.S. Global Change Research Program, Washington, DC, p. 291.
10 Lipton, D., et al. (2018). Ch. 7: Ecosystems, ecosystem services, and biodiversity . In: Impacts, risks, and adaptation in the United States: Fourth national climate assessment, volume II . U.S. Global Change Research Program, Washington, DC, p. 283.
11 Lipton, D., et al. (2018). Ch. 7: Ecosystems, ecosystem services, and biodiversity . In: Impacts, risks, and adaptation in the United States: Fourth national climate assessment, volume II . U.S. Global Change Research Program, Washington, DC, p. 282.
12 Lipton, D., et al. (2018). Ch. 7: Ecosystems, ecosystem services, and biodiversity . In: Impacts, risks, and adaptation in the United States: Fourth national climate assessment, volume II . U.S. Global Change Research Program, Washington, DC, p. 278.
13 Lipton, D., et al. (2018). Ch. 7: Ecosystems, ecosystem services, and biodiversity . In: Impacts, risks, and adaptation in the United States: Fourth national climate assessment, volume II . U.S. Global Change Research Program, Washington, DC, p. 284.
14 Lipton, D., et al. (2018). Ch. 7: Ecosystems, ecosystem services, and biodiversity . In: Impacts, risks, and adaptation in the United States: Fourth national climate assessment, volume II . U.S. Global Change Research Program, Washington, DC, p. 284.
15 Vose, J.M., et al. (2018). Ch. 6: Forests . In: Impacts, risks, and adaptation in the United States: Fourth national climate assessment, volume II . U.S. Global Change Research Program, Washington, DC, p. 244.
16 Vose, J.M., et al. (2018). Ch. 6: Forests . In: Impacts, risks, and adaptation in the United States: Fourth national climate assessment, volume II . U.S. Global Change Research Program, Washington, DC, p. 244.
17 U.S. Climate Resilience Toolkit. (2021). Ecosystems . Retrieved 03/18/2022.
18 Lipton, D., et al. (2018). Ch. 7: Ecosystems, ecosystem services, and biodiversity . In: Impacts, risks, and adaptation in the United States: Fourth national climate assessment, volume II . U.S. Global Change Research Program, Washington, DC, p. 284.
19 Lipton, D., et al. (2018). Ch. 7: Ecosystems, ecosystem services, and biodiversity . In: Impacts, risks, and adaptation in the United States: Fourth national climate assessment, volume II . U.S. Global Change Research Program, Washington, DC, p. 278.
20 Moore, C., et al. (2021). Estimating the economic impacts of climate change on 16 major U.S. fisheries . Climate Change Economics , 12(1).
21 Vose, J.M., et al. (2018). Ch. 6: Forests . In: Impacts, risks, and adaptation in the United States: Fourth national climate assessment, volume II . U.S. Global Change Research Program, Washington, DC, p. 234.
22 U.S. Environmental Protection Agency (EPA). (2016). Climate change indicators in the United States . Washington, DC, p. 10.
23 Lipton, D., et al. (2018). Ch. 7: Ecosystems, ecosystem services, and biodiversity . In: Impacts, risks, and adaptation in the United States: Fourth national climate assessment, volume II . U.S. Global Change Research Program, Washington, DC, p. 270.
24 EPA. The effects: Economy . Retrieved 03/18/2022.
25 EPA. (2017). Ch. 23: Coral reefs . In: Multi-model framework for quantitative sectoral impacts analysis: A technical report for the fourth national climate assessment . Washington, DC, p. 173.
26 Jantarasami, L.C., et al. (2018). Ch. 15: Tribes and Indigenous peoples . In: Impacts, risks, and adaptation in the United States: Fourth national climate assessment, volume II . U.S. Global Change Research Program, Washington, DC, p. 582.
27 Jantarasami, L.C., et al. (2018). Ch. 15: Tribes and Indigenous peoples . In: Impacts, risks, and adaptation in the United States: Fourth national climate assessment, volume II . U.S. Global Change Research Program, Washington, DC, p. 583.
28 Jantarasami, L.C., et al. (2018). Ch. 15: Tribes and Indigenous peoples . In: Impacts, risks, and adaptation in the United States: Fourth national climate assessment, volume II . U.S. Global Change Research Program, Washington, DC, p. 584.
29 Lipton, D., et al. (2018). Ch. 7: Ecosystems, ecosystem services, and biodiversity . In: Impacts, risks, and adaptation in the United States: Fourth national climate assessment, volume II . U.S. Global Change Research Program, Washington, DC, p. 278.
30 Lipton, D., et al. (2018). Ch. 7: Ecosystems, ecosystem services, and biodiversity . In: Impacts, risks, and adaptation in the United States: Fourth national climate assessment, volume II . U.S. Global Change Research Program, Washington, DC, p. 277.
31 Carlson, S.M. (2017). Synchronous timing of food sources triggers bears to switch from salmon to berries . Proceedings of the National Academy of Sciences of the United States of America, 114 (39): 10309–10311.
32 Lipton, D., et al. (2018). Ch. 7: Ecosystems, ecosystem services, and biodiversity . In: Impacts, risks, and adaptation in the United States: Fourth national climate assessment, volume II . U.S. Global Change Research Program, Washington, DC, p. 284.
- Climate Change Impacts Home
- Agriculture and Food Supply
- Air Quality
- Built Environment
- Freshwater Resources
- Ocean and Marine Resources
- Transportation
- Human Health
- State and Regional Impacts
- Climate Equity
When you choose to publish with PLOS, your research makes an impact. Make your work accessible to all, without restrictions, and accelerate scientific discovery with options like preprints and published peer review that make your work more Open.
- PLOS Biology
- PLOS Climate
- PLOS Complex Systems
- PLOS Computational Biology
- PLOS Digital Health
- PLOS Genetics
- PLOS Global Public Health
- PLOS Medicine
- PLOS Mental Health
- PLOS Neglected Tropical Diseases
- PLOS Pathogens
- PLOS Sustainability and Transformation
- PLOS Collections
- Collections Home
- About Collections
- Browse Collections
- Calls for Papers
Ecological Impacts of Climate Change
The ecological impacts of climate change are broad and diverse, altering species' range limits, plant phenology and growth, carbon and nutrient cycling, as well as biodiversity and extinction risk. Over the past year, PLOS has published a wide range of research on the ecological impacts of climate change. The breadth of topics includes everything from impacts of climate change on the immune competency of marine mammals to the global distribution of mosquito borne-diseases and their hosts. This collection also includes some recent research modeling and analyzing the global ecological impacts of climate change as well as the synthesis of datasets from literature in two new systematic reviews . PLOS journals recognize the importance in publishing and highlighting climate change research, and we thank our authors for their significant contributions to this field.
Image Credit: (Clockwise from top): William Warby. Flickr.com. Thomas Vignaud. PLOS Biology. 2011. 9(4). Colombi et al. PLOS ONE. 2013. Soto-Azat et al. PLOS ONE. 2013.
- Large-scale Vulnerability Assessments
- Pests and Disturbance
- Soils in a Warming World
- Climate Impacts: Plants
- Climate Impacts: Oceans
- Climate Impacts: Animals
- Ecosystems and Human Health
- Learning from the Past
- Management and Conservation
- Interactions and Communities
- 2015-2016 Collection Update
- Associated Collection Blogs
- Image credit 10.1371/journal.pone.0173812 PLOS ONE A systematic review of ecological attributes that confer resilience to climate change in environmental restoration March 16, 2017 Britta L. Timpane-Padgham, Tim Beechie, Terrie Klinger
- Image credit 10.1371/journal.pbio.2001104 PLOS Biology Climate-Related Local Extinctions Are Already Widespread among Plant and Animal Species December 8, 2016 John J. Wiens
- Image credit 10.1371/journal.pone.0157929 PLOS ONE Human Impacts and Climate Change Influence Nestedness and Modularity in Food-Web and Mutualistic Networks June 20, 2016 Kazuhiro Takemoto, Kosuke Kajihara
- Image credit 10.1371/journal.pone.0153589 PLOS ONE Incorporating Anthropogenic Influences into Fire Probability Models: Effects of Human Activity and Climate Change on Fire Activity in California April 28, 2016 Michael L. Mann, Enric Batllori, Max A. Moritz, Eric K. Waller, Peter Berck, Alan L. Flint, Lorraine E. Flint, Emmalee Dolfi
- Image credit 10.1371/journal.pbio.1002415 PLOS Biology Remotely Sensed High-Resolution Global Cloud Dynamics for Predicting Ecosystem and Biodiversity Distributions March 31, 2016 Adam M. Wilson, Walter Jetz
- Image credit 10.1371/journal.pbio.1002413 PLOS Biology Conservation Research Is Not Happening Where It Is Most Needed March 29, 2016 Kerrie A. Wilson, Nancy A. Auerbach, Katerina Sam, Ariana G. Magini, Alexander St. L. Moss, Simone D. Langhans, Sugeng Budiharta, Dilva Terzano, Erik Meijaard
- Image credit 10.1371/journal.pone.0122163 PLOS ONE Drivers and Patterns of Ground-Dwelling Beetle Biodiversity across Northern Canada April 22, 2015 Crystal Ernst, Christopher Buddle
- Image credit 10.1371/journal.pone.0120198 PLOS ONE Dead Shrimp Blues: A Global Assessment of Extinction Risk in Freshwater Shrimps (Crustacea: Decapoda: Caridea) March 25, 2015 Sammy De Grave, Kevin Smith, Nils Adeler, Dave Allen, Fernando Alvarez, Arthur Anker, Yixiong Cai, Savrina Carrizo, Werner Klotz, Fernando Mantelatto, Timothy Page, Jhy-Yun Shy, José Luis Villalobos, Daisy Wowor
- Image credit 10.1371/journal.pone.0113118 PLOS ONE Trends in Extinction Risk for Imperiled Species in Canada November 17, 2014 Brett Favaro, Danielle Claar, Caroline Fox, Cameron Freshwater, Jessica Holden, Allan Roberts
- Image credit 10.1371/journal.pone.0113746 PLOS ONE Projected Polar Bear Sea Ice Habitat in the Canadian Arctic Archipelago November 26, 2014 Stephen Hamilton, Laura Castro de la Guardia, Andrew Derocher, Vicki Sahanatien, Bruno Tremblay, David Huard
- Image credit 10.1371/journal.pone.0107525 PLOS ONE Persistence and Change in Community Composition of Reef Corals through Present, Past, and Future Climates October 1, 2014 Peter Edmunds, Mehdi Adjeroud, Marissa Baskett, Iliana Baums, Ann Budd, Robert Carpenter, Nicholas Fabina, Tung-Yung Fan, Erik Franklin, Kevin Gross, Xueying Han, Lianne Jacobson, James Klaus, Tim McClanahan, Jennifer O'Leary, Madeleine van Oppen, Xavier Pochon, Hollie Putnam, Tyler Smith, Michael Stat, Hugh Sweatman, Robert van Woesik, Ruth Gates
- Image credit 10.1371/journal.pbio.1001682 PLOS Biology Biotic and Human Vulnerability to Projected Changes in Ocean Biogeochemistry over the 21st Century October 15, 2013 Camilo Mora, Chih-Lin Wei, Audrey Rollo, Teresa Amaro, Amy Baco, David Billett, Laurent Bopp, Qi Chen, Mark Collier, Roberto Danovaro, Andrew Gooday, Benjamin Grupe, Paul Halloran, Jeroen Ingels, Daniel Jones, Lisa Levin, Hideyuki Nakano, Karl Norling, Eva Ramirez-Llodra, Michael Rex, Henry Ruhl, Craig Smith, Andrew Sweetman, Andrew Thurber, Jerry Tjiputra, Paolo Usseglio, Les Watling, Tongwen Wu, Moriaki Yasuhara
- Image credit 10.1371/journal.pone.0074989 PLOS ONE Threats from Climate Change to Terrestrial Vertebrate Hotspots in Europe September 16, 2013 Luigi Maiorano, Giovanni Amori, Massimo Capula, Alessandra Falcucci, Monica Masi, Alessandro Montemaggiori, Julien Pottier, Achilleas Psomas, Carlo Rondinini, Danilo Russo, Niklaus Zimmermann, Luigi Boitani, Antoine Guisan
- Image credit 10.1371/journal.pone.0065427 PLOS ONE Identifying the World’s Most Climate Change Vulnerable Species: A Systematic Trait-Based Assessment of all Birds, Amphibians and Corals June 12, 2013 Wendy B. Foden, Stuart H. M. Butchart, Simon N. Stuart, Jean-Christophe Vié, H. Resit Akçakaya, Ariadne Angulo, Lyndon M. DeVantier, Alexander Gutsche, Emre Turak, Long Cao, Simon D. Donner, Vineet Katariya, Rodolphe Bernard, Robert A. Holland, Adrian F. Hughes, Susannah E. O’Hanlon, Stephen T. Garnett, Çagan H. Şekercioğlu, Georgina M. Mace
- Image credit 10.1371/journal.pone.0029507 PLOS ONE A Climate Change Vulnerability Assessment of California’s At-Risk Birds March 2, 2012 Thomas Gardali, Nathaniel Seavy, Ryan DiGaudio, Lyann Comrack
- Image credit 10.1371/journal.pone.0153237 PLOS ONE Future Risks of Pest Species under Changing Climatic Conditions April 7, 2016 Lisa Biber-Freudenberger, Jasmin Ziemacki, Henri E. Z. Tonnang, Christian Borgemeister
- Image credit 10.1371/journal.pcbi.1004151 PLOS Computational Biology Modeling the Impact of White-Plague Coral Disease in Climate Change Scenarios June 18, 2015 Assaf Zvuloni, Guy Katriel, Yossi Loya, Lewi Stone, Yael Artzy-Randrup
- Image credit 10.1371/journal.pone.0106971 PLOS ONE Historical, Observed, and Modeled Wildfire Severity in Montane Forests of the Colorado Front Range September 24, 2014 Rosemary Sherriff, Rutherford Platt, Thomas Veblen, Tania Schoennagel, Meredith Gartner
- Image credit 10.1371/journal.pone.0092770 PLOS ONE Growth-Mortality Relationships in Piñon Pine ( Pinus edulis) during Severe Droughts of the Past Century: Shifting Processes in Space and Time May 2, 2014 Alison Macalady, Harald Bugmann
- Image credit 10.1371/journal.pone.0075438 PLOS ONE Climate Change May Boost the Invasion of the Asian Needle Ant October 4, 2013 Cleo Bertelsmeier, Benoît Guénard, Franck Courchamp
- Image credit 10.1371/journal.pone.0059687 PLOS ONE Urban Warming Drives Insect Pest Abundance on Street Trees March 27, 2013 Emily Meineke, Robert Dunn, Joseph Sexton, Steven Frank
- Image credit 10.1371/journal.pone.0024528 PLOS ONE Some Like It Hot: The Influence and Implications of Climate Change on Coffee Berry Borer ( Hypothenemus hampei) and Coffee Production in East Africa September 14, 2011 Juliana Jaramillo, Eric Muchugu, Fernando E. Vega, Aaron Davis, Christian Borgemeister, Adenirin Chabi-Olaye
- Image credit 10.1371/journal.pone.0165448 PLOS ONE The Role of Microbial Community Composition in Controlling Soil Respiration Responses to Temperature October 31, 2016 Marc D. Auffret, Kristiina Karhu, Amit Khachane, Jennifer A. J. Dungait, Fiona Fraser, David W. Hopkins, Philip A. Wookey, Brajesh K. Singh, Thomas E. Freitag, Iain P. Hartley, James I. Prosser
- Image credit 10.1371/journal.pone.0156083 PLOS ONE Climate Change and Maize Yield in Iowa May 24, 2016 Hong Xu, Tracy E. Twine, Evan Girvetz
- Image credit 10.1371/journal.pone.0153357 PLOS ONE Deforestation Induced Climate Change: Effects of Spatial Scale April 21, 2016 Patrick Longobardi, Hugo Beltrami, Michael Eby, Alvaro Montenegro
- Image credit 10.1371/journal.pone.0123999 PLOS ONE Properties of Soil Pore Space Regulate Pathways of Plant Residue Decomposition and Community Structure of Associated Bacteria April 24, 2015 Wakene Negassa, Andrey Guber, Alexandra Kravchenko, Terence Marsh, Britton Hildebrandt, Mark Rivers
- Image credit 10.1371/journal.pone.0119184 PLOS ONE Turnover of Grassland Roots in Mountain Ecosystems Revealed by Their Radiocarbon Signature: Role of Temperature and Management March 3, 2015 Jens Leifeld, Stefanie Meyer, Karen Budge, Maria Teresa Sebastia, Michael Zimmermann, Juerg Fuhrer
- Image credit 10.1371/journal.pone.0158823 PLOS ONE Climate Change Impairs Nitrogen Cycling in European Beech Forests July 13, 2016 Michael Dannenmann, Carolin Bimüller, Silvia Gschwendtner, Martin Leberecht, Javier Tejedor, Silvija Bilela, Rainer Gasche, Marc Hanewinkel, Andri Baltensweiler, Ingrid Kögel-Knabner, Andrea Polle, Michael Schloter, Judy Simon, Heinz Rennenberg
- Image credit 10.1371/journal.pone.0151811 PLOS ONE Exploring Climate Niches of Ponderosa Pine ( Pinus ponderosa Douglas ex Lawson) Haplotypes in the Western United States: Implications for Evolutionary History and Conservation March 17, 2016 Douglas J. Shinneman, Robert E. Means, Kevin M. Potter, Valerie D. Hipkins
- Image credit 10.1371/journal.pone.0148607 PLOS ONE Functional Resilience against Climate-Driven Extinctions – Comparing the Functional Diversity of European and North American Tree Floras February 5, 2016 Mario Liebergesell, Björn Reu, Ulrike Stahl, Martin Freiberg, Erik Welk, Jens Kattge, J. Hans C. Cornelissen, Josep Peñuelas, Christian Wirth
- Image credit 10.1371/journal.pone.0105380 PLOS ONE Pushing the Pace of Tree Species Migration August 27, 2014 Eli Lazarus, Brian McGill
- Image credit 10.1371/journal.pone.0100577 PLOS ONE Growth and Phenology of Three Dwarf Shrub Species in a Six-Year Soil Warming Experiment at the Alpine Treeline June 23, 2014 Alba Anadon-Rosell, Christian Rixen, Paolo Cherubini, Sonja Wipf, Frank Hagedorn, Melissa Dawes
- Image credit 10.1371/journal.pone.0053788 PLOS ONE Record-Breaking Early Flowering in the Eastern United States January 16, 2013 Elizabeth Ellwood, Stanley Temple, Richard Primack, Nina Bradley, Charles Davis
- Image credit 10.1371/journal.pone.0047981 PLOS ONE The Impact of Climate Change on Indigenous Arabica Coffee ( Coffea arabica ): Predicting Future Trends and Identifying Priorities November 7, 2012 Aaron Davis, Tadesse Gole, Susana Baena, Justin Moat
- Image credit 10.1371/journal.pone.0179359 PLOS ONE Climatic anomaly affects the immune competence of California sea lions June 28, 2017 Marina Banuet-Martínez, Wendy Espinosa-de Aquino, Fernando R. Elorriaga-Verplancken, Adriana Flores-Morán, Olga P. García, Mariela Camacho, Karina Acevedo-Whitehouse
- Image credit 10.1371/journal.pone.0159068 PLOS ONE Influence of Ocean Acidification on a Natural Winter-to-Summer Plankton Succession: First Insights from a Long-Term Mesocosm Study Draw Attention to Periods of Low Nutrient Concentrations August 15, 2016 Lennart T. Bach, Jan Taucher, Tim Boxhammer, Andrea Ludwig, The Kristineberg KOSMOS Consortium , Eric P. Achterberg, María Algueró-Muñiz, Leif G. Anderson, Jessica Bellworthy, Jan Büdenbender, Jan Czerny, Ylva Ericson, Mario Esposito, Matthias Fischer, Mathias Haunost, Dana Hellemann, Henriette G. Horn, Thomas Hornick, Jana Meyer, Michael Sswat, Maren Zark, Ulf Riebesell
- Image credit 10.1371/journal.pone.0139223 PLOS ONE Transcriptomic Changes in Coral Holobionts Provide Insights into Physiological Challenges of Future Climate and Ocean Change October 28, 2015 Paulina Kaniewska, Chon-Kit Kenneth Chan, David Kline, Edmund Yew Siang Ling, Nedeljka Rosic, David Edwards, Ove Hoegh-Guldberg, Sophie Dove
- Image credit 10.1371/journal.pone.0115655 PLOS ONE Global Warming and Mass Mortalities of Benthic Invertebrates in the Mediterranean Sea December 23, 2014 Irene Rivetti, Simonetta Fraschetti, Piero Lionello, Enrico Zambianchi, Ferdinando Boero
- Image credit 10.1371/journal.pone.0074413 PLOS ONE Impacts of Temperature on Primary Productivity and Respiration in Naturally Structured Macroalgal Assemblages September 13, 2013 Leigh Tait, David Schiel
- Image credit 10.1371/journal.pone.0066475 PLOS ONE Climate Change and Eutrophication Induced Shifts in Northern Summer Plankton Communities June 12, 2013 Sanna Suikkanen, Silvia Pulina, Jonna Engström-Öst, Maiju Lehtiniemi, Sirpa Lehtinen, Andreas Brutemark
- Image credit 10.1371/journal.pone.0060568 PLOS ONE Climate Change Winners: Receding Ice Fields Facilitate Colony Expansion and Altered Dynamics in an Adélie Penguin Metapopulation April 3, 2013 Michelle LaRue, David Ainley, Matthew Swanson, Katie Dugger, Phil Lyver, Kerry Barton, Grant Ballard
- Image credit 10.1371/journal.pone.0050685 PLOS ONE Coral Thermal Tolerance: Tuning Gene Expression to Resist Thermal Stress November 30, 2012 Anthony Bellantuono, Camila Granados-Cifuentes, David Miller, Ove Hoegh-Guldberg, Mauricio Rodriguez-Lanetty
- Image credit 10.1371/journal.pone.0033353 PLOS ONE Contrasting Patterns of Coral Bleaching Susceptibility in 2010 Suggest an Adaptive Response to Thermal Stress March 9, 2012 James Guest, Andrew Baird, Jeffrey Maynard, Efin Muttaqin, Alasdair Edwards, Stuart Campbell, Katie Yewdall, Yang Amri Affendi, Loke Ming Chou
- Image credit 10.1371/journal.pbio.1002592 PLOS Biology Bigger Is Fitter? Quantitative Genetic Decomposition of Selection Reveals an Adaptive Evolutionary Decline of Body Mass in a Wild Rodent Population January 26, 2017 Timothée Bonnet, Peter Wandeler, Glauco Camenisch, Erik Postma
- Image credit 10.1371/journal.pone.0165073 PLOS ONE Assessing Mammal Exposure to Climate Change in the Brazilian Amazon November 9, 2016 Bruno R. Ribeiro, Lilian P. Sales, Paulo De Marco Jr., Rafael Loyola
- Image credit 10.1371/journal.pone.0152636 PLOS ONE Range Expansion of Moose in Arctic Alaska Linked to Warming and Increased Shrub Habitat April 13, 2016 Ken D. Tape, David D. Gustine, Roger W. Ruess, Layne G. Adams, Jason A. Clark
- Image credit 10.1371/journal.pone.0142213 PLOS ONE Increased Land Use by Chukchi Sea Polar Bears in Relation to Changing Sea Ice Conditions November 18, 2015 Karyn D. Rode, Ryan R. Wilson, Eric V. Regehr, Michelle St. Martin, David C. Douglas, Jay Olson
- Image credit 10.1371/journal.pone.0135350 PLOS ONE Conservation Status of North American Birds in the Face of Future Climate Change September 2, 2015 Gary M. Langham, Justin G. Schuetz, Trisha Distler, Candan U. Soykan, Chad Wilsey
- Image credit 10.1371/journal.pone.0098351 PLOS ONE Individualistic Population Responses of Five Frog Species in Two Changing Tropical Environments over Time May 30, 2014 Mason Ryan, Michael Fuller, Norman Scott, Joseph Cook, Steven Poe, Beatriz Willink, Gerardo Chaves, Federico Bolaños
- Image credit 10.1371/journal.pone.0085602 PLOS ONE Climate Change Increases Reproductive Failure in Magellanic Penguins January 29, 2014 Dee Boersma, Ginger Rebstock
- Image credit 10.1371/journal.pone.0087245 PLOS ONE Signals of Climate Change in Butterfly Communities in a Mediterranean Protected Area January 29, 2014 Konstantina Zografou, Vassiliki Kati, Andrea Grill, Robert Wilson, Elli Tzirkalli, Lazaros Pamperis, John Halley
- Image credit 10.1371/journal.pone.0087291 PLOS ONE Designing Optimized Multi-Species Monitoring Networks to Detect Range Shifts Driven by Climate Change: A Case Study with Bats in the North of Portugal January 27, 2014 Francisco Amorim, Sílvia Carvalho, João Honrado, Hugo Rebelo
- Image credit 10.1371/journal.pone.0082490 PLOS ONE Plants, Birds and Butterflies: Short-Term Responses of Species Communities to Climate Warming Vary by Taxon and with Altitude January 8, 2014 Tobias Roth, Matthias Plattner, Valentin Amrhein
- Image credit 10.1371/journal.pone.0067065 PLOS ONE Long-Term Climate Sensitivity of Grazer Performance: A Cross-Site Study June 20, 2013 Joseph Craine
- Image credit 10.1371/journal.pone.0065408 PLOS ONE Citizen Science Reveals an Extensive Shift in the Winter Distribution of Migratory Western Grebes June 19, 2013 Scott Wilson, Eric Anderson, Amy Wilson, Douglas Bertram, Peter Arcese
- Image credit 10.1371/journal.pbio.1001517 PLOS Biology The Case of the Missing Mechanism: How Does Temperature Influence Seasonal Timing in Endotherms? April 2, 2013 Samuel Caro, Sonja Schaper, Roelof Hut, Gregory Ball, Marcel Visser
- Image credit 10.1371/journal.pone.0163863 PLOS ONE Climate Change Influences on the Global Potential Distribution of the Mosquito Culex quinquefasciatus , Vector of West Nile Virus and Lymphatic Filariasis October 3, 2016 Abdallah M. Samy, Arwa H. Elaagip, Mohamed A. Kenawy, Constância F. J. Ayres, A. Townsend Peterson, Doaa E. Soliman
- Image credit 10.1371/journal.pone.0105480 PLOS ONE Urbanisation at Multiple Scales Is Associated with Larger Size and Higher Fecundity of an Orb-Weaving Spider August 20, 2014 Elizabeth Lowe, Shawn Wilder, Dieter Hochuli
- Image credit 10.1371/journal.pntd.0003210 PLOS Neglected Tropical Diseases Cutaneous Leishmaniasis and Sand Fly Fluctuations Are Associated with El Niño in Panamá October 2, 2014 Luis Fernando Chaves, Jose Calzada, Anayansi Valderrama, Azael Saldaña
- Image credit 10.1371/journal.pone.0066071 PLOS ONE Pathogen-Host Associations and Predicted Range Shifts of Human Monkeypox in Response to Climate Change in Central Africa July 31, 2013 Henri Thomassen, Trevon Fuller, Salvi Asefi-Najafabady, Julia Shiplacoff, Prime Mulembakani, Seth Blumberg, Sara Johnston, Neville Kisalu, Timothée Kinkela, Joseph Fair, Nathan Wolfe, Robert Shongo, Matthew LeBreton, Hermann Meyer, Linda Wright, Jean-Jacques Muyembe, Wolfgang Buermann, Emile Okitolonda, Lisa Hensley, James Lloyd-Smith, Thomas Smith, Anne Rimoin
- Image credit 10.1371/journal.pone.0060874 PLOS ONE Climate Change and Range Expansion of the Asian Tiger Mosquito ( Aedes albopictus ) in Northeastern USA: Implications for Public Health Practitioners April 2, 2013 ILIA ROCHLIN, Dominick Ninivaggi, Michael Hutchinson, Ary Farajollahi
- Image credit 10.1371/journal.pone.0034076 PLOS ONE Changes to Airborne Pollen Counts across Europe April 13, 2012 Chiara Ziello, Tim Sparks, Nicole Estrella, Jordina Belmonte, Karl Bergmann, Edith Bucher, Maria Antonia Brighetti, Athanasios Damialis, Monique Detandt, Carmen Galán, Regula Gehrig, Lukasz Grewling, Adela Gutiérrez Bustillo, Margrét Hallsdóttir, Marie-Claire Kockhans-Bieda, Concepción De Linares, Dorota Myszkowska, Anna Pàldy, Adriana Sánchez, Matthew Smith, Michel Thibaudon, Alessandro Travaglini, Agnieszka Uruska, Rosa Valencia-Barrera, Despoina Vokou, Reinhard Wachter, Letty de Weger, Annette Menzel
- Image credit 10.1371/journal.pone.0161462 PLOS ONE How Climate Change Beliefs among U.S. Teachers Do and Do Not Translate to Students September 7, 2016 Kathryn T. Stevenson, M. Nils Peterson, Amy Bradshaw
- Image credit 10.1371/journal.pone.0167983 PLOS ONE Narrative Style Influences Citation Frequency in Climate Change Science December 15, 2016 Ann Hillier, Ryan P. Kelly, Terrie Klinger
- Image credit 10.1371/journal.pone.0115246 PLOS ONE Paleoceanographic Insights on Recent Oxygen Minimum Zone Expansion: Lessons for Modern Oceanography January 28, 2015 Sarah E. Moffitt, Russell A. Moffitt, Wilson Sauthoff, Catherine V. Davis, Kathryn Hewett, Tessa M. Hill
- Image credit 10.1371/journal.pone.0114282 PLOS ONE Western Spruce Budworm Outbreaks Did Not Increase Fire Risk over the Last Three Centuries: A Dendrochronological Analysis of Inter-Disturbance Synergism December 19, 2014 Aquila Flower, Daniel Gavin, Emily Heyerdahl, Russel Parsons, Gregory Cohn
- Image credit 10.1371/journal.pbio.1001949 PLOS Biology Plant Ecological Strategies Shift Across the Cretaceous–Paleogene Boundary September 16, 2014 Benjamin Blonder, Dana Royer, Kirk Johnson, Ian Miller, Brian Enquist
- Image credit 10.1371/journal.pone.0106651 PLOS ONE The Impact of 850,000 Years of Climate Changes on the Structure and Dynamics of Mammal Food Webs September 10, 2014 Hedvig Nenzen, Sara Varela, Daniel Montoya
- Image credit 10.1371/journal.pone.0102090 PLOS ONE Vulnerability of Mediterranean Ecosystems to Long-Term Changes along the Coast of Israel July 8, 2014 David Kaniewski, Elise Van Campo, Christophe Morhange, Joël Guiot, Dov Zviely, Sabine Le Burel, Thierry Otto, Michal Artzy
- Image credit 10.1371/journal.pone.0071797 PLOS ONE Tree Migration-Rates: Narrowing the Gap between Inferred Post-Glacial Rates and Projected Rates August 26, 2013 Angelica Feurdean, Shonil Bhagwat, Katherine Willis, H. John Birks, Heike Lischke, Thomas Hickler
- Image credit 10.1371/journal.pgen.1000554 PLOS Genetics Rapid Response of a Marine Mammal Species to Holocene Climate and Habitat Change July 10, 2009 Mark de Bruyn, Brenda L. Hall, Lucas F. Chauke, Carlo Baroni, Paul L. Koch, A. Rus Hoelzel
- Image credit 10.1371/journal.pbio.2002634 PLOS Biology AEDT: A new concept for ecological dynamics in the ever-changing world May 30, 2017 Peter Chesson
- Image credit 10.1371/journal.pone.0165797 PLOS ONE The Impacts of Dietary Change on Greenhouse Gas Emissions, Land Use, Water Use, and Health: A Systematic Review November 3, 2016 Lukasz Aleksandrowicz, Rosemary Green, Edward J. M. Joy, Pete Smith, Andy Haines
- Image credit Walden & Lindborg PLOS Ecology Community Related blog: Restoring what was lost: semi-natural grassland habitat restoration in Sweden May 31, 2016 Alexandra (Sasha) Wright
- Image credit 10.1371/journal.pone.0155836 PLOS ONE Long Term Positive Effect of Grassland Restoration on Plant Diversity – Success or Not? May 19, 2016 Emelie Waldén, Regina Lindborg
- Image credit 10.1371/journal.pone.0124014 PLOS ONE Noah’s Ark Conservation Will Not Preserve Threatened Ecological Communities under Climate Change April 16, 2015 Rebecca Harris, Oberon Carter, Louise Gilfedder, Luciana Porfirio, Greg Lee, Nathan Bindoff
- Image credit 10.1371/journal.pone.0121143 PLOS ONE Managed Bumblebees Outperform Honeybees in Increasing Peach Fruit Set in China: Different Limiting Processes with Different Pollinators March 23, 2015 Hong Zhang, Jiaxing Huang, Paul H. Williams, Bernard E. Vaissière, Zhiyong Zhou, Qinbao Gai, Jie Dong, Jiandong An
- Image credit 10.1371/journal.pone.0121137 PLOS ONE Ecological and Geographical Analysis of the Distribution of the Mountain Tapir ( Tapirus pinchaque ) in Ecuador: Importance of Protected Areas in Future Scenarios of Global Warming March 23, 2015 H. Mauricio Ortega-Andrade, David Prieto-Torres, Ignacio Gómez-Lora, Diego Lizcano
- Image credit 10.1371/journal.pone.0110742 PLOS ONE Demographic Outcomes and Ecosystem Implications of Giant Tortoise Reintroduction to Española Island, Galapagos October 28, 2014 James Gibbs, Elizabeth Hunter, Kevin Shoemaker, Washington Tapia, Linda Cayot
- Image credit 10.1371/journal.pone.0086487 PLOS ONE Land Use Compounds Habitat Losses under Projected Climate Change in a Threatened California Ecosystem January 21, 2014 Erin Riordan, Philip Rundel
- Image credit 10.1371/journal.pone.0075814 PLOS ONE How to Decide Whether to Move Species Threatened by Climate Change October 16, 2013 Tracy Rout, Eve McDonald-Madden, Tara Martin, Nicola Mitchell, Hugh Possingham, Doug Armstrong
- Image credit 10.1371/journal.pone.0181333 PLOS ONE Plant species dispersed by Galapagos tortoises surf the wave of habitat suitability under anthropogenic climate change July 20, 2017 Diego Ellis-Soto, Stephen Blake, Alaaeldin Soultan, Anne Guézou, Fredy Cabrera, Stefan Lötters
- Image credit 10.1371/journal.pone.0140275 PLOS ONE Extreme Environments Facilitate Hybrid Superiority – The Story of a Successful Daphnia galeata × longispina Hybrid Clone October 8, 2015 Johanna Griebel, Sabine Gießler, Monika Poxleitner, Amanda Navas Faria, Mingbo Yin, Justyna Wolinska
- Image credit 10.1371/journal.pone.0119276 PLOS ONE Effect of Landscape Pattern on Insect Species Density within Urban Green Spaces in Beijing, China March 20, 2015 Zhimin Su, Xiaoma Li, Weiqi Zhou, Zhiyun Ouyang
- Image credit 10.1371/journal.pone.0082886 PLOS ONE Assessing the Effects of Climate on Host-Parasite Interactions: A Comparative Study of European Birds and Their Parasites December 31, 2013 Anders Møller, Santiago Merino, Juan José Soler, Anton Antonov, Elisa Badás, Miguel Calero-Torralbo, Florentino de Lope, Tapio Eeva, Jordi Figuerola, Einar Flensted-Jensen, Laszlo Z. Garamszegi, Sonia González-Braojos, Helga Gwinner, Sveinn Hanssen, Dieter Heylen, Petteri Ilmonen, Kurt Klarborg, Erkki Korpimäki, Javier Martinez, Josue Martinez-de la Puente, Alfonso Marzal, Erik Matthysen, Piotr Matyjasiak, Mercedes Molina-Morales, Juan Moreno, Timothy Mousseau, Jan Nielsen, Péter Pap, Juan Rivero-de Aguilar, Peter Shurulinkov, Tore Slagsvold, Tibor Szép, Eszter Szöllősiw, Janos Török, Radovan Vaclav, Francisco Valera, Nadia Ziane
- Image credit 10.1371/journal.pbio.1001705 PLOS Biology Water Stress Strengthens Mutualism Among Ants, Trees, and Scale Insects November 5, 2013 Elizabeth Pringle, Erol Akçay, Ted Raab, Rodolfo Dirzo, Deborah Gordon
- Image credit 10.1371/journal.pbio.1001685 PLOS Biology Coevolution and the Effects of Climate Change on Interacting Species October 22, 2013 Tobin Northfield, Anthony Ives
- Image credit 10.1371/journal.pbio.1001569 PLOS Biology Rare Species Support Vulnerable Functions in High-Diversity Ecosystems May 28, 2013 David Mouillot, David Bellwood, Christopher Baraloto, Jerome Chave, Rene Galzin, Mireille Harmelin-Vivien, Michel Kulbicki, Sebastien Lavergne, Sandra Lavorel, Nicolas Mouquet, C.E. Timothy Paine, Julien Renaud, Wilfried Thuiller
- Image credit William Warby. Flickr.com. Thomas Vignaud. PLOS Biology. 2011. 9(4). Colombi et al. PLOS ONE. 2013. Soto-Azat et al. PLOS ONE. 2013. PLOS Ecology Community Ecological Impacts of Climate Change Collection 2015-2016 August 1, 2016 Jeff Atkins
- Image credit J. Atkins PLOS Ecology Community Playing with matches: Incorporating human activity into wildfire forecasting May 12, 2016 Jeff Atkins
- Image credit Wilson & Jetz (2016) PLOS Ecology Community Remotely-sensed cloud cover predicts biodiversity & climate change vulnerability April 15, 2016 Alexandra (Sasha) Wright
- Image credit 10.1371/journal.pbio.1002413 PLOS Ecology Community Is Biodiversity Research Biased? April 11, 2016 Jeff Atkins
- Image credit pone.0115246 PLOS Ecology Community Ice ages and suffocating ocean zones: reconstructing past oxygen minimums with an eye to the future February 8, 2016 Jens Hegg
- Image credit David Shankbone PLOS Ecology Community Life as a Bird – biodiversity management in New York City January 27, 2016 Lauren Alleman & Sasha Wright
- Image credit N. Miller-Struttmann PLOS Ecology Community Where have all the flowers gone: complexity & worldwide bee declines by Nicole Miller-Struttmann January 11, 2016 Nicole Miller-Struttmann, Assistant Professor of Biology at SUNY – Old Westbury
- Image credit (Clockwise from top): William Warby. Flickr.com. Thomas Vignaud. PLOS Biology. 2011. 9(4). Colombi et al. PLOS ONE. 2013. Soto-Azat et al. PLOS ONE. 2013. PLOS Collections Blog The 2015 Ecological Impacts of Climate Change Collection August 19, 2015 Ben Bond-Lamberty
- Image credit William Warby. Flickr.com. Thomas Vignaud. PLOS Biology. 2011. 9(4). Colombi et al. PLOS ONE. 2013. Soto-Azat et al. PLOS ONE. 2013. EveryONE The 2014 Ecological Impacts of Climate Change Collection August 8, 2014 Ben Bond-Lamberty
- Image credit William Warby. Flickr.com. Thomas Vignaud. PLOS Biology. 2011. 9(4). Colombi et al. PLOS ONE. 2013. Soto-Azat et al. PLOS ONE. 2013 EveryONE Announcing the Ecological Impacts of Climate Change Collection August 5, 2013 Ben Bond-Lamberty
- Image credit Gideon Pisanty (Gidip) PLOS Blogs What Ecologists are (Most) Worried About Right Now: 5 Emerging Trends in Climate Change Ecology August 12, 2014 John H. Matthews
This collection is curated by PLOS ONE Section Editor, Dr. Ben Bond-Lamberty and the PLOS Ecology Community Editors
Receive monthly email updates

An official website of the United States government
The .gov means it’s official. Federal government websites often end in .gov or .mil. Before sharing sensitive information, make sure you’re on a federal government site.
The site is secure. The https:// ensures that you are connecting to the official website and that any information you provide is encrypted and transmitted securely.
- Publications
- Account settings
Preview improvements coming to the PMC website in October 2024. Learn More or Try it out now .
- Advanced Search
- Journal List
- Springer Nature - PMC COVID-19 Collection

A review of the global climate change impacts, adaptation, and sustainable mitigation measures
Kashif abbass.
1 School of Economics and Management, Nanjing University of Science and Technology, Nanjing, 210094 People’s Republic of China
Muhammad Zeeshan Qasim
2 Jiangsu Key Laboratory of Chemical Pollution Control and Resources Reuse, School of Environmental and Biological Engineering, Nanjing University of Science and Technology, Xiaolingwei 200, Nanjing, 210094 People’s Republic of China
Huaming Song
Muntasir murshed.
3 School of Business and Economics, North South University, Dhaka, 1229 Bangladesh
4 Department of Journalism, Media and Communications, Daffodil International University, Dhaka, Bangladesh
Haider Mahmood
5 Department of Finance, College of Business Administration, Prince Sattam Bin Abdulaziz University, 173, Alkharj, 11942 Saudi Arabia
Ijaz Younis
Associated data.
Data sources and relevant links are provided in the paper to access data.
Climate change is a long-lasting change in the weather arrays across tropics to polls. It is a global threat that has embarked on to put stress on various sectors. This study is aimed to conceptually engineer how climate variability is deteriorating the sustainability of diverse sectors worldwide. Specifically, the agricultural sector’s vulnerability is a globally concerning scenario, as sufficient production and food supplies are threatened due to irreversible weather fluctuations. In turn, it is challenging the global feeding patterns, particularly in countries with agriculture as an integral part of their economy and total productivity. Climate change has also put the integrity and survival of many species at stake due to shifts in optimum temperature ranges, thereby accelerating biodiversity loss by progressively changing the ecosystem structures. Climate variations increase the likelihood of particular food and waterborne and vector-borne diseases, and a recent example is a coronavirus pandemic. Climate change also accelerates the enigma of antimicrobial resistance, another threat to human health due to the increasing incidence of resistant pathogenic infections. Besides, the global tourism industry is devastated as climate change impacts unfavorable tourism spots. The methodology investigates hypothetical scenarios of climate variability and attempts to describe the quality of evidence to facilitate readers’ careful, critical engagement. Secondary data is used to identify sustainability issues such as environmental, social, and economic viability. To better understand the problem, gathered the information in this report from various media outlets, research agencies, policy papers, newspapers, and other sources. This review is a sectorial assessment of climate change mitigation and adaptation approaches worldwide in the aforementioned sectors and the associated economic costs. According to the findings, government involvement is necessary for the country’s long-term development through strict accountability of resources and regulations implemented in the past to generate cutting-edge climate policy. Therefore, mitigating the impacts of climate change must be of the utmost importance, and hence, this global threat requires global commitment to address its dreadful implications to ensure global sustenance.
Introduction
Worldwide observed and anticipated climatic changes for the twenty-first century and global warming are significant global changes that have been encountered during the past 65 years. Climate change (CC) is an inter-governmental complex challenge globally with its influence over various components of the ecological, environmental, socio-political, and socio-economic disciplines (Adger et al. 2005 ; Leal Filho et al. 2021 ; Feliciano et al. 2022 ). Climate change involves heightened temperatures across numerous worlds (Battisti and Naylor 2009 ; Schuurmans 2021 ; Weisheimer and Palmer 2005 ; Yadav et al. 2015 ). With the onset of the industrial revolution, the problem of earth climate was amplified manifold (Leppänen et al. 2014 ). It is reported that the immediate attention and due steps might increase the probability of overcoming its devastating impacts. It is not plausible to interpret the exact consequences of climate change (CC) on a sectoral basis (Izaguirre et al. 2021 ; Jurgilevich et al. 2017 ), which is evident by the emerging level of recognition plus the inclusion of climatic uncertainties at both local and national level of policymaking (Ayers et al. 2014 ).
Climate change is characterized based on the comprehensive long-haul temperature and precipitation trends and other components such as pressure and humidity level in the surrounding environment. Besides, the irregular weather patterns, retreating of global ice sheets, and the corresponding elevated sea level rise are among the most renowned international and domestic effects of climate change (Lipczynska-Kochany 2018 ; Michel et al. 2021 ; Murshed and Dao 2020 ). Before the industrial revolution, natural sources, including volcanoes, forest fires, and seismic activities, were regarded as the distinct sources of greenhouse gases (GHGs) such as CO 2 , CH 4 , N 2 O, and H 2 O into the atmosphere (Murshed et al. 2020 ; Hussain et al. 2020 ; Sovacool et al. 2021 ; Usman and Balsalobre-Lorente 2022 ; Murshed 2022 ). United Nations Framework Convention on Climate Change (UNFCCC) struck a major agreement to tackle climate change and accelerate and intensify the actions and investments required for a sustainable low-carbon future at Conference of the Parties (COP-21) in Paris on December 12, 2015. The Paris Agreement expands on the Convention by bringing all nations together for the first time in a single cause to undertake ambitious measures to prevent climate change and adapt to its impacts, with increased funding to assist developing countries in doing so. As so, it marks a turning point in the global climate fight. The core goal of the Paris Agreement is to improve the global response to the threat of climate change by keeping the global temperature rise this century well below 2 °C over pre-industrial levels and to pursue efforts to limit the temperature increase to 1.5° C (Sharma et al. 2020 ; Sharif et al. 2020 ; Chien et al. 2021 .
Furthermore, the agreement aspires to strengthen nations’ ability to deal with the effects of climate change and align financing flows with low GHG emissions and climate-resilient paths (Shahbaz et al. 2019 ; Anwar et al. 2021 ; Usman et al. 2022a ). To achieve these lofty goals, adequate financial resources must be mobilized and provided, as well as a new technology framework and expanded capacity building, allowing developing countries and the most vulnerable countries to act under their respective national objectives. The agreement also establishes a more transparent action and support mechanism. All Parties are required by the Paris Agreement to do their best through “nationally determined contributions” (NDCs) and to strengthen these efforts in the coming years (Balsalobre-Lorente et al. 2020 ). It includes obligations that all Parties regularly report on their emissions and implementation activities. A global stock-take will be conducted every five years to review collective progress toward the agreement’s goal and inform the Parties’ future individual actions. The Paris Agreement became available for signature on April 22, 2016, Earth Day, at the United Nations Headquarters in New York. On November 4, 2016, it went into effect 30 days after the so-called double threshold was met (ratification by 55 nations accounting for at least 55% of world emissions). More countries have ratified and continue to ratify the agreement since then, bringing 125 Parties in early 2017. To fully operationalize the Paris Agreement, a work program was initiated in Paris to define mechanisms, processes, and recommendations on a wide range of concerns (Murshed et al. 2021 ). Since 2016, Parties have collaborated in subsidiary bodies (APA, SBSTA, and SBI) and numerous formed entities. The Conference of the Parties functioning as the meeting of the Parties to the Paris Agreement (CMA) convened for the first time in November 2016 in Marrakesh in conjunction with COP22 and made its first two resolutions. The work plan is scheduled to be finished by 2018. Some mitigation and adaptation strategies to reduce the emission in the prospective of Paris agreement are following firstly, a long-term goal of keeping the increase in global average temperature to well below 2 °C above pre-industrial levels, secondly, to aim to limit the rise to 1.5 °C, since this would significantly reduce risks and the impacts of climate change, thirdly, on the need for global emissions to peak as soon as possible, recognizing that this will take longer for developing countries, lastly, to undertake rapid reductions after that under the best available science, to achieve a balance between emissions and removals in the second half of the century. On the other side, some adaptation strategies are; strengthening societies’ ability to deal with the effects of climate change and to continue & expand international assistance for developing nations’ adaptation.
However, anthropogenic activities are currently regarded as most accountable for CC (Murshed et al. 2022 ). Apart from the industrial revolution, other anthropogenic activities include excessive agricultural operations, which further involve the high use of fuel-based mechanization, burning of agricultural residues, burning fossil fuels, deforestation, national and domestic transportation sectors, etc. (Huang et al. 2016 ). Consequently, these anthropogenic activities lead to climatic catastrophes, damaging local and global infrastructure, human health, and total productivity. Energy consumption has mounted GHGs levels concerning warming temperatures as most of the energy production in developing countries comes from fossil fuels (Balsalobre-Lorente et al. 2022 ; Usman et al. 2022b ; Abbass et al. 2021a ; Ishikawa-Ishiwata and Furuya 2022 ).
This review aims to highlight the effects of climate change in a socio-scientific aspect by analyzing the existing literature on various sectorial pieces of evidence globally that influence the environment. Although this review provides a thorough examination of climate change and its severe affected sectors that pose a grave danger for global agriculture, biodiversity, health, economy, forestry, and tourism, and to purpose some practical prophylactic measures and mitigation strategies to be adapted as sound substitutes to survive from climate change (CC) impacts. The societal implications of irregular weather patterns and other effects of climate changes are discussed in detail. Some numerous sustainable mitigation measures and adaptation practices and techniques at the global level are discussed in this review with an in-depth focus on its economic, social, and environmental aspects. Methods of data collection section are included in the supplementary information.
Review methodology
Related study and its objectives.
Today, we live an ordinary life in the beautiful digital, globalized world where climate change has a decisive role. What happens in one country has a massive influence on geographically far apart countries, which points to the current crisis known as COVID-19 (Sarkar et al. 2021 ). The most dangerous disease like COVID-19 has affected the world’s climate changes and economic conditions (Abbass et al. 2022 ; Pirasteh-Anosheh et al. 2021 ). The purpose of the present study is to review the status of research on the subject, which is based on “Global Climate Change Impacts, adaptation, and sustainable mitigation measures” by systematically reviewing past published and unpublished research work. Furthermore, the current study seeks to comment on research on the same topic and suggest future research on the same topic. Specifically, the present study aims: The first one is, organize publications to make them easy and quick to find. Secondly, to explore issues in this area, propose an outline of research for future work. The third aim of the study is to synthesize the previous literature on climate change, various sectors, and their mitigation measurement. Lastly , classify the articles according to the different methods and procedures that have been adopted.
Review methodology for reviewers
This review-based article followed systematic literature review techniques that have proved the literature review as a rigorous framework (Benita 2021 ; Tranfield et al. 2003 ). Moreover, we illustrate in Fig. 1 the search method that we have started for this research. First, finalized the research theme to search literature (Cooper et al. 2018 ). Second, used numerous research databases to search related articles and download from the database (Web of Science, Google Scholar, Scopus Index Journals, Emerald, Elsevier Science Direct, Springer, and Sciverse). We focused on various articles, with research articles, feedback pieces, short notes, debates, and review articles published in scholarly journals. Reports used to search for multiple keywords such as “Climate Change,” “Mitigation and Adaptation,” “Department of Agriculture and Human Health,” “Department of Biodiversity and Forestry,” etc.; in summary, keyword list and full text have been made. Initially, the search for keywords yielded a large amount of literature.

Methodology search for finalized articles for investigations.
Source : constructed by authors
Since 2020, it has been impossible to review all the articles found; some restrictions have been set for the literature exhibition. The study searched 95 articles on a different database mentioned above based on the nature of the study. It excluded 40 irrelevant papers due to copied from a previous search after readings tiles, abstract and full pieces. The criteria for inclusion were: (i) articles focused on “Global Climate Change Impacts, adaptation, and sustainable mitigation measures,” and (ii) the search key terms related to study requirements. The complete procedure yielded 55 articles for our study. We repeat our search on the “Web of Science and Google Scholars” database to enhance the search results and check the referenced articles.
In this study, 55 articles are reviewed systematically and analyzed for research topics and other aspects, such as the methods, contexts, and theories used in these studies. Furthermore, this study analyzes closely related areas to provide unique research opportunities in the future. The study also discussed future direction opportunities and research questions by understanding the research findings climate changes and other affected sectors. The reviewed paper framework analysis process is outlined in Fig. 2 .

Framework of the analysis Process.
Natural disasters and climate change’s socio-economic consequences
Natural and environmental disasters can be highly variable from year to year; some years pass with very few deaths before a significant disaster event claims many lives (Symanski et al. 2021 ). Approximately 60,000 people globally died from natural disasters each year on average over the past decade (Ritchie and Roser 2014 ; Wiranata and Simbolon 2021 ). So, according to the report, around 0.1% of global deaths. Annual variability in the number and share of deaths from natural disasters in recent decades are shown in Fig. 3 . The number of fatalities can be meager—sometimes less than 10,000, and as few as 0.01% of all deaths. But shock events have a devastating impact: the 1983–1985 famine and drought in Ethiopia; the 2004 Indian Ocean earthquake and tsunami; Cyclone Nargis, which struck Myanmar in 2008; and the 2010 Port-au-Prince earthquake in Haiti and now recent example is COVID-19 pandemic (Erman et al. 2021 ). These events pushed global disaster deaths to over 200,000—more than 0.4% of deaths in these years. Low-frequency, high-impact events such as earthquakes and tsunamis are not preventable, but such high losses of human life are. Historical evidence shows that earlier disaster detection, more robust infrastructure, emergency preparedness, and response programmers have substantially reduced disaster deaths worldwide. Low-income is also the most vulnerable to disasters; improving living conditions, facilities, and response services in these areas would be critical in reducing natural disaster deaths in the coming decades.

Global deaths from natural disasters, 1978 to 2020.
Source EMDAT ( 2020 )
The interior regions of the continent are likely to be impacted by rising temperatures (Dimri et al. 2018 ; Goes et al. 2020 ; Mannig et al. 2018 ; Schuurmans 2021 ). Weather patterns change due to the shortage of natural resources (water), increase in glacier melting, and rising mercury are likely to cause extinction to many planted species (Gampe et al. 2016 ; Mihiretu et al. 2021 ; Shaffril et al. 2018 ).On the other hand, the coastal ecosystem is on the verge of devastation (Perera et al. 2018 ; Phillips 2018 ). The temperature rises, insect disease outbreaks, health-related problems, and seasonal and lifestyle changes are persistent, with a strong probability of these patterns continuing in the future (Abbass et al. 2021c ; Hussain et al. 2018 ). At the global level, a shortage of good infrastructure and insufficient adaptive capacity are hammering the most (IPCC 2013 ). In addition to the above concerns, a lack of environmental education and knowledge, outdated consumer behavior, a scarcity of incentives, a lack of legislation, and the government’s lack of commitment to climate change contribute to the general public’s concerns. By 2050, a 2 to 3% rise in mercury and a drastic shift in rainfall patterns may have serious consequences (Huang et al. 2022 ; Gorst et al. 2018 ). Natural and environmental calamities caused huge losses globally, such as decreased agriculture outputs, rehabilitation of the system, and rebuilding necessary technologies (Ali and Erenstein 2017 ; Ramankutty et al. 2018 ; Yu et al. 2021 ) (Table (Table1). 1 ). Furthermore, in the last 3 or 4 years, the world has been plagued by smog-related eye and skin diseases, as well as a rise in road accidents due to poor visibility.
Main natural danger statistics for 1985–2020 at the global level
Source: EM-DAT ( 2020 )
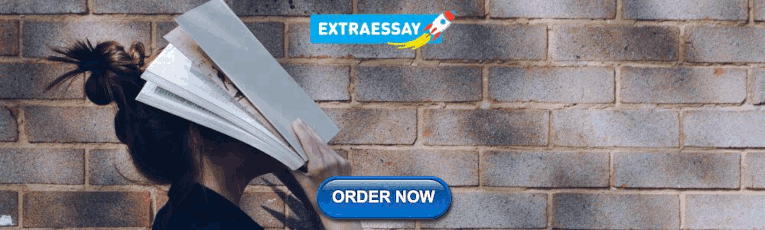
Climate change and agriculture
Global agriculture is the ultimate sector responsible for 30–40% of all greenhouse emissions, which makes it a leading industry predominantly contributing to climate warming and significantly impacted by it (Grieg; Mishra et al. 2021 ; Ortiz et al. 2021 ; Thornton and Lipper 2014 ). Numerous agro-environmental and climatic factors that have a dominant influence on agriculture productivity (Pautasso et al. 2012 ) are significantly impacted in response to precipitation extremes including floods, forest fires, and droughts (Huang 2004 ). Besides, the immense dependency on exhaustible resources also fuels the fire and leads global agriculture to become prone to devastation. Godfray et al. ( 2010 ) mentioned that decline in agriculture challenges the farmer’s quality of life and thus a significant factor to poverty as the food and water supplies are critically impacted by CC (Ortiz et al. 2021 ; Rosenzweig et al. 2014 ). As an essential part of the economic systems, especially in developing countries, agricultural systems affect the overall economy and potentially the well-being of households (Schlenker and Roberts 2009 ). According to the report published by the Intergovernmental Panel on Climate Change (IPCC), atmospheric concentrations of greenhouse gases, i.e., CH 4, CO 2 , and N 2 O, are increased in the air to extraordinary levels over the last few centuries (Usman and Makhdum 2021 ; Stocker et al. 2013 ). Climate change is the composite outcome of two different factors. The first is the natural causes, and the second is the anthropogenic actions (Karami 2012 ). It is also forecasted that the world may experience a typical rise in temperature stretching from 1 to 3.7 °C at the end of this century (Pachauri et al. 2014 ). The world’s crop production is also highly vulnerable to these global temperature-changing trends as raised temperatures will pose severe negative impacts on crop growth (Reidsma et al. 2009 ). Some of the recent modeling about the fate of global agriculture is briefly described below.
Decline in cereal productivity
Crop productivity will also be affected dramatically in the next few decades due to variations in integral abiotic factors such as temperature, solar radiation, precipitation, and CO 2 . These all factors are included in various regulatory instruments like progress and growth, weather-tempted changes, pest invasions (Cammell and Knight 1992 ), accompanying disease snags (Fand et al. 2012 ), water supplies (Panda et al. 2003 ), high prices of agro-products in world’s agriculture industry, and preeminent quantity of fertilizer consumption. Lobell and field ( 2007 ) claimed that from 1962 to 2002, wheat crop output had condensed significantly due to rising temperatures. Therefore, during 1980–2011, the common wheat productivity trends endorsed extreme temperature events confirmed by Gourdji et al. ( 2013 ) around South Asia, South America, and Central Asia. Various other studies (Asseng, Cao, Zhang, and Ludwig 2009 ; Asseng et al. 2013 ; García et al. 2015 ; Ortiz et al. 2021 ) also proved that wheat output is negatively affected by the rising temperatures and also caused adverse effects on biomass productivity (Calderini et al. 1999 ; Sadras and Slafer 2012 ). Hereafter, the rice crop is also influenced by the high temperatures at night. These difficulties will worsen because the temperature will be rising further in the future owing to CC (Tebaldi et al. 2006 ). Another research conducted in China revealed that a 4.6% of rice production per 1 °C has happened connected with the advancement in night temperatures (Tao et al. 2006 ). Moreover, the average night temperature growth also affected rice indicia cultivar’s output pragmatically during 25 years in the Philippines (Peng et al. 2004 ). It is anticipated that the increase in world average temperature will also cause a substantial reduction in yield (Hatfield et al. 2011 ; Lobell and Gourdji 2012 ). In the southern hemisphere, Parry et al. ( 2007 ) noted a rise of 1–4 °C in average daily temperatures at the end of spring season unti the middle of summers, and this raised temperature reduced crop output by cutting down the time length for phenophases eventually reduce the yield (Hatfield and Prueger 2015 ; R. Ortiz 2008 ). Also, world climate models have recommended that humid and subtropical regions expect to be plentiful prey to the upcoming heat strokes (Battisti and Naylor 2009 ). Grain production is the amalgamation of two constituents: the average weight and the grain output/m 2 , however, in crop production. Crop output is mainly accredited to the grain quantity (Araus et al. 2008 ; Gambín and Borrás 2010 ). In the times of grain set, yield resources are mainly strewn between hitherto defined components, i.e., grain usual weight and grain output, which presents a trade-off between them (Gambín and Borrás 2010 ) beside disparities in per grain integration (B. L. Gambín et al. 2006 ). In addition to this, the maize crop is also susceptible to raised temperatures, principally in the flowering stage (Edreira and Otegui 2013 ). In reality, the lower grain number is associated with insufficient acclimatization due to intense photosynthesis and higher respiration and the high-temperature effect on the reproduction phenomena (Edreira and Otegui 2013 ). During the flowering phase, maize visible to heat (30–36 °C) seemed less anthesis-silking intermissions (Edreira et al. 2011 ). Another research by Dupuis and Dumas ( 1990 ) proved that a drop in spikelet when directly visible to high temperatures above 35 °C in vitro pollination. Abnormalities in kernel number claimed by Vega et al. ( 2001 ) is related to conceded plant development during a flowering phase that is linked with the active ear growth phase and categorized as a critical phase for approximation of kernel number during silking (Otegui and Bonhomme 1998 ).
The retort of rice output to high temperature presents disparities in flowering patterns, and seed set lessens and lessens grain weight (Qasim et al. 2020 ; Qasim, Hammad, Maqsood, Tariq, & Chawla). During the daytime, heat directly impacts flowers which lessens the thesis period and quickens the earlier peak flowering (Tao et al. 2006 ). Antagonistic effect of higher daytime temperature d on pollen sprouting proposed seed set decay, whereas, seed set was lengthily reduced than could be explicated by pollen growing at high temperatures 40◦C (Matsui et al. 2001 ).
The decline in wheat output is linked with higher temperatures, confirmed in numerous studies (Semenov 2009 ; Stone and Nicolas 1994 ). High temperatures fast-track the arrangements of plant expansion (Blum et al. 2001 ), diminution photosynthetic process (Salvucci and Crafts‐Brandner 2004 ), and also considerably affect the reproductive operations (Farooq et al. 2011 ).
The destructive impacts of CC induced weather extremes to deteriorate the integrity of crops (Chaudhary et al. 2011 ), e.g., Spartan cold and extreme fog cause falling and discoloration of betel leaves (Rosenzweig et al. 2001 ), giving them a somehow reddish appearance, squeezing of lemon leaves (Pautasso et al. 2012 ), as well as root rot of pineapple, have reported (Vedwan and Rhoades 2001 ). Henceforth, in tackling the disruptive effects of CC, several short-term and long-term management approaches are the crucial need of time (Fig. 4 ). Moreover, various studies (Chaudhary et al. 2011 ; Patz et al. 2005 ; Pautasso et al. 2012 ) have demonstrated adapting trends such as ameliorating crop diversity can yield better adaptability towards CC.

Schematic description of potential impacts of climate change on the agriculture sector and the appropriate mitigation and adaptation measures to overcome its impact.
Climate change impacts on biodiversity
Global biodiversity is among the severe victims of CC because it is the fastest emerging cause of species loss. Studies demonstrated that the massive scale species dynamics are considerably associated with diverse climatic events (Abraham and Chain 1988 ; Manes et al. 2021 ; A. M. D. Ortiz et al. 2021 ). Both the pace and magnitude of CC are altering the compatible habitat ranges for living entities of marine, freshwater, and terrestrial regions. Alterations in general climate regimes influence the integrity of ecosystems in numerous ways, such as variation in the relative abundance of species, range shifts, changes in activity timing, and microhabitat use (Bates et al. 2014 ). The geographic distribution of any species often depends upon its ability to tolerate environmental stresses, biological interactions, and dispersal constraints. Hence, instead of the CC, the local species must only accept, adapt, move, or face extinction (Berg et al. 2010 ). So, the best performer species have a better survival capacity for adjusting to new ecosystems or a decreased perseverance to survive where they are already situated (Bates et al. 2014 ). An important aspect here is the inadequate habitat connectivity and access to microclimates, also crucial in raising the exposure to climate warming and extreme heatwave episodes. For example, the carbon sequestration rates are undergoing fluctuations due to climate-driven expansion in the range of global mangroves (Cavanaugh et al. 2014 ).
Similarly, the loss of kelp-forest ecosystems in various regions and its occupancy by the seaweed turfs has set the track for elevated herbivory by the high influx of tropical fish populations. Not only this, the increased water temperatures have exacerbated the conditions far away from the physiological tolerance level of the kelp communities (Vergés et al. 2016 ; Wernberg et al. 2016 ). Another pertinent danger is the devastation of keystone species, which even has more pervasive effects on the entire communities in that habitat (Zarnetske et al. 2012 ). It is particularly important as CC does not specify specific populations or communities. Eventually, this CC-induced redistribution of species may deteriorate carbon storage and the net ecosystem productivity (Weed et al. 2013 ). Among the typical disruptions, the prominent ones include impacts on marine and terrestrial productivity, marine community assembly, and the extended invasion of toxic cyanobacteria bloom (Fossheim et al. 2015 ).
The CC-impacted species extinction is widely reported in the literature (Beesley et al. 2019 ; Urban 2015 ), and the predictions of demise until the twenty-first century are dreadful (Abbass et al. 2019 ; Pereira et al. 2013 ). In a few cases, northward shifting of species may not be formidable as it allows mountain-dwelling species to find optimum climates. However, the migrant species may be trapped in isolated and incompatible habitats due to losing topography and range (Dullinger et al. 2012 ). For example, a study indicated that the American pika has been extirpated or intensely diminished in some regions, primarily attributed to the CC-impacted extinction or at least local extirpation (Stewart et al. 2015 ). Besides, the anticipation of persistent responses to the impacts of CC often requires data records of several decades to rigorously analyze the critical pre and post CC patterns at species and ecosystem levels (Manes et al. 2021 ; Testa et al. 2018 ).
Nonetheless, the availability of such long-term data records is rare; hence, attempts are needed to focus on these profound aspects. Biodiversity is also vulnerable to the other associated impacts of CC, such as rising temperatures, droughts, and certain invasive pest species. For instance, a study revealed the changes in the composition of plankton communities attributed to rising temperatures. Henceforth, alterations in such aquatic producer communities, i.e., diatoms and calcareous plants, can ultimately lead to variation in the recycling of biological carbon. Moreover, such changes are characterized as a potential contributor to CO 2 differences between the Pleistocene glacial and interglacial periods (Kohfeld et al. 2005 ).
Climate change implications on human health
It is an understood corporality that human health is a significant victim of CC (Costello et al. 2009 ). According to the WHO, CC might be responsible for 250,000 additional deaths per year during 2030–2050 (Watts et al. 2015 ). These deaths are attributed to extreme weather-induced mortality and morbidity and the global expansion of vector-borne diseases (Lemery et al. 2021; Yang and Usman 2021 ; Meierrieks 2021 ; UNEP 2017 ). Here, some of the emerging health issues pertinent to this global problem are briefly described.
Climate change and antimicrobial resistance with corresponding economic costs
Antimicrobial resistance (AMR) is an up-surging complex global health challenge (Garner et al. 2019 ; Lemery et al. 2021 ). Health professionals across the globe are extremely worried due to this phenomenon that has critical potential to reverse almost all the progress that has been achieved so far in the health discipline (Gosling and Arnell 2016 ). A massive amount of antibiotics is produced by many pharmaceutical industries worldwide, and the pathogenic microorganisms are gradually developing resistance to them, which can be comprehended how strongly this aspect can shake the foundations of national and global economies (UNEP 2017 ). This statement is supported by the fact that AMR is not developing in a particular region or country. Instead, it is flourishing in every continent of the world (WHO 2018 ). This plague is heavily pushing humanity to the post-antibiotic era, in which currently antibiotic-susceptible pathogens will once again lead to certain endemics and pandemics after being resistant(WHO 2018 ). Undesirably, if this statement would become a factuality, there might emerge certain risks in undertaking sophisticated interventions such as chemotherapy, joint replacement cases, and organ transplantation (Su et al. 2018 ). Presently, the amplification of drug resistance cases has made common illnesses like pneumonia, post-surgical infections, HIV/AIDS, tuberculosis, malaria, etc., too difficult and costly to be treated or cure well (WHO 2018 ). From a simple example, it can be assumed how easily antibiotic-resistant strains can be transmitted from one person to another and ultimately travel across the boundaries (Berendonk et al. 2015 ). Talking about the second- and third-generation classes of antibiotics, e.g., most renowned generations of cephalosporin antibiotics that are more expensive, broad-spectrum, more toxic, and usually require more extended periods whenever prescribed to patients (Lemery et al. 2021 ; Pärnänen et al. 2019 ). This scenario has also revealed that the abundance of resistant strains of pathogens was also higher in the Southern part (WHO 2018 ). As southern parts are generally warmer than their counterparts, it is evident from this example how CC-induced global warming can augment the spread of antibiotic-resistant strains within the biosphere, eventually putting additional economic burden in the face of developing new and costlier antibiotics. The ARG exchange to susceptible bacteria through one of the potential mechanisms, transformation, transduction, and conjugation; Selection pressure can be caused by certain antibiotics, metals or pesticides, etc., as shown in Fig. 5 .

A typical interaction between the susceptible and resistant strains.
Source: Elsayed et al. ( 2021 ); Karkman et al. ( 2018 )
Certain studies highlighted that conventional urban wastewater treatment plants are typical hotspots where most bacterial strains exchange genetic material through horizontal gene transfer (Fig. 5 ). Although at present, the extent of risks associated with the antibiotic resistance found in wastewater is complicated; environmental scientists and engineers have particular concerns about the potential impacts of these antibiotic resistance genes on human health (Ashbolt 2015 ). At most undesirable and worst case, these antibiotic-resistant genes containing bacteria can make their way to enter into the environment (Pruden et al. 2013 ), irrigation water used for crops and public water supplies and ultimately become a part of food chains and food webs (Ma et al. 2019 ; D. Wu et al. 2019 ). This problem has been reported manifold in several countries (Hendriksen et al. 2019 ), where wastewater as a means of irrigated water is quite common.
Climate change and vector borne-diseases
Temperature is a fundamental factor for the sustenance of living entities regardless of an ecosystem. So, a specific living being, especially a pathogen, requires a sophisticated temperature range to exist on earth. The second essential component of CC is precipitation, which also impacts numerous infectious agents’ transport and dissemination patterns. Global rising temperature is a significant cause of many species extinction. On the one hand, this changing environmental temperature may be causing species extinction, and on the other, this warming temperature might favor the thriving of some new organisms. Here, it was evident that some pathogens may also upraise once non-evident or reported (Patz et al. 2000 ). This concept can be exemplified through certain pathogenic strains of microorganisms that how the likelihood of various diseases increases in response to climate warming-induced environmental changes (Table (Table2 2 ).
Examples of how various environmental changes affect various infectious diseases in humans
Source: Aron and Patz ( 2001 )
A recent example is an outburst of coronavirus (COVID-19) in the Republic of China, causing pneumonia and severe acute respiratory complications (Cui et al. 2021 ; Song et al. 2021 ). The large family of viruses is harbored in numerous animals, bats, and snakes in particular (livescience.com) with the subsequent transfer into human beings. Hence, it is worth noting that the thriving of numerous vectors involved in spreading various diseases is influenced by Climate change (Ogden 2018 ; Santos et al. 2021 ).
Psychological impacts of climate change
Climate change (CC) is responsible for the rapid dissemination and exaggeration of certain epidemics and pandemics. In addition to the vast apparent impacts of climate change on health, forestry, agriculture, etc., it may also have psychological implications on vulnerable societies. It can be exemplified through the recent outburst of (COVID-19) in various countries around the world (Pal 2021 ). Besides, the victims of this viral infection have made healthy beings scarier and terrified. In the wake of such epidemics, people with common colds or fever are also frightened and must pass specific regulatory protocols. Living in such situations continuously terrifies the public and makes the stress familiar, which eventually makes them psychologically weak (npr.org).
CC boosts the extent of anxiety, distress, and other issues in public, pushing them to develop various mental-related problems. Besides, frequent exposure to extreme climatic catastrophes such as geological disasters also imprints post-traumatic disorder, and their ubiquitous occurrence paves the way to developing chronic psychological dysfunction. Moreover, repetitive listening from media also causes an increase in the person’s stress level (Association 2020 ). Similarly, communities living in flood-prone areas constantly live in extreme fear of drowning and die by floods. In addition to human lives, the flood-induced destruction of physical infrastructure is a specific reason for putting pressure on these communities (Ogden 2018 ). For instance, Ogden ( 2018 ) comprehensively denoted that Katrina’s Hurricane augmented the mental health issues in the victim communities.
Climate change impacts on the forestry sector
Forests are the global regulators of the world’s climate (FAO 2018 ) and have an indispensable role in regulating global carbon and nitrogen cycles (Rehman et al. 2021 ; Reichstein and Carvalhais 2019 ). Hence, disturbances in forest ecology affect the micro and macro-climates (Ellison et al. 2017 ). Climate warming, in return, has profound impacts on the growth and productivity of transboundary forests by influencing the temperature and precipitation patterns, etc. As CC induces specific changes in the typical structure and functions of ecosystems (Zhang et al. 2017 ) as well impacts forest health, climate change also has several devastating consequences such as forest fires, droughts, pest outbreaks (EPA 2018 ), and last but not the least is the livelihoods of forest-dependent communities. The rising frequency and intensity of another CC product, i.e., droughts, pose plenty of challenges to the well-being of global forests (Diffenbaugh et al. 2017 ), which is further projected to increase soon (Hartmann et al. 2018 ; Lehner et al. 2017 ; Rehman et al. 2021 ). Hence, CC induces storms, with more significant impacts also put extra pressure on the survival of the global forests (Martínez-Alvarado et al. 2018 ), significantly since their influences are augmented during higher winter precipitations with corresponding wetter soils causing weak root anchorage of trees (Brázdil et al. 2018 ). Surging temperature regimes causes alterations in usual precipitation patterns, which is a significant hurdle for the survival of temperate forests (Allen et al. 2010 ; Flannigan et al. 2013 ), letting them encounter severe stress and disturbances which adversely affects the local tree species (Hubbart et al. 2016 ; Millar and Stephenson 2015 ; Rehman et al. 2021 ).
Climate change impacts on forest-dependent communities
Forests are the fundamental livelihood resource for about 1.6 billion people worldwide; out of them, 350 million are distinguished with relatively higher reliance (Bank 2008 ). Agro-forestry-dependent communities comprise 1.2 billion, and 60 million indigenous people solely rely on forests and their products to sustain their lives (Sunderlin et al. 2005 ). For example, in the entire African continent, more than 2/3rd of inhabitants depend on forest resources and woodlands for their alimonies, e.g., food, fuelwood and grazing (Wasiq and Ahmad 2004 ). The livings of these people are more intensely affected by the climatic disruptions making their lives harder (Brown et al. 2014 ). On the one hand, forest communities are incredibly vulnerable to CC due to their livelihoods, cultural and spiritual ties as well as socio-ecological connections, and on the other, they are not familiar with the term “climate change.” (Rahman and Alam 2016 ). Among the destructive impacts of temperature and rainfall, disruption of the agroforestry crops with resultant downscale growth and yield (Macchi et al. 2008 ). Cruz ( 2015 ) ascribed that forest-dependent smallholder farmers in the Philippines face the enigma of delayed fruiting, more severe damages by insect and pest incidences due to unfavorable temperature regimes, and changed rainfall patterns.
Among these series of challenges to forest communities, their well-being is also distinctly vulnerable to CC. Though the detailed climate change impacts on human health have been comprehensively mentioned in the previous section, some studies have listed a few more devastating effects on the prosperity of forest-dependent communities. For instance, the Himalayan people have been experiencing frequent skin-borne diseases such as malaria and other skin diseases due to increasing mosquitoes, wild boar as well, and new wasps species, particularly in higher altitudes that were almost non-existent before last 5–10 years (Xu et al. 2008 ). Similarly, people living at high altitudes in Bangladesh have experienced frequent mosquito-borne calamities (Fardous; Sharma 2012 ). In addition, the pace of other waterborne diseases such as infectious diarrhea, cholera, pathogenic induced abdominal complications and dengue has also been boosted in other distinguished regions of Bangladesh (Cell 2009 ; Gunter et al. 2008 ).
Pest outbreak
Upscaling hotter climate may positively affect the mobile organisms with shorter generation times because they can scurry from harsh conditions than the immobile species (Fettig et al. 2013 ; Schoene and Bernier 2012 ) and are also relatively more capable of adapting to new environments (Jactel et al. 2019 ). It reveals that insects adapt quickly to global warming due to their mobility advantages. Due to past outbreaks, the trees (forests) are relatively more susceptible victims (Kurz et al. 2008 ). Before CC, the influence of factors mentioned earlier, i.e., droughts and storms, was existent and made the forests susceptible to insect pest interventions; however, the global forests remain steadfast, assiduous, and green (Jactel et al. 2019 ). The typical reasons could be the insect herbivores were regulated by several tree defenses and pressures of predation (Wilkinson and Sherratt 2016 ). As climate greatly influences these phenomena, the global forests cannot be so sedulous against such challenges (Jactel et al. 2019 ). Table Table3 3 demonstrates some of the particular considerations with practical examples that are essential while mitigating the impacts of CC in the forestry sector.
Essential considerations while mitigating the climate change impacts on the forestry sector
Source : Fischer ( 2019 )
Climate change impacts on tourism
Tourism is a commercial activity that has roots in multi-dimensions and an efficient tool with adequate job generation potential, revenue creation, earning of spectacular foreign exchange, enhancement in cross-cultural promulgation and cooperation, a business tool for entrepreneurs and eventually for the country’s national development (Arshad et al. 2018 ; Scott 2021 ). Among a plethora of other disciplines, the tourism industry is also a distinct victim of climate warming (Gössling et al. 2012 ; Hall et al. 2015 ) as the climate is among the essential resources that enable tourism in particular regions as most preferred locations. Different places at different times of the year attract tourists both within and across the countries depending upon the feasibility and compatibility of particular weather patterns. Hence, the massive variations in these weather patterns resulting from CC will eventually lead to monumental challenges to the local economy in that specific area’s particular and national economy (Bujosa et al. 2015 ). For instance, the Intergovernmental Panel on Climate Change (IPCC) report demonstrated that the global tourism industry had faced a considerable decline in the duration of ski season, including the loss of some ski areas and the dramatic shifts in tourist destinations’ climate warming.
Furthermore, different studies (Neuvonen et al. 2015 ; Scott et al. 2004 ) indicated that various currently perfect tourist spots, e.g., coastal areas, splendid islands, and ski resorts, will suffer consequences of CC. It is also worth noting that the quality and potential of administrative management potential to cope with the influence of CC on the tourism industry is of crucial significance, which renders specific strengths of resiliency to numerous destinations to withstand against it (Füssel and Hildén 2014 ). Similarly, in the partial or complete absence of adequate socio-economic and socio-political capital, the high-demanding tourist sites scurry towards the verge of vulnerability. The susceptibility of tourism is based on different components such as the extent of exposure, sensitivity, life-supporting sectors, and capacity assessment factors (Füssel and Hildén 2014 ). It is obvious corporality that sectors such as health, food, ecosystems, human habitat, infrastructure, water availability, and the accessibility of a particular region are prone to CC. Henceforth, the sensitivity of these critical sectors to CC and, in return, the adaptive measures are a hallmark in determining the composite vulnerability of climate warming (Ionescu et al. 2009 ).
Moreover, the dependence on imported food items, poor hygienic conditions, and inadequate health professionals are dominant aspects affecting the local terrestrial and aquatic biodiversity. Meanwhile, the greater dependency on ecosystem services and its products also makes a destination more fragile to become a prey of CC (Rizvi et al. 2015 ). Some significant non-climatic factors are important indicators of a particular ecosystem’s typical health and functioning, e.g., resource richness and abundance portray the picture of ecosystem stability. Similarly, the species abundance is also a productive tool that ensures that the ecosystem has a higher buffering capacity, which is terrific in terms of resiliency (Roscher et al. 2013 ).
Climate change impacts on the economic sector
Climate plays a significant role in overall productivity and economic growth. Due to its increasingly global existence and its effect on economic growth, CC has become one of the major concerns of both local and international environmental policymakers (Ferreira et al. 2020 ; Gleditsch 2021 ; Abbass et al. 2021b ; Lamperti et al. 2021 ). The adverse effects of CC on the overall productivity factor of the agricultural sector are therefore significant for understanding the creation of local adaptation policies and the composition of productive climate policy contracts. Previous studies on CC in the world have already forecasted its effects on the agricultural sector. Researchers have found that global CC will impact the agricultural sector in different world regions. The study of the impacts of CC on various agrarian activities in other demographic areas and the development of relative strategies to respond to effects has become a focal point for researchers (Chandioet al. 2020 ; Gleditsch 2021 ; Mosavi et al. 2020 ).
With the rapid growth of global warming since the 1980s, the temperature has started increasing globally, which resulted in the incredible transformation of rain and evaporation in the countries. The agricultural development of many countries has been reliant, delicate, and susceptible to CC for a long time, and it is on the development of agriculture total factor productivity (ATFP) influence different crops and yields of farmers (Alhassan 2021 ; Wu 2020 ).
Food security and natural disasters are increasing rapidly in the world. Several major climatic/natural disasters have impacted local crop production in the countries concerned. The effects of these natural disasters have been poorly controlled by the development of the economies and populations and may affect human life as well. One example is China, which is among the world’s most affected countries, vulnerable to natural disasters due to its large population, harsh environmental conditions, rapid CC, low environmental stability, and disaster power. According to the January 2016 statistical survey, China experienced an economic loss of 298.3 billion Yuan, and about 137 million Chinese people were severely affected by various natural disasters (Xie et al. 2018 ).
Mitigation and adaptation strategies of climate changes
Adaptation and mitigation are the crucial factors to address the response to CC (Jahanzad et al. 2020 ). Researchers define mitigation on climate changes, and on the other hand, adaptation directly impacts climate changes like floods. To some extent, mitigation reduces or moderates greenhouse gas emission, and it becomes a critical issue both economically and environmentally (Botzen et al. 2021 ; Jahanzad et al. 2020 ; Kongsager 2018 ; Smit et al. 2000 ; Vale et al. 2021 ; Usman et al. 2021 ; Verheyen 2005 ).
Researchers have deep concern about the adaptation and mitigation methodologies in sectoral and geographical contexts. Agriculture, industry, forestry, transport, and land use are the main sectors to adapt and mitigate policies(Kärkkäinen et al. 2020 ; Waheed et al. 2021 ). Adaptation and mitigation require particular concern both at the national and international levels. The world has faced a significant problem of climate change in the last decades, and adaptation to these effects is compulsory for economic and social development. To adapt and mitigate against CC, one should develop policies and strategies at the international level (Hussain et al. 2020 ). Figure 6 depicts the list of current studies on sectoral impacts of CC with adaptation and mitigation measures globally.

Sectoral impacts of climate change with adaptation and mitigation measures.
Conclusion and future perspectives
Specific socio-agricultural, socio-economic, and physical systems are the cornerstone of psychological well-being, and the alteration in these systems by CC will have disastrous impacts. Climate variability, alongside other anthropogenic and natural stressors, influences human and environmental health sustainability. Food security is another concerning scenario that may lead to compromised food quality, higher food prices, and inadequate food distribution systems. Global forests are challenged by different climatic factors such as storms, droughts, flash floods, and intense precipitation. On the other hand, their anthropogenic wiping is aggrandizing their existence. Undoubtedly, the vulnerability scale of the world’s regions differs; however, appropriate mitigation and adaptation measures can aid the decision-making bodies in developing effective policies to tackle its impacts. Presently, modern life on earth has tailored to consistent climatic patterns, and accordingly, adapting to such considerable variations is of paramount importance. Because the faster changes in climate will make it harder to survive and adjust, this globally-raising enigma calls for immediate attention at every scale ranging from elementary community level to international level. Still, much effort, research, and dedication are required, which is the most critical time. Some policy implications can help us to mitigate the consequences of climate change, especially the most affected sectors like the agriculture sector;
Warming might lengthen the season in frost-prone growing regions (temperate and arctic zones), allowing for longer-maturing seasonal cultivars with better yields (Pfadenhauer 2020 ; Bonacci 2019 ). Extending the planting season may allow additional crops each year; when warming leads to frequent warmer months highs over critical thresholds, a split season with a brief summer fallow may be conceivable for short-period crops such as wheat barley, cereals, and many other vegetable crops. The capacity to prolong the planting season in tropical and subtropical places where the harvest season is constrained by precipitation or agriculture farming occurs after the year may be more limited and dependent on how precipitation patterns vary (Wu et al. 2017 ).
The genetic component is comprehensive for many yields, but it is restricted like kiwi fruit for a few. Ali et al. ( 2017 ) investigated how new crops will react to climatic changes (also stated in Mall et al. 2017 ). Hot temperature, drought, insect resistance; salt tolerance; and overall crop production and product quality increases would all be advantageous (Akkari 2016 ). Genetic mapping and engineering can introduce a greater spectrum of features. The adoption of genetically altered cultivars has been slowed, particularly in the early forecasts owing to the complexity in ensuring features are expediently expressed throughout the entire plant, customer concerns, economic profitability, and regulatory impediments (Wirehn 2018 ; Davidson et al. 2016 ).
To get the full benefit of the CO 2 would certainly require additional nitrogen and other fertilizers. Nitrogen not consumed by the plants may be excreted into groundwater, discharged into water surface, or emitted from the land, soil nitrous oxide when large doses of fertilizer are sprayed. Increased nitrogen levels in groundwater sources have been related to human chronic illnesses and impact marine ecosystems. Cultivation, grain drying, and other field activities have all been examined in depth in the studies (Barua et al. 2018 ).
- The technological and socio-economic adaptation
The policy consequence of the causative conclusion is that as a source of alternative energy, biofuel production is one of the routes that explain oil price volatility separate from international macroeconomic factors. Even though biofuel production has just begun in a few sample nations, there is still a tremendous worldwide need for feedstock to satisfy industrial expansion in China and the USA, which explains the food price relationship to the global oil price. Essentially, oil-exporting countries may create incentives in their economies to increase food production. It may accomplish by giving farmers financing, seedlings, fertilizers, and farming equipment. Because of the declining global oil price and, as a result, their earnings from oil export, oil-producing nations may be unable to subsidize food imports even in the near term. As a result, these countries can boost the agricultural value chain for export. It may be accomplished through R&D and adding value to their food products to increase income by correcting exchange rate misalignment and adverse trade terms. These nations may also diversify their economies away from oil, as dependence on oil exports alone is no longer economically viable given the extreme volatility of global oil prices. Finally, resource-rich and oil-exporting countries can convert to non-food renewable energy sources such as solar, hydro, coal, wind, wave, and tidal energy. By doing so, both world food and oil supplies would be maintained rather than harmed.
IRENA’s modeling work shows that, if a comprehensive policy framework is in place, efforts toward decarbonizing the energy future will benefit economic activity, jobs (outweighing losses in the fossil fuel industry), and welfare. Countries with weak domestic supply chains and a large reliance on fossil fuel income, in particular, must undertake structural reforms to capitalize on the opportunities inherent in the energy transition. Governments continue to give major policy assistance to extract fossil fuels, including tax incentives, financing, direct infrastructure expenditures, exemptions from environmental regulations, and other measures. The majority of major oil and gas producing countries intend to increase output. Some countries intend to cut coal output, while others plan to maintain or expand it. While some nations are beginning to explore and execute policies aimed at a just and equitable transition away from fossil fuel production, these efforts have yet to impact major producing countries’ plans and goals. Verifiable and comparable data on fossil fuel output and assistance from governments and industries are critical to closing the production gap. Governments could increase openness by declaring their production intentions in their climate obligations under the Paris Agreement.
It is firmly believed that achieving the Paris Agreement commitments is doubtlful without undergoing renewable energy transition across the globe (Murshed 2020 ; Zhao et al. 2022 ). Policy instruments play the most important role in determining the degree of investment in renewable energy technology. This study examines the efficacy of various policy strategies in the renewable energy industry of multiple nations. Although its impact is more visible in established renewable energy markets, a renewable portfolio standard is also a useful policy instrument. The cost of producing renewable energy is still greater than other traditional energy sources. Furthermore, government incentives in the R&D sector can foster innovation in this field, resulting in cost reductions in the renewable energy industry. These nations may export their technologies and share their policy experiences by forming networks among their renewable energy-focused organizations. All policy measures aim to reduce production costs while increasing the proportion of renewables to a country’s energy system. Meanwhile, long-term contracts with renewable energy providers, government commitment and control, and the establishment of long-term goals can assist developing nations in deploying renewable energy technology in their energy sector.
Author contribution
KA: Writing the original manuscript, data collection, data analysis, Study design, Formal analysis, Visualization, Revised draft, Writing-review, and editing. MZQ: Writing the original manuscript, data collection, data analysis, Writing-review, and editing. HS: Contribution to the contextualization of the theme, Conceptualization, Validation, Supervision, literature review, Revised drapt, and writing review and editing. MM: Writing review and editing, compiling the literature review, language editing. HM: Writing review and editing, compiling the literature review, language editing. IY: Contribution to the contextualization of the theme, literature review, and writing review and editing.
Availability of data and material
Declarations.
Not applicable.
The authors declare no competing interests.
Publisher's Note
Springer Nature remains neutral with regard to jurisdictional claims in published maps and institutional affiliations.
Contributor Information
Kashif Abbass, Email: nc.ude.tsujn@ssabbafihsak .
Muhammad Zeeshan Qasim, Email: moc.kooltuo@888misaqnahseez .
Huaming Song, Email: nc.ude.tsujn@gnimauh .
Muntasir Murshed, Email: [email protected] .
Haider Mahmood, Email: moc.liamtoh@doomhamrediah .
Ijaz Younis, Email: nc.ude.tsujn@sinuoyzaji .
- Abbass K, Begum H, Alam ASA, Awang AH, Abdelsalam MK, Egdair IMM, Wahid R (2022) Fresh Insight through a Keynesian Theory Approach to Investigate the Economic Impact of the COVID-19 Pandemic in Pakistan. Sustain 14(3):1054
- Abbass K, Niazi AAK, Qazi TF, Basit A, Song H (2021a) The aftermath of COVID-19 pandemic period: barriers in implementation of social distancing at workplace. Library Hi Tech
- Abbass K, Song H, Khan F, Begum H, Asif M (2021b) Fresh insight through the VAR approach to investigate the effects of fiscal policy on environmental pollution in Pakistan. Environ Scie Poll Res 1–14 [ PubMed ]
- Abbass K, Song H, Shah SM, Aziz B. Determinants of Stock Return for Non-Financial Sector: Evidence from Energy Sector of Pakistan. J Bus Fin Aff. 2019; 8 (370):2167–0234. [ Google Scholar ]
- Abbass K, Tanveer A, Huaming S, Khatiya AA (2021c) Impact of financial resources utilization on firm performance: a case of SMEs working in Pakistan
- Abraham E, Chain E. An enzyme from bacteria able to destroy penicillin. 1940. Rev Infect Dis. 1988; 10 (4):677. [ PubMed ] [ Google Scholar ]
- Adger WN, Arnell NW, Tompkins EL. Successful adaptation to climate change across scales. Glob Environ Chang. 2005; 15 (2):77–86. doi: 10.1016/j.gloenvcha.2004.12.005. [ CrossRef ] [ Google Scholar ]
- Akkari C, Bryant CR. The co-construction approach as approach to developing adaptation strategies in the face of climate change and variability: A conceptual framework. Agricultural Research. 2016; 5 (2):162–173. doi: 10.1007/s40003-016-0208-8. [ CrossRef ] [ Google Scholar ]
- Alhassan H (2021) The effect of agricultural total factor productivity on environmental degradation in sub-Saharan Africa. Sci Afr 12:e00740
- Ali A, Erenstein O. Assessing farmer use of climate change adaptation practices and impacts on food security and poverty in Pakistan. Clim Risk Manag. 2017; 16 :183–194. doi: 10.1016/j.crm.2016.12.001. [ CrossRef ] [ Google Scholar ]
- Allen CD, Macalady AK, Chenchouni H, Bachelet D, McDowell N, Vennetier M, Hogg ET. A global overview of drought and heat-induced tree mortality reveals emerging climate change risks for forests. For Ecol Manag. 2010; 259 (4):660–684. doi: 10.1016/j.foreco.2009.09.001. [ CrossRef ] [ Google Scholar ]
- Anwar A, Sinha A, Sharif A, Siddique M, Irshad S, Anwar W, Malik S (2021) The nexus between urbanization, renewable energy consumption, financial development, and CO2 emissions: evidence from selected Asian countries. Environ Dev Sust. 10.1007/s10668-021-01716-2
- Araus JL, Slafer GA, Royo C, Serret MD. Breeding for yield potential and stress adaptation in cereals. Crit Rev Plant Sci. 2008; 27 (6):377–412. doi: 10.1080/07352680802467736. [ CrossRef ] [ Google Scholar ]
- Aron JL, Patz J (2001) Ecosystem change and public health: a global perspective: JHU Press
- Arshad MI, Iqbal MA, Shahbaz M. Pakistan tourism industry and challenges: a review. Asia Pacific Journal of Tourism Research. 2018; 23 (2):121–132. doi: 10.1080/10941665.2017.1410192. [ CrossRef ] [ Google Scholar ]
- Ashbolt NJ. Microbial contamination of drinking water and human health from community water systems. Current Environmental Health Reports. 2015; 2 (1):95–106. doi: 10.1007/s40572-014-0037-5. [ PMC free article ] [ PubMed ] [ CrossRef ] [ Google Scholar ]
- Asseng S, Cao W, Zhang W, Ludwig F (2009) Crop physiology, modelling and climate change: impact and adaptation strategies. Crop Physiol 511–543
- Asseng S, Ewert F, Rosenzweig C, Jones JW, Hatfield JL, Ruane AC, Cammarano D. Uncertainty in simulating wheat yields under climate change. Nat Clim Chang. 2013; 3 (9):827–832. doi: 10.1038/nclimate1916. [ CrossRef ] [ Google Scholar ]
- Association A (2020) Climate change is threatening mental health, American Psychological Association, “Kirsten Weir, . from < https://www.apa.org/monitor/2016/07-08/climate-change >, Accessed on 26 Jan 2020.
- Ayers J, Huq S, Wright H, Faisal A, Hussain S. Mainstreaming climate change adaptation into development in Bangladesh. Clim Dev. 2014; 6 :293–305. doi: 10.1080/17565529.2014.977761. [ CrossRef ] [ Google Scholar ]
- Balsalobre-Lorente D, Driha OM, Bekun FV, Sinha A, Adedoyin FF (2020) Consequences of COVID-19 on the social isolation of the Chinese economy: accounting for the role of reduction in carbon emissions. Air Qual Atmos Health 13(12):1439–1451
- Balsalobre-Lorente D, Ibáñez-Luzón L, Usman M, Shahbaz M. The environmental Kuznets curve, based on the economic complexity, and the pollution haven hypothesis in PIIGS countries. Renew Energy. 2022; 185 :1441–1455. doi: 10.1016/j.renene.2021.10.059. [ CrossRef ] [ Google Scholar ]
- Bank W (2008) Forests sourcebook: practical guidance for sustaining forests in development cooperation: World Bank
- Barua S, Valenzuela E (2018) Climate change impacts on global agricultural trade patterns: evidence from the past 50 years. In Proceedings of the Sixth International Conference on Sustainable Development (pp. 26–28)
- Bates AE, Pecl GT, Frusher S, Hobday AJ, Wernberg T, Smale DA, Colwell RK. Defining and observing stages of climate-mediated range shifts in marine systems. Glob Environ Chang. 2014; 26 :27–38. doi: 10.1016/j.gloenvcha.2014.03.009. [ CrossRef ] [ Google Scholar ]
- Battisti DS, Naylor RL. Historical warnings of future food insecurity with unprecedented seasonal heat. Science. 2009; 323 (5911):240–244. doi: 10.1126/science.1164363. [ PubMed ] [ CrossRef ] [ Google Scholar ]
- Beesley L, Close PG, Gwinn DC, Long M, Moroz M, Koster WM, Storer T. Flow-mediated movement of freshwater catfish, Tandanus bostocki, in a regulated semi-urban river, to inform environmental water releases. Ecol Freshw Fish. 2019; 28 (3):434–445. doi: 10.1111/eff.12466. [ CrossRef ] [ Google Scholar ]
- Benita F (2021) Human mobility behavior in COVID-19: A systematic literature review and bibliometric analysis. Sustain Cities Soc 70:102916 [ PMC free article ] [ PubMed ]
- Berendonk TU, Manaia CM, Merlin C, Fatta-Kassinos D, Cytryn E, Walsh F, Pons M-N. Tackling antibiotic resistance: the environmental framework. Nat Rev Microbiol. 2015; 13 (5):310–317. doi: 10.1038/nrmicro3439. [ PubMed ] [ CrossRef ] [ Google Scholar ]
- Berg MP, Kiers ET, Driessen G, Van DerHEIJDEN M, Kooi BW, Kuenen F, Ellers J. Adapt or disperse: understanding species persistence in a changing world. Glob Change Biol. 2010; 16 (2):587–598. doi: 10.1111/j.1365-2486.2009.02014.x. [ CrossRef ] [ Google Scholar ]
- Blum A, Klueva N, Nguyen H. Wheat cellular thermotolerance is related to yield under heat stress. Euphytica. 2001; 117 (2):117–123. doi: 10.1023/A:1004083305905. [ CrossRef ] [ Google Scholar ]
- Bonacci O. Air temperature and precipitation analyses on a small Mediterranean island: the case of the remote island of Lastovo (Adriatic Sea, Croatia) Acta Hydrotechnica. 2019; 32 (57):135–150. doi: 10.15292/acta.hydro.2019.10. [ CrossRef ] [ Google Scholar ]
- Botzen W, Duijndam S, van Beukering P (2021) Lessons for climate policy from behavioral biases towards COVID-19 and climate change risks. World Dev 137:105214 [ PMC free article ] [ PubMed ]
- Brázdil R, Stucki P, Szabó P, Řezníčková L, Dolák L, Dobrovolný P, Suchánková S. Windstorms and forest disturbances in the Czech Lands: 1801–2015. Agric for Meteorol. 2018; 250 :47–63. doi: 10.1016/j.agrformet.2017.11.036. [ CrossRef ] [ Google Scholar ]
- Brown HCP, Smit B, Somorin OA, Sonwa DJ, Nkem JN. Climate change and forest communities: prospects for building institutional adaptive capacity in the Congo Basin forests. Ambio. 2014; 43 (6):759–769. doi: 10.1007/s13280-014-0493-z. [ PMC free article ] [ PubMed ] [ CrossRef ] [ Google Scholar ]
- Bujosa A, Riera A, Torres CM. Valuing tourism demand attributes to guide climate change adaptation measures efficiently: the case of the Spanish domestic travel market. Tour Manage. 2015; 47 :233–239. doi: 10.1016/j.tourman.2014.09.023. [ CrossRef ] [ Google Scholar ]
- Calderini D, Abeledo L, Savin R, Slafer GA. Effect of temperature and carpel size during pre-anthesis on potential grain weight in wheat. J Agric Sci. 1999; 132 (4):453–459. doi: 10.1017/S0021859699006504. [ CrossRef ] [ Google Scholar ]
- Cammell M, Knight J. Effects of climatic change on the population dynamics of crop pests. Adv Ecol Res. 1992; 22 :117–162. doi: 10.1016/S0065-2504(08)60135-X. [ CrossRef ] [ Google Scholar ]
- Cavanaugh KC, Kellner JR, Forde AJ, Gruner DS, Parker JD, Rodriguez W, Feller IC. Poleward expansion of mangroves is a threshold response to decreased frequency of extreme cold events. Proc Natl Acad Sci. 2014; 111 (2):723–727. doi: 10.1073/pnas.1315800111. [ PMC free article ] [ PubMed ] [ CrossRef ] [ Google Scholar ]
- Cell CC (2009) Climate change and health impacts in Bangladesh. Clima Chang Cell DoE MoEF
- Chandio AA, Jiang Y, Rehman A, Rauf A (2020) Short and long-run impacts of climate change on agriculture: an empirical evidence from China. Int J Clim Chang Strat Manag
- Chaudhary P, Rai S, Wangdi S, Mao A, Rehman N, Chettri S, Bawa KS (2011) Consistency of local perceptions of climate change in the Kangchenjunga Himalaya landscape. Curr Sci 504–513
- Chien F, Anwar A, Hsu CC, Sharif A, Razzaq A, Sinha A (2021) The role of information and communication technology in encountering environmental degradation: proposing an SDG framework for the BRICS countries. Technol Soc 65:101587
- Cooper C, Booth A, Varley-Campbell J, Britten N, Garside R. Defining the process to literature searching in systematic reviews: a literature review of guidance and supporting studies. BMC Med Res Methodol. 2018; 18 (1):1–14. doi: 10.1186/s12874-018-0545-3. [ PMC free article ] [ PubMed ] [ CrossRef ] [ Google Scholar ]
- Costello A, Abbas M, Allen A, Ball S, Bell S, Bellamy R, Kett M. Managing the health effects of climate change: lancet and University College London Institute for Global Health Commission. The Lancet. 2009; 373 (9676):1693–1733. doi: 10.1016/S0140-6736(09)60935-1. [ PubMed ] [ CrossRef ] [ Google Scholar ]
- Cruz DLA (2015) Mother Figured. University of Chicago Press. Retrieved from, 10.7208/9780226315072
- Cui W, Ouyang T, Qiu Y, Cui D (2021) Literature Review of the Implications of Exercise Rehabilitation Strategies for SARS Patients on the Recovery of COVID-19 Patients. Paper presented at the Healthcare [ PMC free article ] [ PubMed ]
- Davidson D. Gaps in agricultural climate adaptation research. Nat Clim Chang. 2016; 6 (5):433–435. doi: 10.1038/nclimate3007. [ CrossRef ] [ Google Scholar ]
- Diffenbaugh NS, Singh D, Mankin JS, Horton DE, Swain DL, Touma D, Tsiang M. Quantifying the influence of global warming on unprecedented extreme climate events. Proc Natl Acad Sci. 2017; 114 (19):4881–4886. doi: 10.1073/pnas.1618082114. [ PMC free article ] [ PubMed ] [ CrossRef ] [ Google Scholar ]
- Dimri A, Kumar D, Choudhary A, Maharana P. Future changes over the Himalayas: mean temperature. Global Planet Change. 2018; 162 :235–251. doi: 10.1016/j.gloplacha.2018.01.014. [ CrossRef ] [ Google Scholar ]
- Dullinger S, Gattringer A, Thuiller W, Moser D, Zimmermann N, Guisan A. Extinction debt of high-mountain plants under twenty-first-century climate change. Nat Clim Chang: Nature Publishing Group; 2012. [ Google Scholar ]
- Dupuis I, Dumas C. Influence of temperature stress on in vitro fertilization and heat shock protein synthesis in maize (Zea mays L.) reproductive tissues. Plant Physiol. 1990; 94 (2):665–670. doi: 10.1104/pp.94.2.665. [ PMC free article ] [ PubMed ] [ CrossRef ] [ Google Scholar ]
- Edreira JR, Otegui ME. Heat stress in temperate and tropical maize hybrids: a novel approach for assessing sources of kernel loss in field conditions. Field Crop Res. 2013; 142 :58–67. doi: 10.1016/j.fcr.2012.11.009. [ CrossRef ] [ Google Scholar ]
- Edreira JR, Carpici EB, Sammarro D, Otegui M. Heat stress effects around flowering on kernel set of temperate and tropical maize hybrids. Field Crop Res. 2011; 123 (2):62–73. doi: 10.1016/j.fcr.2011.04.015. [ CrossRef ] [ Google Scholar ]
- Ellison D, Morris CE, Locatelli B, Sheil D, Cohen J, Murdiyarso D, Pokorny J. Trees, forests and water: Cool insights for a hot world. Glob Environ Chang. 2017; 43 :51–61. doi: 10.1016/j.gloenvcha.2017.01.002. [ CrossRef ] [ Google Scholar ]
- Elsayed ZM, Eldehna WM, Abdel-Aziz MM, El Hassab MA, Elkaeed EB, Al-Warhi T, Mohammed ER. Development of novel isatin–nicotinohydrazide hybrids with potent activity against susceptible/resistant Mycobacterium tuberculosis and bronchitis causing–bacteria. J Enzyme Inhib Med Chem. 2021; 36 (1):384–393. doi: 10.1080/14756366.2020.1868450. [ PMC free article ] [ PubMed ] [ CrossRef ] [ Google Scholar ]
- EM-DAT (2020) EMDAT: OFDA/CRED International Disaster Database, Université catholique de Louvain – Brussels – Belgium. from http://www.emdat.be
- EPA U (2018) United States Environmental Protection Agency, EPA Year in Review
- Erman A, De Vries Robbe SA, Thies SF, Kabir K, Maruo M (2021) Gender Dimensions of Disaster Risk and Resilience
- Fand BB, Kamble AL, Kumar M. Will climate change pose serious threat to crop pest management: a critical review. Int J Sci Res Publ. 2012; 2 (11):1–14. [ Google Scholar ]
- FAO (2018).The State of the World’s Forests 2018 - Forest Pathways to Sustainable Development.
- Fardous S Perception of climate change in Kaptai National Park. Rural Livelihoods and Protected Landscape: Co-Management in the Wetlands and Forests of Bangladesh, 186–204
- Farooq M, Bramley H, Palta JA, Siddique KH. Heat stress in wheat during reproductive and grain-filling phases. Crit Rev Plant Sci. 2011; 30 (6):491–507. doi: 10.1080/07352689.2011.615687. [ CrossRef ] [ Google Scholar ]
- Feliciano D, Recha J, Ambaw G, MacSween K, Solomon D, Wollenberg E (2022) Assessment of agricultural emissions, climate change mitigation and adaptation practices in Ethiopia. Clim Policy 1–18
- Ferreira JJ, Fernandes CI, Ferreira FA (2020) Technology transfer, climate change mitigation, and environmental patent impact on sustainability and economic growth: a comparison of European countries. Technol Forecast Soc Change 150:119770
- Fettig CJ, Reid ML, Bentz BJ, Sevanto S, Spittlehouse DL, Wang T. Changing climates, changing forests: a western North American perspective. J Forest. 2013; 111 (3):214–228. doi: 10.5849/jof.12-085. [ CrossRef ] [ Google Scholar ]
- Fischer AP. Characterizing behavioral adaptation to climate change in temperate forests. Landsc Urban Plan. 2019; 188 :72–79. doi: 10.1016/j.landurbplan.2018.09.024. [ CrossRef ] [ Google Scholar ]
- Flannigan M, Cantin AS, De Groot WJ, Wotton M, Newbery A, Gowman LM. Global wildland fire season severity in the 21st century. For Ecol Manage. 2013; 294 :54–61. doi: 10.1016/j.foreco.2012.10.022. [ CrossRef ] [ Google Scholar ]
- Fossheim M, Primicerio R, Johannesen E, Ingvaldsen RB, Aschan MM, Dolgov AV. Recent warming leads to a rapid borealization of fish communities in the Arctic. Nat Clim Chang. 2015; 5 (7):673–677. doi: 10.1038/nclimate2647. [ CrossRef ] [ Google Scholar ]
- Füssel HM, Hildén M (2014) How is uncertainty addressed in the knowledge base for national adaptation planning? Adapting to an Uncertain Climate (pp. 41–66): Springer
- Gambín BL, Borrás L, Otegui ME. Source–sink relations and kernel weight differences in maize temperate hybrids. Field Crop Res. 2006; 95 (2–3):316–326. doi: 10.1016/j.fcr.2005.04.002. [ CrossRef ] [ Google Scholar ]
- Gambín B, Borrás L. Resource distribution and the trade-off between seed number and seed weight: a comparison across crop species. Annals of Applied Biology. 2010; 156 (1):91–102. doi: 10.1111/j.1744-7348.2009.00367.x. [ CrossRef ] [ Google Scholar ]
- Gampe D, Nikulin G, Ludwig R. Using an ensemble of regional climate models to assess climate change impacts on water scarcity in European river basins. Sci Total Environ. 2016; 573 :1503–1518. doi: 10.1016/j.scitotenv.2016.08.053. [ PubMed ] [ CrossRef ] [ Google Scholar ]
- García GA, Dreccer MF, Miralles DJ, Serrago RA. High night temperatures during grain number determination reduce wheat and barley grain yield: a field study. Glob Change Biol. 2015; 21 (11):4153–4164. doi: 10.1111/gcb.13009. [ PubMed ] [ CrossRef ] [ Google Scholar ]
- Garner E, Inyang M, Garvey E, Parks J, Glover C, Grimaldi A, Edwards MA. Impact of blending for direct potable reuse on premise plumbing microbial ecology and regrowth of opportunistic pathogens and antibiotic resistant bacteria. Water Res. 2019; 151 :75–86. doi: 10.1016/j.watres.2018.12.003. [ PubMed ] [ CrossRef ] [ Google Scholar ]
- Gleditsch NP (2021) This time is different! Or is it? NeoMalthusians and environmental optimists in the age of climate change. J Peace Res 0022343320969785
- Godfray HCJ, Beddington JR, Crute IR, Haddad L, Lawrence D, Muir JF, Toulmin C. Food security: the challenge of feeding 9 billion people. Science. 2010; 327 (5967):812–818. doi: 10.1126/science.1185383. [ PubMed ] [ CrossRef ] [ Google Scholar ]
- Goes S, Hasterok D, Schutt DL, Klöcking M (2020) Continental lithospheric temperatures: A review. Phys Earth Planet Inter 106509
- Gorst A, Dehlavi A, Groom B. Crop productivity and adaptation to climate change in Pakistan. Environ Dev Econ. 2018; 23 (6):679–701. doi: 10.1017/S1355770X18000232. [ CrossRef ] [ Google Scholar ]
- Gosling SN, Arnell NW. A global assessment of the impact of climate change on water scarcity. Clim Change. 2016; 134 (3):371–385. doi: 10.1007/s10584-013-0853-x. [ CrossRef ] [ Google Scholar ]
- Gössling S, Scott D, Hall CM, Ceron J-P, Dubois G. Consumer behaviour and demand response of tourists to climate change. Ann Tour Res. 2012; 39 (1):36–58. doi: 10.1016/j.annals.2011.11.002. [ CrossRef ] [ Google Scholar ]
- Gourdji SM, Sibley AM, Lobell DB. Global crop exposure to critical high temperatures in the reproductive period: historical trends and future projections. Environ Res Lett. 2013; 8 (2):024041. doi: 10.1088/1748-9326/8/2/024041. [ CrossRef ] [ Google Scholar ]
- Grieg E Responsible Consumption and Production
- Gunter BG, Rahman A, Rahman A (2008) How Vulnerable are Bangladesh’s Indigenous People to Climate Change? Bangladesh Development Research Center (BDRC)
- Hall CM, Amelung B, Cohen S, Eijgelaar E, Gössling S, Higham J, Scott D. On climate change skepticism and denial in tourism. J Sustain Tour. 2015; 23 (1):4–25. doi: 10.1080/09669582.2014.953544. [ CrossRef ] [ Google Scholar ]
- Hartmann H, Moura CF, Anderegg WR, Ruehr NK, Salmon Y, Allen CD, Galbraith D. Research frontiers for improving our understanding of drought-induced tree and forest mortality. New Phytol. 2018; 218 (1):15–28. doi: 10.1111/nph.15048. [ PubMed ] [ CrossRef ] [ Google Scholar ]
- Hatfield JL, Prueger JH. Temperature extremes: Effect on plant growth and development. Weather and Climate Extremes. 2015; 10 :4–10. doi: 10.1016/j.wace.2015.08.001. [ CrossRef ] [ Google Scholar ]
- Hatfield JL, Boote KJ, Kimball B, Ziska L, Izaurralde RC, Ort D, Wolfe D. Climate impacts on agriculture: implications for crop production. Agron J. 2011; 103 (2):351–370. doi: 10.2134/agronj2010.0303. [ CrossRef ] [ Google Scholar ]
- Hendriksen RS, Munk P, Njage P, Van Bunnik B, McNally L, Lukjancenko O, Kjeldgaard J. Global monitoring of antimicrobial resistance based on metagenomics analyses of urban sewage. Nat Commun. 2019; 10 (1):1124. doi: 10.1038/s41467-019-08853-3. [ PMC free article ] [ PubMed ] [ CrossRef ] [ Google Scholar ]
- Huang S (2004) Global trade patterns in fruits and vegetables. USDA-ERS Agriculture and Trade Report No. WRS-04–06
- Huang W, Gao Q-X, Cao G-L, Ma Z-Y, Zhang W-D, Chao Q-C. Effect of urban symbiosis development in China on GHG emissions reduction. Adv Clim Chang Res. 2016; 7 (4):247–252. doi: 10.1016/j.accre.2016.12.003. [ CrossRef ] [ Google Scholar ]
- Huang Y, Haseeb M, Usman M, Ozturk I (2022) Dynamic association between ICT, renewable energy, economic complexity and ecological footprint: Is there any difference between E-7 (developing) and G-7 (developed) countries? Tech Soc 68:101853
- Hubbart JA, Guyette R, Muzika R-M. More than drought: precipitation variance, excessive wetness, pathogens and the future of the western edge of the eastern deciduous forest. Sci Total Environ. 2016; 566 :463–467. doi: 10.1016/j.scitotenv.2016.05.108. [ PubMed ] [ CrossRef ] [ Google Scholar ]
- Hussain M, Butt AR, Uzma F, Ahmed R, Irshad S, Rehman A, Yousaf B. A comprehensive review of climate change impacts, adaptation, and mitigation on environmental and natural calamities in Pakistan. Environ Monit Assess. 2020; 192 (1):48. doi: 10.1007/s10661-019-7956-4. [ PubMed ] [ CrossRef ] [ Google Scholar ]
- Hussain M, Liu G, Yousaf B, Ahmed R, Uzma F, Ali MU, Butt AR. Regional and sectoral assessment on climate-change in Pakistan: social norms and indigenous perceptions on climate-change adaptation and mitigation in relation to global context. J Clean Prod. 2018; 200 :791–808. doi: 10.1016/j.jclepro.2018.07.272. [ CrossRef ] [ Google Scholar ]
- Intergov. Panel Clim Chang 33 from 10.1017/CBO9781107415324
- Ionescu C, Klein RJ, Hinkel J, Kumar KK, Klein R. Towards a formal framework of vulnerability to climate change. Environ Model Assess. 2009; 14 (1):1–16. doi: 10.1007/s10666-008-9179-x. [ CrossRef ] [ Google Scholar ]
- IPCC (2013) Summary for policymakers. Clim Chang Phys Sci Basis Contrib Work Gr I Fifth Assess Rep
- Ishikawa-Ishiwata Y, Furuya J (2022) Economic evaluation and climate change adaptation measures for rice production in vietnam using a supply and demand model: special emphasis on the Mekong River Delta region in Vietnam. In Interlocal Adaptations to Climate Change in East and Southeast Asia (pp. 45–53). Springer, Cham
- Izaguirre C, Losada I, Camus P, Vigh J, Stenek V. Climate change risk to global port operations. Nat Clim Chang. 2021; 11 (1):14–20. doi: 10.1038/s41558-020-00937-z. [ CrossRef ] [ Google Scholar ]
- Jactel H, Koricheva J, Castagneyrol B (2019) Responses of forest insect pests to climate change: not so simple. Current opinion in insect science [ PubMed ]
- Jahanzad E, Holtz BA, Zuber CA, Doll D, Brewer KM, Hogan S, Gaudin AC. Orchard recycling improves climate change adaptation and mitigation potential of almond production systems. PLoS ONE. 2020; 15 (3):e0229588. doi: 10.1371/journal.pone.0229588. [ PMC free article ] [ PubMed ] [ CrossRef ] [ Google Scholar ]
- Jurgilevich A, Räsänen A, Groundstroem F, Juhola S. A systematic review of dynamics in climate risk and vulnerability assessments. Environ Res Lett. 2017; 12 (1):013002. doi: 10.1088/1748-9326/aa5508. [ CrossRef ] [ Google Scholar ]
- Karami E (2012) Climate change, resilience and poverty in the developing world. Paper presented at the Culture, Politics and Climate change conference
- Kärkkäinen L, Lehtonen H, Helin J, Lintunen J, Peltonen-Sainio P, Regina K, . . . Packalen T (2020) Evaluation of policy instruments for supporting greenhouse gas mitigation efforts in agricultural and urban land use. Land Use Policy 99:104991
- Karkman A, Do TT, Walsh F, Virta MP. Antibiotic-resistance genes in waste water. Trends Microbiol. 2018; 26 (3):220–228. doi: 10.1016/j.tim.2017.09.005. [ PubMed ] [ CrossRef ] [ Google Scholar ]
- Kohfeld KE, Le Quéré C, Harrison SP, Anderson RF. Role of marine biology in glacial-interglacial CO2 cycles. Science. 2005; 308 (5718):74–78. doi: 10.1126/science.1105375. [ PubMed ] [ CrossRef ] [ Google Scholar ]
- Kongsager R. Linking climate change adaptation and mitigation: a review with evidence from the land-use sectors. Land. 2018; 7 (4):158. doi: 10.3390/land7040158. [ CrossRef ] [ Google Scholar ]
- Kurz WA, Dymond C, Stinson G, Rampley G, Neilson E, Carroll A, Safranyik L. Mountain pine beetle and forest carbon feedback to climate change. Nature. 2008; 452 (7190):987. doi: 10.1038/nature06777. [ PubMed ] [ CrossRef ] [ Google Scholar ]
- Lamperti F, Bosetti V, Roventini A, Tavoni M, Treibich T (2021) Three green financial policies to address climate risks. J Financial Stab 54:100875
- Leal Filho W, Azeiteiro UM, Balogun AL, Setti AFF, Mucova SA, Ayal D, . . . Oguge NO (2021) The influence of ecosystems services depletion to climate change adaptation efforts in Africa. Sci Total Environ 146414 [ PubMed ]
- Lehner F, Coats S, Stocker TF, Pendergrass AG, Sanderson BM, Raible CC, Smerdon JE. Projected drought risk in 1.5 C and 2 C warmer climates. Geophys Res Lett. 2017; 44 (14):7419–7428. doi: 10.1002/2017GL074117. [ CrossRef ] [ Google Scholar ]
- Lemery J, Knowlton K, Sorensen C (2021) Global climate change and human health: from science to practice: John Wiley & Sons
- Leppänen S, Saikkonen L, Ollikainen M (2014) Impact of Climate Change on cereal grain production in Russia: Mimeo
- Lipczynska-Kochany E. Effect of climate change on humic substances and associated impacts on the quality of surface water and groundwater: a review. Sci Total Environ. 2018; 640 :1548–1565. doi: 10.1016/j.scitotenv.2018.05.376. [ PubMed ] [ CrossRef ] [ Google Scholar ]
- livescience.com. New coronavirus may have ‘jumped’ to humans from snakes, study finds, live science,. from < https://www.livescience.com/new-coronavirus-origin-snakes.html > accessed on Jan 2020
- Lobell DB, Field CB. Global scale climate–crop yield relationships and the impacts of recent warming. Environ Res Lett. 2007; 2 (1):014002. doi: 10.1088/1748-9326/2/1/014002. [ CrossRef ] [ Google Scholar ]
- Lobell DB, Gourdji SM. The influence of climate change on global crop productivity. Plant Physiol. 2012; 160 (4):1686–1697. doi: 10.1104/pp.112.208298. [ PMC free article ] [ PubMed ] [ CrossRef ] [ Google Scholar ]
- Ma L, Li B, Zhang T. New insights into antibiotic resistome in drinking water and management perspectives: a metagenomic based study of small-sized microbes. Water Res. 2019; 152 :191–201. doi: 10.1016/j.watres.2018.12.069. [ PubMed ] [ CrossRef ] [ Google Scholar ]
- Macchi M, Oviedo G, Gotheil S, Cross K, Boedhihartono A, Wolfangel C, Howell M (2008) Indigenous and traditional peoples and climate change. International Union for the Conservation of Nature, Gland, Suiza
- Mall RK, Gupta A, Sonkar G (2017) Effect of climate change on agricultural crops. In Current developments in biotechnology and bioengineering (pp. 23–46). Elsevier
- Manes S, Costello MJ, Beckett H, Debnath A, Devenish-Nelson E, Grey KA, . . . Krause C (2021) Endemism increases species’ climate change risk in areas of global biodiversity importance. Biol Conserv 257:109070
- Mannig B, Pollinger F, Gafurov A, Vorogushyn S, Unger-Shayesteh K (2018) Impacts of climate change in Central Asia Encyclopedia of the Anthropocene (pp. 195–203): Elsevier
- Martínez-Alvarado O, Gray SL, Hart NC, Clark PA, Hodges K, Roberts MJ. Increased wind risk from sting-jet windstorms with climate change. Environ Res Lett. 2018; 13 (4):044002. doi: 10.1088/1748-9326/aaae3a. [ CrossRef ] [ Google Scholar ]
- Matsui T, Omasa K, Horie T. The difference in sterility due to high temperatures during the flowering period among japonica-rice varieties. Plant Production Science. 2001; 4 (2):90–93. doi: 10.1626/pps.4.90. [ CrossRef ] [ Google Scholar ]
- Meierrieks D (2021) Weather shocks, climate change and human health. World Dev 138:105228
- Michel D, Eriksson M, Klimes M (2021) Climate change and (in) security in transboundary river basins Handbook of Security and the Environment: Edward Elgar Publishing
- Mihiretu A, Okoyo EN, Lemma T. Awareness of climate change and its associated risks jointly explain context-specific adaptation in the Arid-tropics. Northeast Ethiopia SN Social Sciences. 2021; 1 (2):1–18. [ Google Scholar ]
- Millar CI, Stephenson NL. Temperate forest health in an era of emerging megadisturbance. Science. 2015; 349 (6250):823–826. doi: 10.1126/science.aaa9933. [ PubMed ] [ CrossRef ] [ Google Scholar ]
- Mishra A, Bruno E, Zilberman D (2021) Compound natural and human disasters: Managing drought and COVID-19 to sustain global agriculture and food sectors. Sci Total Environ 754:142210 [ PMC free article ] [ PubMed ]
- Mosavi SH, Soltani S, Khalilian S (2020) Coping with climate change in agriculture: Evidence from Hamadan-Bahar plain in Iran. Agric Water Manag 241:106332
- Murshed M (2020) An empirical analysis of the non-linear impacts of ICT-trade openness on renewable energy transition, energy efficiency, clean cooking fuel access and environmental sustainability in South Asia. Environ Sci Pollut Res 27(29):36254–36281. 10.1007/s11356-020-09497-3 [ PMC free article ] [ PubMed ]
- Murshed M. Pathways to clean cooking fuel transition in low and middle income Sub-Saharan African countries: the relevance of improving energy use efficiency. Sustainable Production and Consumption. 2022; 30 :396–412. doi: 10.1016/j.spc.2021.12.016. [ CrossRef ] [ Google Scholar ]
- Murshed M, Dao NTT. Revisiting the CO2 emission-induced EKC hypothesis in South Asia: the role of Export Quality Improvement. GeoJournal. 2020 doi: 10.1007/s10708-020-10270-9. [ CrossRef ] [ Google Scholar ]
- Murshed M, Abbass K, Rashid S. Modelling renewable energy adoption across south Asian economies: Empirical evidence from Bangladesh, India, Pakistan and Sri Lanka. Int J Finan Eco. 2021; 26 (4):5425–5450. doi: 10.1002/ijfe.2073. [ CrossRef ] [ Google Scholar ]
- Murshed M, Nurmakhanova M, Elheddad M, Ahmed R. Value addition in the services sector and its heterogeneous impacts on CO2 emissions: revisiting the EKC hypothesis for the OPEC using panel spatial estimation techniques. Environ Sci Pollut Res. 2020; 27 (31):38951–38973. doi: 10.1007/s11356-020-09593-4. [ PubMed ] [ CrossRef ] [ Google Scholar ]
- Murshed M, Nurmakhanova M, Al-Tal R, Mahmood H, Elheddad M, Ahmed R (2022) Can intra-regional trade, renewable energy use, foreign direct investments, and economic growth reduce ecological footprints in South Asia? Energy Sources, Part B: Economics, Planning, and Policy. 10.1080/15567249.2022.2038730
- Neuvonen M, Sievänen T, Fronzek S, Lahtinen I, Veijalainen N, Carter TR. Vulnerability of cross-country skiing to climate change in Finland–an interactive mapping tool. J Outdoor Recreat Tour. 2015; 11 :64–79. doi: 10.1016/j.jort.2015.06.010. [ CrossRef ] [ Google Scholar ]
- npr.org. Please Help Me.’ What people in China are saying about the outbreak on social media, npr.org, . from < https://www.npr.org/sections/goatsandsoda/2020/01/24/799000379/please-help-me-what-people-in-china-are-saying-about-the-outbreak-on-social-medi >, Accessed on 26 Jan 2020.
- Ogden LE. Climate change, pathogens, and people: the challenges of monitoring a moving target. Bioscience. 2018; 68 (10):733–739. doi: 10.1093/biosci/biy101. [ CrossRef ] [ Google Scholar ]
- Ortiz AMD, Outhwaite CL, Dalin C, Newbold T. A review of the interactions between biodiversity, agriculture, climate change, and international trade: research and policy priorities. One Earth. 2021; 4 (1):88–101. doi: 10.1016/j.oneear.2020.12.008. [ CrossRef ] [ Google Scholar ]
- Ortiz R. Crop genetic engineering under global climate change. Ann Arid Zone. 2008; 47 (3):343. [ Google Scholar ]
- Otegui MAE, Bonhomme R. Grain yield components in maize: I. Ear growth and kernel set. Field Crop Res. 1998; 56 (3):247–256. doi: 10.1016/S0378-4290(97)00093-2. [ CrossRef ] [ Google Scholar ]
- Pachauri RK, Allen MR, Barros VR, Broome J, Cramer W, Christ R, . . . Dasgupta P (2014) Climate change 2014: synthesis report. Contribution of Working Groups I, II and III to the fifth assessment report of the Intergovernmental Panel on Climate Change: Ipcc
- Pal JK. Visualizing the knowledge outburst in global research on COVID-19. Scientometrics. 2021; 126 (5):4173–4193. doi: 10.1007/s11192-021-03912-3. [ PMC free article ] [ PubMed ] [ CrossRef ] [ Google Scholar ]
- Panda R, Behera S, Kashyap P. Effective management of irrigation water for wheat under stressed conditions. Agric Water Manag. 2003; 63 (1):37–56. doi: 10.1016/S0378-3774(03)00099-4. [ CrossRef ] [ Google Scholar ]
- Pärnänen KM, Narciso-da-Rocha C, Kneis D, Berendonk TU, Cacace D, Do TT, Jaeger T. Antibiotic resistance in European wastewater treatment plants mirrors the pattern of clinical antibiotic resistance prevalence. Sci Adv. 2019; 5 (3):eaau9124. doi: 10.1126/sciadv.aau9124. [ PMC free article ] [ PubMed ] [ CrossRef ] [ Google Scholar ]
- Parry M, Parry ML, Canziani O, Palutikof J, Van der Linden P, Hanson C (2007) Climate change 2007-impacts, adaptation and vulnerability: Working group II contribution to the fourth assessment report of the IPCC (Vol. 4): Cambridge University Press
- Patz JA, Campbell-Lendrum D, Holloway T, Foley JA. Impact of regional climate change on human health. Nature. 2005; 438 (7066):310–317. doi: 10.1038/nature04188. [ PubMed ] [ CrossRef ] [ Google Scholar ]
- Patz JA, Graczyk TK, Geller N, Vittor AY. Effects of environmental change on emerging parasitic diseases. Int J Parasitol. 2000; 30 (12–13):1395–1405. doi: 10.1016/S0020-7519(00)00141-7. [ PubMed ] [ CrossRef ] [ Google Scholar ]
- Pautasso M, Döring TF, Garbelotto M, Pellis L, Jeger MJ. Impacts of climate change on plant diseases—opinions and trends. Eur J Plant Pathol. 2012; 133 (1):295–313. doi: 10.1007/s10658-012-9936-1. [ CrossRef ] [ Google Scholar ]
- Peng S, Huang J, Sheehy JE, Laza RC, Visperas RM, Zhong X, Cassman KG. Rice yields decline with higher night temperature from global warming. Proc Natl Acad Sci. 2004; 101 (27):9971–9975. doi: 10.1073/pnas.0403720101. [ PMC free article ] [ PubMed ] [ CrossRef ] [ Google Scholar ]
- Pereira HM, Ferrier S, Walters M, Geller GN, Jongman R, Scholes RJ, Cardoso A. Essential biodiversity variables. Science. 2013; 339 (6117):277–278. doi: 10.1126/science.1229931. [ PubMed ] [ CrossRef ] [ Google Scholar ]
- Perera K, De Silva K, Amarasinghe M. Potential impact of predicted sea level rise on carbon sink function of mangrove ecosystems with special reference to Negombo estuary, Sri Lanka. Global Planet Change. 2018; 161 :162–171. doi: 10.1016/j.gloplacha.2017.12.016. [ CrossRef ] [ Google Scholar ]
- Pfadenhauer JS, Klötzli FA (2020) Zonal Vegetation of the Subtropical (Warm–Temperate) Zone with Winter Rain. In Global Vegetation (pp. 455–514). Springer, Cham
- Phillips JD. Environmental gradients and complexity in coastal landscape response to sea level rise. CATENA. 2018; 169 :107–118. doi: 10.1016/j.catena.2018.05.036. [ CrossRef ] [ Google Scholar ]
- Pirasteh-Anosheh H, Parnian A, Spasiano D, Race M, Ashraf M (2021) Haloculture: A system to mitigate the negative impacts of pandemics on the environment, society and economy, emphasizing COVID-19. Environ Res 111228 [ PMC free article ] [ PubMed ]
- Pruden A, Larsson DJ, Amézquita A, Collignon P, Brandt KK, Graham DW, Snape JR. Management options for reducing the release of antibiotics and antibiotic resistance genes to the environment. Environ Health Perspect. 2013; 121 (8):878–885. doi: 10.1289/ehp.1206446. [ PMC free article ] [ PubMed ] [ CrossRef ] [ Google Scholar ]
- Qasim MZ, Hammad HM, Abbas F, Saeed S, Bakhat HF, Nasim W, Fahad S. The potential applications of picotechnology in biomedical and environmental sciences. Environ Sci Pollut Res. 2020; 27 (1):133–142. doi: 10.1007/s11356-019-06554-4. [ PubMed ] [ CrossRef ] [ Google Scholar ]
- Qasim MZ, Hammad HM, Maqsood F, Tariq T, Chawla MS Climate Change Implication on Cereal Crop Productivity
- Rahman M, Alam K. Forest dependent indigenous communities’ perception and adaptation to climate change through local knowledge in the protected area—a Bangladesh case study. Climate. 2016; 4 (1):12. doi: 10.3390/cli4010012. [ CrossRef ] [ Google Scholar ]
- Ramankutty N, Mehrabi Z, Waha K, Jarvis L, Kremen C, Herrero M, Rieseberg LH. Trends in global agricultural land use: implications for environmental health and food security. Annu Rev Plant Biol. 2018; 69 :789–815. doi: 10.1146/annurev-arplant-042817-040256. [ PubMed ] [ CrossRef ] [ Google Scholar ]
- Rehman A, Ma H, Ahmad M, Irfan M, Traore O, Chandio AA (2021) Towards environmental Sustainability: devolving the influence of carbon dioxide emission to population growth, climate change, Forestry, livestock and crops production in Pakistan. Ecol Indic 125:107460
- Reichstein M, Carvalhais N. Aspects of forest biomass in the Earth system: its role and major unknowns. Surv Geophys. 2019; 40 (4):693–707. doi: 10.1007/s10712-019-09551-x. [ CrossRef ] [ Google Scholar ]
- Reidsma P, Ewert F, Boogaard H, van Diepen K. Regional crop modelling in Europe: the impact of climatic conditions and farm characteristics on maize yields. Agric Syst. 2009; 100 (1–3):51–60. doi: 10.1016/j.agsy.2008.12.009. [ CrossRef ] [ Google Scholar ]
- Ritchie H, Roser M (2014) Natural disasters. Our World in Data
- Rizvi AR, Baig S, Verdone M. Ecosystems based adaptation: knowledge gaps in making an economic case for investing in nature based solutions for climate change. Gland, Switzerland: IUCN; 2015. p. 48. [ Google Scholar ]
- Roscher C, Fergus AJ, Petermann JS, Buchmann N, Schmid B, Schulze E-D. What happens to the sown species if a biodiversity experiment is not weeded? Basic Appl Ecol. 2013; 14 (3):187–198. doi: 10.1016/j.baae.2013.01.003. [ CrossRef ] [ Google Scholar ]
- Rosenzweig C, Elliott J, Deryng D, Ruane AC, Müller C, Arneth A, Khabarov N. Assessing agricultural risks of climate change in the 21st century in a global gridded crop model intercomparison. Proc Natl Acad Sci. 2014; 111 (9):3268–3273. doi: 10.1073/pnas.1222463110. [ PMC free article ] [ PubMed ] [ CrossRef ] [ Google Scholar ]
- Rosenzweig C, Iglesius A, Yang XB, Epstein PR, Chivian E (2001) Climate change and extreme weather events-implications for food production, plant diseases, and pests
- Sadras VO, Slafer GA. Environmental modulation of yield components in cereals: heritabilities reveal a hierarchy of phenotypic plasticities. Field Crop Res. 2012; 127 :215–224. doi: 10.1016/j.fcr.2011.11.014. [ CrossRef ] [ Google Scholar ]
- Salvucci ME, Crafts-Brandner SJ. Inhibition of photosynthesis by heat stress: the activation state of Rubisco as a limiting factor in photosynthesis. Physiol Plant. 2004; 120 (2):179–186. doi: 10.1111/j.0031-9317.2004.0173.x. [ PubMed ] [ CrossRef ] [ Google Scholar ]
- Santos WS, Gurgel-Gonçalves R, Garcez LM, Abad-Franch F. Deforestation effects on Attalea palms and their resident Rhodnius, vectors of Chagas disease, in eastern Amazonia. PLoS ONE. 2021; 16 (5):e0252071. doi: 10.1371/journal.pone.0252071. [ PMC free article ] [ PubMed ] [ CrossRef ] [ Google Scholar ]
- Sarkar P, Debnath N, Reang D (2021) Coupled human-environment system amid COVID-19 crisis: a conceptual model to understand the nexus. Sci Total Environ 753:141757 [ PMC free article ] [ PubMed ]
- Schlenker W, Roberts MJ. Nonlinear temperature effects indicate severe damages to US crop yields under climate change. Proc Natl Acad Sci. 2009; 106 (37):15594–15598. doi: 10.1073/pnas.0906865106. [ PMC free article ] [ PubMed ] [ CrossRef ] [ Google Scholar ]
- Schoene DH, Bernier PY. Adapting forestry and forests to climate change: a challenge to change the paradigm. Forest Policy Econ. 2012; 24 :12–19. doi: 10.1016/j.forpol.2011.04.007. [ CrossRef ] [ Google Scholar ]
- Schuurmans C (2021) The world heat budget: expected changes Climate Change (pp. 1–15): CRC Press
- Scott D. Sustainable Tourism and the Grand Challenge of Climate Change. Sustainability. 2021; 13 (4):1966. doi: 10.3390/su13041966. [ CrossRef ] [ Google Scholar ]
- Scott D, McBoyle G, Schwartzentruber M. Climate change and the distribution of climatic resources for tourism in North America. Climate Res. 2004; 27 (2):105–117. doi: 10.3354/cr027105. [ CrossRef ] [ Google Scholar ]
- Semenov MA. Impacts of climate change on wheat in England and Wales. J R Soc Interface. 2009; 6 (33):343–350. doi: 10.1098/rsif.2008.0285. [ PMC free article ] [ PubMed ] [ CrossRef ] [ Google Scholar ]
- Shaffril HAM, Krauss SE, Samsuddin SF. A systematic review on Asian’s farmers’ adaptation practices towards climate change. Sci Total Environ. 2018; 644 :683–695. doi: 10.1016/j.scitotenv.2018.06.349. [ PubMed ] [ CrossRef ] [ Google Scholar ]
- Shahbaz M, Balsalobre-Lorente D, Sinha A (2019) Foreign direct Investment–CO2 emissions nexus in Middle East and North African countries: Importance of biomass energy consumption. J Clean Product 217:603–614
- Sharif A, Mishra S, Sinha A, Jiao Z, Shahbaz M, Afshan S (2020) The renewable energy consumption-environmental degradation nexus in Top-10 polluted countries: Fresh insights from quantile-on-quantile regression approach. Renew Energy 150:670–690
- Sharma R. Impacts on human health of climate and land use change in the Hindu Kush-Himalayan region. Mt Res Dev. 2012; 32 (4):480–486. doi: 10.1659/MRD-JOURNAL-D-12-00068.1. [ CrossRef ] [ Google Scholar ]
- Sharma R, Sinha A, Kautish P. Examining the impacts of economic and demographic aspects on the ecological footprint in South and Southeast Asian countries. Environ Sci Pollut Res. 2020; 27 (29):36970–36982. doi: 10.1007/s11356-020-09659-3. [ PubMed ] [ CrossRef ] [ Google Scholar ]
- Smit B, Burton I, Klein RJ, Wandel J (2000) An anatomy of adaptation to climate change and variability Societal adaptation to climate variability and change (pp. 223–251): Springer
- Song Y, Fan H, Tang X, Luo Y, Liu P, Chen Y (2021) The effects of severe acute respiratory syndrome coronavirus 2 (SARS-CoV-2) on ischemic stroke and the possible underlying mechanisms. Int J Neurosci 1–20 [ PMC free article ] [ PubMed ]
- Sovacool BK, Griffiths S, Kim J, Bazilian M (2021) Climate change and industrial F-gases: a critical and systematic review of developments, sociotechnical systems and policy options for reducing synthetic greenhouse gas emissions. Renew Sustain Energy Rev 141:110759
- Stewart JA, Perrine JD, Nichols LB, Thorne JH, Millar CI, Goehring KE, Wright DH. Revisiting the past to foretell the future: summer temperature and habitat area predict pika extirpations in California. J Biogeogr. 2015; 42 (5):880–890. doi: 10.1111/jbi.12466. [ CrossRef ] [ Google Scholar ]
- Stocker T, Qin D, Plattner G, Tignor M, Allen S, Boschung J, . . . Midgley P (2013) Climate change 2013: The physical science basis. Working group I contribution to the IPCC Fifth assessment report: Cambridge: Cambridge University Press. 1535p
- Stone P, Nicolas M. Wheat cultivars vary widely in their responses of grain yield and quality to short periods of post-anthesis heat stress. Funct Plant Biol. 1994; 21 (6):887–900. doi: 10.1071/PP9940887. [ CrossRef ] [ Google Scholar ]
- Su H-C, Liu Y-S, Pan C-G, Chen J, He L-Y, Ying G-G. Persistence of antibiotic resistance genes and bacterial community changes in drinking water treatment system: from drinking water source to tap water. Sci Total Environ. 2018; 616 :453–461. doi: 10.1016/j.scitotenv.2017.10.318. [ PubMed ] [ CrossRef ] [ Google Scholar ]
- Sunderlin WD, Angelsen A, Belcher B, Burgers P, Nasi R, Santoso L, Wunder S. Livelihoods, forests, and conservation in developing countries: an overview. World Dev. 2005; 33 (9):1383–1402. doi: 10.1016/j.worlddev.2004.10.004. [ CrossRef ] [ Google Scholar ]
- Symanski E, Han HA, Han I, McDaniel M, Whitworth KW, McCurdy S, . . . Delclos GL (2021) Responding to natural and industrial disasters: partnerships and lessons learned. Disaster medicine and public health preparedness 1–4 [ PMC free article ] [ PubMed ]
- Tao F, Yokozawa M, Xu Y, Hayashi Y, Zhang Z. Climate changes and trends in phenology and yields of field crops in China, 1981–2000. Agric for Meteorol. 2006; 138 (1–4):82–92. doi: 10.1016/j.agrformet.2006.03.014. [ CrossRef ] [ Google Scholar ]
- Tebaldi C, Hayhoe K, Arblaster JM, Meehl GA. Going to the extremes. Clim Change. 2006; 79 (3–4):185–211. doi: 10.1007/s10584-006-9051-4. [ CrossRef ] [ Google Scholar ]
- Testa G, Koon E, Johannesson L, McKenna G, Anthony T, Klintmalm G, Gunby R (2018) This article has been accepted for publication and undergone full peer review but has not been through the copyediting, typesetting, pagination and proofreading process, which may lead to differences between this version and the Version of Record. Please cite this article as
- Thornton PK, Lipper L (2014) How does climate change alter agricultural strategies to support food security? (Vol. 1340): Intl Food Policy Res Inst
- Tranfield D, Denyer D, Smart P. Towards a methodology for developing evidence-informed management knowledge by means of systematic review. Br J Manag. 2003; 14 (3):207–222. doi: 10.1111/1467-8551.00375. [ CrossRef ] [ Google Scholar ]
- UNEP (2017) United nations environment programme: frontiers 2017. from https://www.unenvironment.org/news-and-stories/press-release/antimicrobial-resistance - environmental-pollution-among-biggest
- Usman M, Balsalobre-Lorente D (2022) Environmental concern in the era of industrialization: Can financial development, renewable energy and natural resources alleviate some load? Ene Policy 162:112780
- Usman M, Makhdum MSA (2021) What abates ecological footprint in BRICS-T region? Exploring the influence of renewable energy, non-renewable energy, agriculture, forest area and financial development. Renew Energy 179:12–28
- Usman M, Balsalobre-Lorente D, Jahanger A, Ahmad P. Pollution concern during globalization mode in financially resource-rich countries: Do financial development, natural resources, and renewable energy consumption matter? Rene. Energy. 2022; 183 :90–102. doi: 10.1016/j.renene.2021.10.067. [ CrossRef ] [ Google Scholar ]
- Usman M, Jahanger A, Makhdum MSA, Balsalobre-Lorente D, Bashir A (2022a) How do financial development, energy consumption, natural resources, and globalization affect Arctic countries’ economic growth and environmental quality? An advanced panel data simulation. Energy 241:122515
- Usman M, Khalid K, Mehdi MA. What determines environmental deficit in Asia? Embossing the role of renewable and non-renewable energy utilization. Renew Energy. 2021; 168 :1165–1176. doi: 10.1016/j.renene.2021.01.012. [ CrossRef ] [ Google Scholar ]
- Urban MC. Accelerating extinction risk from climate change. Science. 2015; 348 (6234):571–573. doi: 10.1126/science.aaa4984. [ PubMed ] [ CrossRef ] [ Google Scholar ]
- Vale MM, Arias PA, Ortega G, Cardoso M, Oliveira BF, Loyola R, Scarano FR (2021) Climate change and biodiversity in the Atlantic Forest: best climatic models, predicted changes and impacts, and adaptation options The Atlantic Forest (pp. 253–267): Springer
- Vedwan N, Rhoades RE. Climate change in the Western Himalayas of India: a study of local perception and response. Climate Res. 2001; 19 (2):109–117. doi: 10.3354/cr019109. [ CrossRef ] [ Google Scholar ]
- Vega CR, Andrade FH, Sadras VO, Uhart SA, Valentinuz OR. Seed number as a function of growth. A comparative study in soybean, sunflower, and maize. Crop Sci. 2001; 41 (3):748–754. doi: 10.2135/cropsci2001.413748x. [ CrossRef ] [ Google Scholar ]
- Vergés A, Doropoulos C, Malcolm HA, Skye M, Garcia-Pizá M, Marzinelli EM, Vila-Concejo A. Long-term empirical evidence of ocean warming leading to tropicalization of fish communities, increased herbivory, and loss of kelp. Proc Natl Acad Sci. 2016; 113 (48):13791–13796. doi: 10.1073/pnas.1610725113. [ PMC free article ] [ PubMed ] [ CrossRef ] [ Google Scholar ]
- Verheyen R (2005) Climate change damage and international law: prevention duties and state responsibility (Vol. 54): Martinus Nijhoff Publishers
- Waheed A, Fischer TB, Khan MI. Climate Change Policy Coherence across Policies, Plans, and Strategies in Pakistan—implications for the China-Pakistan Economic Corridor Plan. Environ Manage. 2021; 67 (5):793–810. doi: 10.1007/s00267-021-01449-y. [ PMC free article ] [ PubMed ] [ CrossRef ] [ Google Scholar ]
- Wasiq M, Ahmad M (2004) Sustaining forests: a development strategy: The World Bank
- Watts N, Adger WN, Agnolucci P, Blackstock J, Byass P, Cai W, Cooper A. Health and climate change: policy responses to protect public health. The Lancet. 2015; 386 (10006):1861–1914. doi: 10.1016/S0140-6736(15)60854-6. [ PubMed ] [ CrossRef ] [ Google Scholar ]
- Weed AS, Ayres MP, Hicke JA. Consequences of climate change for biotic disturbances in North American forests. Ecol Monogr. 2013; 83 (4):441–470. doi: 10.1890/13-0160.1. [ CrossRef ] [ Google Scholar ]
- Weisheimer A, Palmer T (2005) Changing frequency of occurrence of extreme seasonal temperatures under global warming. Geophys Res Lett 32(20)
- Wernberg T, Bennett S, Babcock RC, De Bettignies T, Cure K, Depczynski M, Hovey RK. Climate-driven regime shift of a temperate marine ecosystem. Science. 2016; 353 (6295):169–172. doi: 10.1126/science.aad8745. [ PubMed ] [ CrossRef ] [ Google Scholar ]
- WHO (2018) WHO, 2018. Antimicrobial resistance
- Wilkinson DM, Sherratt TN. Why is the world green? The interactions of top–down and bottom–up processes in terrestrial vegetation ecology. Plant Ecolog Divers. 2016; 9 (2):127–140. doi: 10.1080/17550874.2016.1178353. [ CrossRef ] [ Google Scholar ]
- Wiranata IJ, Simbolon K. Increasing awareness capacity of disaster potential as a support to achieve sustainable development goal (sdg) 13 in lampung province. Jurnal Pir: Power in International Relations. 2021; 5 (2):129–146. doi: 10.22303/pir.5.2.2021.129-146. [ CrossRef ] [ Google Scholar ]
- Wiréhn L. Nordic agriculture under climate change: a systematic review of challenges, opportunities and adaptation strategies for crop production. Land Use Policy. 2018; 77 :63–74. doi: 10.1016/j.landusepol.2018.04.059. [ CrossRef ] [ Google Scholar ]
- Wu D, Su Y, Xi H, Chen X, Xie B. Urban and agriculturally influenced water contribute differently to the spread of antibiotic resistance genes in a mega-city river network. Water Res. 2019; 158 :11–21. doi: 10.1016/j.watres.2019.03.010. [ PubMed ] [ CrossRef ] [ Google Scholar ]
- Wu HX (2020) Losing Steam?—An industry origin analysis of China’s productivity slowdown Measuring Economic Growth and Productivity (pp. 137–167): Elsevier
- Wu H, Qian H, Chen J, Huo C. Assessment of agricultural drought vulnerability in the Guanzhong Plain. China Water Resources Management. 2017; 31 (5):1557–1574. doi: 10.1007/s11269-017-1594-9. [ CrossRef ] [ Google Scholar ]
- Xie W, Huang J, Wang J, Cui Q, Robertson R, Chen K (2018) Climate change impacts on China’s agriculture: the responses from market and trade. China Econ Rev
- Xu J, Sharma R, Fang J, Xu Y. Critical linkages between land-use transition and human health in the Himalayan region. Environ Int. 2008; 34 (2):239–247. doi: 10.1016/j.envint.2007.08.004. [ PubMed ] [ CrossRef ] [ Google Scholar ]
- Yadav MK, Singh R, Singh K, Mall R, Patel C, Yadav S, Singh M. Assessment of climate change impact on productivity of different cereal crops in Varanasi. India J Agrometeorol. 2015; 17 (2):179–184. doi: 10.54386/jam.v17i2.1000. [ CrossRef ] [ Google Scholar ]
- Yang B, Usman M. Do industrialization, economic growth and globalization processes influence the ecological footprint and healthcare expenditures? Fresh insights based on the STIRPAT model for countries with the highest healthcare expenditures. Sust Prod Cons. 2021; 28 :893–910. [ Google Scholar ]
- Yu Z, Razzaq A, Rehman A, Shah A, Jameel K, Mor RS (2021) Disruption in global supply chain and socio-economic shocks: a lesson from COVID-19 for sustainable production and consumption. Oper Manag Res 1–16
- Zarnetske PL, Skelly DK, Urban MC. Biotic multipliers of climate change. Science. 2012; 336 (6088):1516–1518. doi: 10.1126/science.1222732. [ PubMed ] [ CrossRef ] [ Google Scholar ]
- Zhang M, Liu N, Harper R, Li Q, Liu K, Wei X, Liu S. A global review on hydrological responses to forest change across multiple spatial scales: importance of scale, climate, forest type and hydrological regime. J Hydrol. 2017; 546 :44–59. doi: 10.1016/j.jhydrol.2016.12.040. [ CrossRef ] [ Google Scholar ]
- Zhao J, Sinha A, Inuwa N, Wang Y, Murshed M, Abbasi KR (2022) Does Structural Transformation in Economy Impact Inequality in Renewable Energy Productivity? Implications for Sustainable Development. Renew Energy 189:853–864. 10.1016/j.renene.2022.03.050
Thank you for visiting nature.com. You are using a browser version with limited support for CSS. To obtain the best experience, we recommend you use a more up to date browser (or turn off compatibility mode in Internet Explorer). In the meantime, to ensure continued support, we are displaying the site without styles and JavaScript.
- View all journals
- My Account Login
- Explore content
- About the journal
- Publish with us
- Sign up for alerts
- Open access
- Published: 26 February 2024
Reassessment of the risks of climate change for terrestrial ecosystems
- Timo Conradi ORCID: orcid.org/0000-0003-2360-9284 1 ,
- Urs Eggli ORCID: orcid.org/0000-0002-8842-8188 2 ,
- Holger Kreft ORCID: orcid.org/0000-0003-4471-8236 3 , 4 , 5 ,
- Andreas H. Schweiger ORCID: orcid.org/0000-0003-2656-5918 6 ,
- Patrick Weigelt ORCID: orcid.org/0000-0002-2485-3708 3 , 4 , 5 &
- Steven I. Higgins ORCID: orcid.org/0000-0001-5695-9665 1
Nature Ecology & Evolution ( 2024 ) Cite this article
14k Accesses
102 Altmetric
Metrics details
- Biogeography
- Climate-change ecology
Forecasting the risks of climate change for species and ecosystems is necessary for developing targeted conservation strategies. Previous risk assessments mapped the exposure of the global land surface to changes in climate. However, this procedure is unlikely to robustly identify priority areas for conservation actions because nonlinear physiological responses and colimitation processes ensure that ecological changes will not map perfectly to the forecast climatic changes. Here, we combine ecophysiological growth models of 135,153 vascular plant species and plant growth-form information to transform ambient and future climatologies into phytoclimates, which describe the ability of climates to support the plant growth forms that characterize terrestrial ecosystems. We forecast that 33% to 68% of the global land surface will experience a significant change in phytoclimate by 2070 under representative concentration pathways RCP 2.6 and RCP 8.5, respectively. Phytoclimates without present-day analogue are forecast to emerge on 0.3–2.2% of the land surface and 0.1–1.3% of currently realized phytoclimates are forecast to disappear. Notably, the geographic pattern of change, disappearance and novelty of phytoclimates differs markedly from the pattern of analogous trends in climates detected by previous studies, thereby defining new priorities for conservation actions and highlighting the limits of using untransformed climate change exposure indices in ecological risk assessments. Our findings suggest that a profound transformation of the biosphere is underway and emphasize the need for a timely adaptation of biodiversity management practices.
Similar content being viewed by others
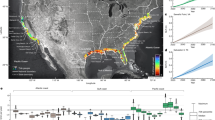
Disappearing cities on US coasts
Leonard O. Ohenhen, Manoochehr Shirzaei, … Robert J. Nicholls
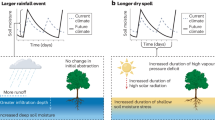
Plant responses to changing rainfall frequency and intensity
Andrew F. Feldman, Xue Feng, … Benjamin Poulter
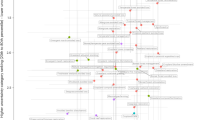
Expert review of the science underlying nature-based climate solutions
B. Buma, D. R. Gordon, … S. P. Hamburg
Global circulation models (GCM) forecast strong climatic changes throughout the twenty-first century under all but the most optimistic greenhouse gas emission scenarios 1 . The anticipated climatic changes are expected to force both continuous and abrupt changes in the distribution of ecosystems and species 2 , 3 . One implication is that ecosystem managers may have to shift their focus from targeting predefined baseline states to managing ecosystem change along trajectories forced by climatic change 4 , 5 , 6 . However, the strength of climatic forcing and the direction of the new trajectories are uncertain, making it difficult for ecosystem managers to define and implement targeted actions 7 , 8 . Not only are climates changing but it is also likely that climate states without present-day analogues (hereafter, novel climates) will emerge and that some of the existing climate states will disappear (hereafter, disappearing climates) 9 , 10 . It has been suggested that disappearing climates may increase the risk of losing species and some types of ecosystems, whereas novel climates may lead to the formation of novel ecosystems 9 , 11 , 12 . Since the functioning of novel ecosystems is by definition unknown, their emergence would further enhance the risk of ecosystem management failure 9 , 12 , 13 . It is thus a research priority to identify regions where climate change is likely to force strong ecological change, so that ecosystem managers can implement timely and targeted actions.
To identify regions with elevated ecological risks from climate change, previous works analysed the exposure of the global land surface to potentially dangerous climatic changes 10 . This includes analyses of the exposure of ecosystems to strong climatic changes and globally novel climates, as well as the disappearance of existing climate states 9 , 14 . In these studies, exposure was calculated as the Euclidean distance between ambient and future climates, standardized by the historical variability in climate variables. Another widely used climate change exposure metric is climate change velocity 15 , 16 , 17 , which quantifies the displacement rate of climate states and a more recent species-focused study identified where and when animal species will be exposed to temperature and precipitation conditions outside their realized niches 3 .
However, risk assessments based on climate change exposure indices oversimplify an organism’s perception of climate exposure. Previous climate change exposure work did not consider that physiological and ecological responses to changing climatic factors are often nonlinear and colimited by several climatic factors 18 , 19 , 20 , 21 and that hierarchies of colimiting climatic factors are expected to reorganize as climate change progresses 22 , 23 . This means that the risk of a one unit increase in a climatic factor is not comparable across locations with different baseline values and is contingent on concomitant changes in colimiting climatic factors. Neither this baseline effect nor the colimitation effect are accounted for by climate change exposure studies. Moreover, individual species and growth forms exhibit contrasting climatic preferences 24 and are therefore likely to respond differently to projected climatic changes. The implication is that the ecological response to altered climatic conditions is unlikely to map perfectly to climate change exposure indices 9 , 25 , which makes the ecological interpretation of climate change exposure indices problematic.
Problems with the ecological interpretation of exposure indices could be addressed by using process-rich ecosystem simulation models 26 , 27 . Such models are explicitly designed to model the impacts of climatic changes on ecosystems. However, ecosystem simulation models are hampered by process and parameter uncertainty 26 , 27 , 28 , evidenced by large discrepancies between outputs of different models forced with the same climate data 29 . The implication is that current approaches to understand ecological climate change risks and impacts trade-off the certainty with which we can make predictions with the ability to ecologically interpret those predictions. Exposure indices have more tractable prediction uncertainty, yet are difficult to interpret ecologically, whereas the opposite is true for ecosystem models.
To reduce this trade-off, we computed a phytoclimatic transform of ambient (mean of 1979–2013) and future (mean of 2061–2080; henceforth 2070) climatologies. The phytoclimatic transform expresses the climate of a grid cell in terms of its suitability for species of 14 plant growth forms that define terrestrial ecosystems. The transformation was based on an existing protocol 24 (Supplementary Fig. 1 ) and involved (1) parameterizing an ecophysiological plant growth model forced with monthly climate data for 135,153 vascular plant species, (2) using the fitted species models to identify climatically suitable grid cells for each species, (3) calculating the proportion of species of each growth form for which a grid cell is climatically suitable and using this proportion as an index of the climatic suitability of a grid cell for a growth form (Fig. 1 ). We refer to the vector of the 14 growth-form suitabilities of a grid cell as its phytoclimate. That is, rather than describing the climate of a location by climate variables such as mean annual temperature or annual rainfall, we describe the climate by its ability to support species of different types of plants that ecologists use to define terrestrial ecosystems. This provides a means to understand which structural changes in ecosystems the future climatic states will promote. For instance, should the climatic suitability of a grid cell for cold-deciduous trees change from 0.2 to 0.3, this would mean that the climate can now accommodate an extra 10% of species of this growth form in that grid cell, which raises the potential for species of this growth form to become more frequent.
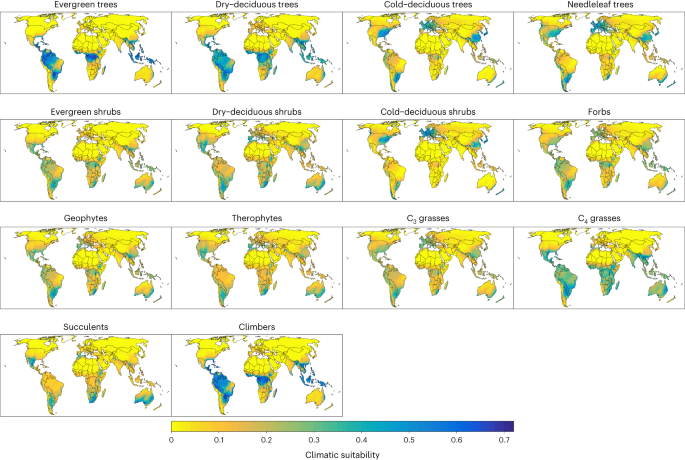
Suitability is expressed as the proportion of plant species of a growth form for which the climate of the cells is suitable according to an ecophysiological plant growth model. The median number of modelled species per growth form was 5,249, with a minimum of 439 (needleleaf trees) and a maximum of 24,853 (evergreen shrubs) species. The total number of modelled species was 135,153.
The premise of this analysis is that we can characterize changes in the climatic forcing of ecosystems by analysing shifts in climatic suitability for the growth forms that define ecosystems. Previous work showed that the growth-form suitability values are useful predictors of ecosystem states 30 , suggesting that shifts in growth-form suitability may be useful indicators of the changing climatic forcing. It should be emphasized that the growth-form suitability may be quite different from growth-form abundances because other ecological processes such as biotic interactions, dispersal or disturbances, determine whether physiological suitability can predict abundance. Our analysis therefore provides a physiologically informed assessment of where and how the climatic drivers of ecosystem assembly are changing.
The plant growth model used here describes how the uptake and allocation of carbon and nitrogen of an individual plant is colimited by monthly temperature, soil moisture, solar radiation and atmospheric CO 2 concentrations 24 , 31 , 32 . We used species distribution data from the BIEN database ( https://bien.nceas.ucsb.edu/bien/ ) to find the physiological parameters of the plant growth model that best explain the observed distribution of a species. For each of the 135,153 species, the parameterized model is then run forward using a monthly gridded climatology to simulate the biomass accumulation of the species in the cells of a global grid and the simulated biomass values are used as the linear predictor in a logistic regression model that predicts whether grid cells are climatically suitable for that species or not. That is, we use data on the observed distribution of species to estimate the parameters of a model that articulates a simplified physiological niche of a plant species 32 . The performance of the model in transferability tests 33 indicates that this physiological niche characterization is predictive of where a plant species could grow.
The growth-form suitability surfaces in Fig. 1 represent a summary of estimated physiological niches of 135,153 plant species grouped by growth form. We aggregate this phytoclimatic transform of the climate by identifying groups of cells with similar growth-form suitability using unsupervised classification. The geographical projection of these groups reveals phytoclimatic zones of the Earth (Fig. 2a ) and provides a plant growth-form centric classification of the climates of the Earth. Supplementary Table 1 and Supplementary Fig. 2 provide overviews of the mean growth-form suitabilities of the ambient phytoclimatic zones. Supplementary Fig. 3 shows that the phytoclimatic zones have distinct climatic characteristics.
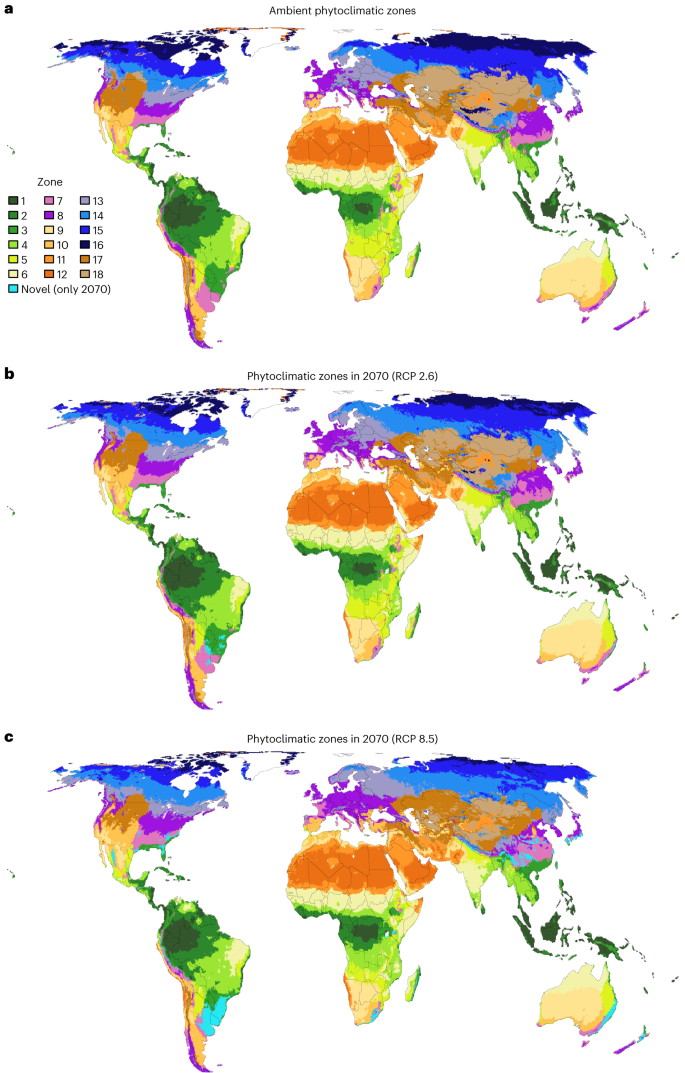
Phytoclimatic zones have internally similar climatic suitability for different plant growth forms. a , Ambient phytoclimatic zones, derived from an unsupervised classification of grid cells by their climatic suitability for 14 plant growth forms (Fig. 1 ). b , c , The arrangement of phytoclimatic zones in 2070 under RCP 2.6 ( b ) and RCP 8.5 ( c ). The median climatic suitabilities for each growth form across five future climatologies were used to determine the future phytoclimatic zone of the grid cells for each RCP. Cells shown as ‘novel’ are projected to have growth-form suitability realizations without present-day analogue. Extended Data Fig. 5 shows shifts in phytoclimatic zones for different combinations of RCP and GCM.
The phytoclimatic zones depicted in Fig. 2a represent areas where the climate supports similar plant types, making the concept closely related to biomes. Indeed, previous work suggested that phytoclimatic zones could be used to define biomes 24 . Yet, biomes are mostly defined as regions where specific combinations of plant growth forms have developed in response to the regional climate 34 , 35 . That is, biomes implicitly consider additional ecological processes that shape the ecosystem structure observed in the field, whereas phytoclimatic zones consider only how the climate influences the physiological performance of plant types. This means that phytoclimatic zones (for example, Fig. 2 ) are more closely affiliated with bioclimatic zones 36 , 37 than with biomes.
The phytoclimatic transformation was then applied to climatologies projected for 2070 under the representative concentration pathways RCP 2.6 and RCP 8.5, which describe a reduced and a high emission scenario, respectively. Specifically, we forced the fitted species growth models with the future climatologies to project climatic suitability maps for each species and grouped species by growth form to calculate growth-form suitability maps for 2070 (Extended Data Figs. 1 and 2 ).
Analogous to work on climate change exposure 9 , we used the ambient and future growth-form suitability maps to calculate three multivariate Euclidean distances that summarize components of ecological risk: (1) The local phytoclimatic change each grid cell will be exposed to, estimated as the distance between the ambient and future growth-form suitabilities of each grid cell. This index summarizes the changing climatic constraints on the plant growth forms in each cell. (2) The novelty of the future phytoclimatic state of each grid cell relative to ambient phytoclimatic states, estimated as the minimum distance between the future growth-form suitabilities of a grid cell and that of an ambient grid cell. A novel phytoclimate is thus a climate that constrains plant growth forms differently to any ambient climate state. (3) The disappearance of the ambient phytoclimatic state of each grid cell, estimated as the minimum distance between the ambient growth-form suitabilities of a grid cell and those of a future grid cell. The disappearing phytoclimate index is thereby a measure of how distinct the ambient phytoclimatic state of a grid cell is relative to future phytoclimatic states. A disappearing phytoclimate indicates disappearance of a way in which an ambient climate constrains plant growth forms.
To interpret which values of the three indices indicate high risks, we use the Euclidean distances between the phytoclimatic zones shown in Fig. 2a as a reference. Specifically, we computed the centroids of each zone in Euclidean growth-form suitability space and calculated the pairwise Euclidean distances between these centroids. The 5th percentile of these intercentroid distances was used as a threshold value to identify ecologically significant risk equivalent to a shift between some of the phytoclimatic zones. Lastly, the future phytoclimatic zone of grid cells was projected by assigning grid cells to the zone of the closest ambient grid cell in Euclidean growth-form suitability space; grid cells that were further than the threshold distance from ambient phytoclimatic states were designated as novel.
Our analysis predicts substantial change in phytoclimates by 2070 (Fig. 3a ). If anthropogenic emissions follow RCP 2.6, 33% of the Earth’s terrestrial surface (excluding the currently ice-covered parts of Greenland and Antarctica) will experience an ecologically significant change in the extent to which the climate can support different plant growth forms. The fraction of land with significant change in phytoclimate increases to 68% if emissions follow RCP 8.5. When calculating the median change value across five projections that used climatologies from different GCMs, the most pronounced phytoclimatic changes are likely to occur in the mountain regions of south China, the Himalayas, northwestern Russia, the Baltic countries, Scandinavia, the southeastern and northeastern United States, Alaska, central Mexico, the tropical Andes, southeastern South America, southeastern Australia and northern New Zealand. Projections based on each GCM are shown in Extended Data Figs. 3a and 4a .
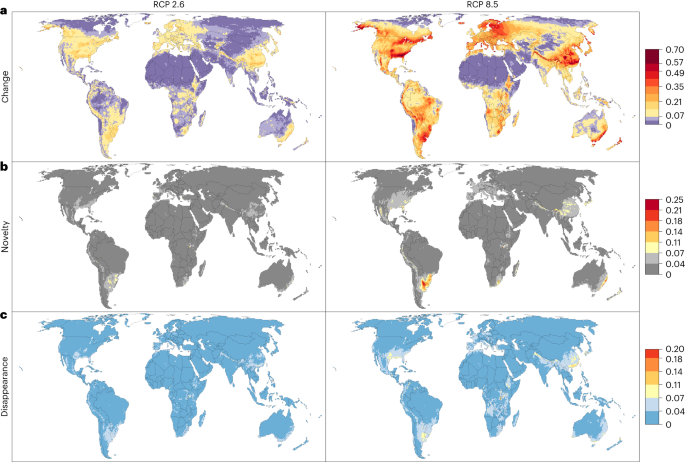
The phytoclimate of the grid cells is the suitability of the local climate for 14 plant growth forms that characterize the structure of terrestrial ecosystems. a , Local change in phytoclimate, expressed as the Euclidean distance between the ambient and future phytoclimates of a cell. b , Novelty of the projected phytoclimate in 2070, expressed as the Euclidean distance of the future phytoclimate of a cell to its closest analogue in the global pool of ambient phytoclimates. c , Risk of disappearance of the existing phytoclimate, expressed as the Euclidean distance of the ambient phytoclimate of a cell to its closest analogue in the global pool of future phytoclimates. In a – c , RCP 2.6 is shown in the left column and RCP 8.5 in the right column; the colour bars are scaled so that yellow to red colours indicate significant values of change, novelty and disappearance, respectively (see main text for the definition of the threshold value and Supplementary Figs. 4 – 6 for the sensitivity of the results to varying the threshold value). Values are medians across future climatologies generated by five different GCMs for each RCP.
The changes in local phytoclimates translate into widespread shifts of phytoclimatic zones (Fig. 2b,c ). Temperate, boreal and polar regions are most strongly affected by these shifts (Supplementary Fig. 7 ). Under RCP 8.5, large parts of zones 13 and 14, which currently support cool-temperate and hemiboreal ecosystems, will shift polewards, as will zone 15, which currently supports boreal ecosystems. Zone 16, which currently supports tundra ecosystems, will be reduced by 72% as it cannot shift further north. This prediction is consistent with observations of more frequent recruitment of shrubs and trees in tundra 38 , 39 , 40 , 41 and increasing tree density at the forest–tundra ecotone 42 . Zone 18, which supports ecosystems of very continental cool-temperate regions will lose 54% of its current extent, mostly to zone 17, which favours less cold-limited continental cool-temperate ecosystems (Supplementary Fig. 3 ) and is generally more suitable for most growth forms (Supplementary Fig. 2 ). Tropical phytoclimatic zones are overall less likely to change in position and extent. However, some regions will be exposed to changes in phytoclimatic zones that may force strong structural changes in ecosystems. For instance, we project that the southeastern and eastern parts of the Amazon will shift to a phytoclimatic zone which supports savanna and some types of drier forest ecosystems (phytoclimatic zone 4 in Fig. 2 ). This projection is consistent with analyses that have used hydrological thresholds to project future changes in South American forests and savannas 43 . Phytoclimatic zone 4 is predicted to advance into the Amazon irrespective of GCM, albeit to very different degrees (Extended Data Fig. 5 ), suggesting that the future phytoclimatic status of the Amazon region is highly uncertain (Extended Data Fig. 6 ).
Despite the strong changes in local phytoclimates and substantial shifts in phytoclimatic zones, most of the future phytoclimates will have a present-day analogue. Specifically, only 0.3% (RCP 2.6) to 2.2% (RCP 8.5) of land cells will exhibit novel phytoclimates (Fig. 2b,c ). The highest novelty indices are forecast in southeastern South America and Australia (Fig. 3b ). The novel phytoclimates are forecast to primarily emerge in what at present are mesic subtropical climates typical of the eastern sides of continents but they are also likely in tropical and subtropical mountain ranges such as the Andes, the Himalayas and the Sierra Madre Occidental. Most grid cells in the South American and Australian novelty centres belong to the ambient phytoclimatic zone 7, which is characterized by relatively high suitability for all growth forms (Supplementary Fig. 2 ). These cells are predicted to exhibit a strongly increased climatic suitability for evergreen trees, dry-deciduous trees and climbers by 2070 but reduced suitability for needleleaf trees, cold-deciduous shrubs and cold-deciduous trees (Extended Data Figs. 7 and 8 ). The emerging phytoclimatic novelty in these cells may be induced by slightly increasing rainfall combined with similar temperature seasonality, albeit with hotter summers and milder winters. It is likely that this combination reduces the cold-limitation of the numerous species of woody growth forms with preference for mesic tropical and subtropical climates in our analysis, whilst the elevated temperatures disfavour many of the needleleaf and cold-deciduous woody species with preference for temperate conditions (Fig. 1 ).
Previous analyses of climatic variables found that the highest climatic novelty will emerge in the warmest of the ambient climates in the tropics 9 , 10 . Our analyses of phytoclimates suggest that these novel tropical climates are not functionally novel. For instance, although parts of the Sahara will be exposed to novel climates 9 , 10 , we predict that these novel climates will remain highly unsuitable for all plant growth forms, which means that the novel climates are not novel from a plant functional perspective. In the wet tropics where decreases in rainfall and higher temperatures are projected, the novel climates may promote more drought-adapted species, yielding growth-form suitability combinations (phytoclimates) already observed elsewhere. For example, the Amazon and parts of the Cerrado are novel climate regions 9 , 10 , yet we predict that phytoclimates currently realized in the Cerrado are advancing in the Amazon and are themselves partially replaced by phytoclimates currently realized in the Caatinga (Fig. 2c ), meaning that no novel phytoclimates emerge in this region.
Only 0.1% (RPC 2.6) to 1.3% (RPC 8.5) of ambient phytoclimates are projected to disappear by 2070 (Fig. 3d ). As with novelty, the disappearance of phytoclimates is forecast to occur primarily in the mesic subtropical climates on the eastern sides of the continents and there was good agreement between the five projections per RCP on where novel and disappearing phytoclimates are located (Extended Data Figs. 3 b,c and 4b,c ). Disappearing phytoclimates were seldom in the same grid cells that were predicted to support novel phytoclimates. For instance, using the threshold applied in Fig. 3 , only 25% of the grid cells with a disappearing phytoclimate were projected to be operating under a novel phytoclimate in 2070, whereas 75% of the cells with disappearing phytoclimates will be operating under a phytoclimate that is found elsewhere today.
The spatial resolution of this analysis can hide details of the geography of changing phytoclimates. While we fitted the species growth models using climatic forcing data with 1 km spatial resolution, our global analysis of phytoclimates used a grid with 25 km spatial resolution. This aggregation removes the (phyto)climatic heterogeneity within the 25 km cells, preventing the detection of finer phytoclimatic patterns in topographic heterogeneous areas such as mountain ranges. For example, the alpine tundra of the European Alps is not visible on our map of phytoclimatic zones (Fig. 2a ). A higher resolution would allow the presence and dynamics of such micro-phytoclimates to be projected.
Moreover, while we explored uncertainty originating from RCP and GCM (Extended Data Figs. 3 – 6 ), we did not explore uncertainty originating from the model used to estimate climatic suitability for the individual plant species. Our growth-form suitability projections are therefore partly contingent on the assumptions of the growth model used to estimate species-level suitability. While in principle any suitability or species distribution model could be used in the workflow, in practice, many existing alternative models may be inappropriate for this task. First, correlative suitability models have limited transferability 33 , 44 , producing implausible growth-form suitability surfaces 24 , whereas the process-based TTR-SDM used here was successful in model transferability tests 33 and produces plausible suitability surfaces 24 , 30 . Second, alternative models do not consider how elevated atmospheric CO 2 concentrations may influence photosynthesis and thereby species ranges. Third, sensitivity analyses (Supplementary Fig. 8 ) show that robust estimates of growth-form suitability surfaces require of the order of hundreds of species distribution models, meaning that only process-based models which can be calibrated for many species are suitable for this task. On another level, uncertainty in the species distribution model needs to be evaluated in the context of other sources of uncertainty, such as uncertainty in the occurrence data, the assignment of species to growth-form classes and the climatic forcing data used to calibrate the models and to project them into the future.
Global greenhouse gas emissions are currently tracking the RCP 8.5 scenario 45 . Under this scenario, our analysis predicts that almost the entire vegetated land surface will be subject to substantial changes in how climate supports the different types of plants that define terrestrial ecosystems (Fig. 3a ). This is likely to impact on ecosystem structure, functioning and dynamics. For example, successional trajectories may no longer follow the usual sequences 21 , 46 , 47 and some undesired growth forms may increase in abundance 48 , complicating ecosystem restoration and management. The prospect of changes in ecosystem structure and functioning supports growing calls for ecosystem managers to realign their goals and practices 4 , 5 , 6 . For instance, conservation management in protected areas often seeks to retard change by removing invading species but, if climatic forcing threatens the baseline states, actions that enhance the ability of ecosystems to track climatic forcing may be more effective 5 , 6 . Tracking climatic forcing would require that managers plan actions that ensure that locally declining species can reach suitable locations elsewhere, for instance species translocations 49 or enhancing the permeability of agricultural landscapes 50 . Management actions that aim to retard change need to be carefully planned since such actions, if not sustained, will be a waste of resources 51 . Similarly, in ecological restoration it is common to use predefined baseline states as target points 52 but these targets will become increasingly unattainable as climate deviates from domains that allow the targeted baseline states 47 . Our analysis can be used as a guide to identify locations where future climates will not support current ecosystem states and where managers could consider switching from preservation to managing the trajectories of change 5 , 6 . Our projections of shifts in phytoclimatic zones (Fig. 2 ) may serve to reduce uncertainty about these trajectories because they show which type of phytoclimate is expected in a locale in the future and where experience with managing ecosystems under such a phytoclimate may already exist.
Our analysis predicts how the climatic forcing of ecosystems is changing but other factors, not considered in our analysis, will influence the extent and rate at which ecosystems follow the trajectories of climatic forcing. In particular, disturbances or dieback of late-successional vegetation can accelerate change in ecosystems 21 . Other factors, such as dispersal limitation 53 , priority effects of resident communities 54 , persistence of declining remnant populations 55 or microclimatic buffering by tree canopies 56 can cause ecosystems to lag behind the climatic forcing, leading to a disequilibrium between climate forcing and ecosystem state. An ecosystem in strong disequilibrium with climate is, however, at high risk of sudden and potentially undesired transitions when the barriers to change have been overcome 32 , underlining that it may be prudent to proactively manage the change in ecosystems to reduce risks associated with mismatches between climate forcing and ecosystem states. This could be achieved by various means including assisted migration, increasing landscape connectivity 49 , 50 , 57 and rewilding 58 , 59 . All of these require authentic shifts in management paradigms, which may be warranted given that most of the land surface will, according to our analysis, be subject to fundamental changes in climatic forcing.
Novel phytoclimates also have implications for ecosystem management. Novelty in our analysis emerges when the climate supports plant growth forms in unprecedented ways. The emergence of novel phytoclimates confronts biodiversity managers with deeper uncertainty on how ecosystems respond to management actions 13 and how the structure and function of the ecosystems may change. That is, where novel phytoclimates emerge, managers cannot rely on experience gained by managers elsewhere. The palynological record indicates that no-analogue climates in the last late-glacial period supported vegetation formations without a present-day analogue 60 , suggesting that the novel phytoclimates of the future may, as they have done in the past, give rise to novel ecosystems.
The interpretation of disappearing phytoclimates is that some of the ways in which climate currently supports plant growth forms will not be realized in the future. As these phytoclimates disappear, there is potential for the ecosystem types that form under these phytoclimates to disappear. It follows that regions in which we project disappearing phytoclimates are high-risk areas for biodiversity loss because it is unlikely that these ecosystem states can assemble or be restored elsewhere. We found a coherent pattern in the distribution of disappearing and novel phytoclimates, suggesting priority areas for conservation monitoring and action.
The hotspots of phytoclimatic change, novel phytoclimate emergence and phytoclimate disappearance predicted in this study (Fig. 3 ) differ clearly from regions of analogous climatic changes identified in previous global studies 9 , 10 . These studies used climate system variables of broad ecological relevance that were standardized against their interannual variability in the time period covered by the ambient climatology. This standardization emphasizes changes in variables that are large relative to their historical variability because such changes are assumed to have stronger ecological effects and tend to upweight changes in temperature over changes in precipitation since the interannual variability of temperature is often smaller 9 . Our approach, by contrast, uses an ecophysiological growth model to transform monthly climate variables into estimates of climatic suitability for individual species and, by grouping species into growth forms, the climatic suitability for the plant growth forms defining terrestrial ecosystems. That is, the ecological effect of temporal changes in climate variables is prescribed by the fitted plant growth models and summarized in the future growth-form suitability surfaces. This phytoclimatic transformation explains why tropical regions, which are predicted to produce novel climates due to warmer temperatures 9 , 10 , are not predicted to produce novel phytoclimates as warming proceeds. Specifically, as warming proceeds, the suitability for all growth forms is forecast to decline in the equatorial regions (Extended Data Figs. 7 and 8 ) and the phytoclimate in these regions will become similar to phytoclimatic zones supporting more drought-adapted and seasonal ecosystems which already exist elsewhere (Fig. 2b,c ).
Global greenhouse gas emissions are currently tracking RCP 8.5 predictions 45 under which severe climatic changes are forecast 1 . Our analysis suggests that these climatic changes are likely to force a profound transformation of the biosphere, ensuring that substantial adaptation measures will be necessary for biodiversity conservation, agriculture and forestry. Our findings, however, also show that changes to the biosphere would be considerably milder under RCP 2.6, which supports the view that cutting greenhouse gas emissions would fundamentally reduce climate change risks for biodiversity, ecosystem functioning and agricultural production.
Environmental data
The plant growth model (described below) is forced with data on monthly minimum, mean and maximum temperature, monthly soil moisture, monthly solar radiation and atmospheric CO 2 concentrations. Nitrogen uptake is simulated as a function of temperature, soil moisture and soil nitrogen content 32 . In this application of the model we assumed the same soil nitrogen content in all grid cells, which means that plant nitrogen uptake in the model is influenced by temperature and soil moisture only. This is appropriate because this analysis aims to estimate the climatic suitability of geolocations. Ambient monthly temperature data (averages for the period 1979–2013) were downloaded from the CHELSA database 61 v.1.2. Solar radiation data were downloaded from the Global Aridity and PET Database v.1 ( https://csidotinfo.wordpress.com/data/global-aridity-and-pet-database/ ). We developed a soil moisture model that is similar to that of the Global Aridity and PET Database and predicts monthly soil moisture on the basis of monthly values of precipitation, solar radiation, minimum, mean and maximum temperature and soil field capacity and wilting point, using a Hargreaves-type model of monthly potential evapotranspiration. Ambient monthly precipitation data (averages for the years 1979–2013) were taken from CHELSA v.1.2 and soil field capacity and wilting point data from the Global Gridded Surfaces of Selected Soil Characteristics (IGBP-DIS) dataset 62 . The modelled soil moisture values reflect soil moisture available for evapotranspiration and are not influenced by the vegetation of a grid cell. For model fitting, we assumed an ambient atmospheric CO 2 concentration of 338 ppm (ref. 63 ). All environmental data were resampled to 1 km resolution if necessary and projected to the equal area World Eckert IV projection, which was used in all analyses and maps.
We downloaded ten downscaled Coupled Model Intercomparison Project Phase 5 (CMIP5) temperature and precipitation climatologies for 2070 (averages for the period 2061–2080) projected by five CMIP5 GCMs under two emission scenarios (RCP 2.6 and 8.5) from CHELSA v.1.2. The five GCMs were: CCSM4 (ref. 64 ), CNRM-CM5 (ref. 65 ), FGOALS-g2 (ref. 66 ), MIROC-ESM (ref. 67 ) and MPI-ESM-LR (ref. 68 ). These models were chosen to represent a wide range of uncertainty in climate change projections originating from different GCMs 69 . Future monthly soil moisture was predicted with our soil moisture model for each of the ten climatologies for 2070. Solar radiation in 2070 was assumed to be the same as today. We focus on RCP 2.6 and RCP 8.5 because the former represents an optimistic emissions reduction scenario whereas the latter represents a pessimistic scenario that assumes continued growth in emissions 70 and is the scenario the world is currently tracking most closely 45 . We assumed atmospheric CO 2 concentrations in 2070 of 438 ppm and 677 ppm for RCP 2.6 and RCP 8.5, respectively 63 .
We classified the grid cells of a global map into 20 environmental zones based on the ambient monthly environmental forcing data used by the plant growth model (Supplementary Fig. 9 ). We used the clara algorithm in the cluster package in R to classify the cells and optimized the separation of cells into the 20 clara clusters by means of a discriminant analysis of principal components (DAPC) 71 computed from the environmental data. The resulting environmental zones were used later for generating stratified samples of species presence and pseudo-absence records that were used to fit the plant growth model.
Species distribution and growth-form data
We downloaded distribution data of all vascular plant species in the BIEN database v.4.1 ( http://bien.nceas.ucsb.edu/bien/ ), using the BIEN R package 72 . We used the non-public version of BIEN, which contains sensitive occurrence data of endangered species not included in the public BIEN version and was made available to us upon request.
Most occurrence records in BIEN come from herbarium collections (see Acknowledgments section for collections used in this analysis), ecological plots and surveys 73 , 74 as well as from plant trait observations. BIEN also includes data from NeoTropTree ( http://www.neotroptree.info/ ), RAINBIO ( http://rainbio.cesab.org/ ), TEAM ( https://www.wildlifeinsights.org/team-network ) and The Royal Botanical Garden of Sydney, Australia ( https://www.rbgsyd.nsw.gov.au/ ) and plot data from CVS, CTFS, FIA, NVS, SALVIAS, TEAM, VEGBANK and MADIDI ( https://bien.nceas.ucsb.edu/bien/data-contributors/all/ ). A full list of references for BIEN occurrence records used in this study can be found in Supplementary Table 2 .
The R package CoordinateCleaner v.2.0–11 (ref. 75 ) was used to remove records with either zero longitude or latitude and records within a buffer of 10 km around country and province centroids, 5 km around country capitals and 200 m around biodiversity institutions (herbariums, museums or universities), respectively. In addition, we computed country centroids from the Database of Global Administrative Areas v.3.4 and removed records within a 50 km buffer to these centroids. We only retained one occurrence record per 1 km grid cell and species.
The species were grouped into 14 growth forms: evergreen broadleaf trees and shrubs, cold-deciduous and drought-deciduous broadleaf trees and shrubs, needleleaf trees, C 3 and C 4 grasses, forbs (excluding geophytes and therophytes), geophytes, therophytes, terrestrial succulents and climbers. All Pinales, except Gnetales and Podocarpaceae, were classified as needleleaf trees, that is all Araucariaceae, Cephalotaxaceae, Cupressaceae, Pinaceae, Sciadopityaceae and Taxaceae. All species of the Poales families Poaceae, Cyperaceae, Juncaceae, Anarthriaceae, Centrolepidaceae, Ecdeiocoleaceae, Joinvilleaceae, Restionaceae, Thurniaceae and Typhaceae were classified as grasses. The database of ref. 76 was then used to identify grass species with C 4 photosynthetic pathway. We classified all species listed in the Illustrated Handbook of Succulent Plants as succulents 77 , with updated lists for monocotyledons 78 and Cactaceae 79 . Woody succulents were treated as succulents and not as trees or shrubs.
For all remaining species, we extracted information on growth form and leaf phenology from BIEN and GIFT v.2.1 (ref. 80 ). We first searched for species-level information in BIEN and filled the gaps with species-level information in GIFT. If growth form and phenology data were still missing for a species, we searched for genus-level information in BIEN and filled the gaps with genus-level information in GIFT. If data were still missing after this step, we searched for family-level information in BIEN and filled the gaps with family-level information in GIFT. If several entries with contrasting information were available (for example, a species had entries as a shrub and a tree), we used the most frequent entry. We assigned species classified in BIEN and GIFT as herb or fern to the forb category. Species classified as graminoids by BIEN and GIFT were also classified as forbs by us, unless they belonged to one of the Poales mentioned above. The forbs were then split into geophytes, therophytes and other forbs using life-form information in GIFT. We assigned species classified in BIEN as climber, liana or vine to the climber category, as well as species classified in GIFT as obligatory climbers, liana or vine. Palms were treated as trees. We excluded tree and shrub species with leaf phenology entries ’variable’ (GIFT) or ‘variable or conflicting information’ (BIEN), aquatic species and all epiphytes (including succulent epiphytes) because they do not use soil moisture and thus cannot be modelled with our approach (GIFT includes a comprehensive global checklist of vascular epiphytes 81 that was used to identify epiphytes). Cold-deciduousness and drought-deciduousness of trees and shrubs were determined by plotting the BIEN distribution records on a world map of Köppen–Geiger climates 82 . Species with >50% of records in cold climates (Köppen–Geiger zones Cf, D and E) were defined as cold-deciduous and the remaining species were assumed to be drought-deciduous. References for BIEN growth-form data can be found in Supplementary Table 3 .
Name matching between data sources was accomplished with the Taxonomic Name Resolution Service 83 , which uses the Missouri Botanical Garden’s Tropicos database ( https://tropicos.org ), The Plant List (http://www.theplantlist.org, now inactive) and the USDA Plants Database ( http://plants.usda.gov ).
Growth and species distribution modelling
We used the TTR-SDM 32 , an ecophysiological species distribution model for plants, to identify climatically suitable grid cells for each plant species. The TTR-SDM is based on Thornley’s transport resistance (TTR) model 31 , which describes in a series of dynamic equations how the biomass accumulation of an individual plant is influenced by the uptake of carbon and nitrogen, their allocation between sinks and sources and growth processes. The TTR-SDM 32 includes a series of functions that describe how the resource uptake (carbon and nitrogen) and growth processes in the TTR model are influenced by monthly minimum, mean and maximum temperature, soil moisture, solar radiation, soil nitrogen and atmospheric CO 2 concentration. Supplementary Fig. 10 provides a graphical representation of how these environmental forcing variables influence the resource uptake and growth rates in the TTR-SDM. The model’s equations prescribe the general shape of these relationships (trapezoidal or saturating); however, the values of the forcing variables at which the physiological rates change are species-specific and are estimated for each plant species separately; these values are the model’s parameters ( n = 18; Supplementary Fig. 10 ) and we estimate them from species distribution data as described further below.
The model’s equations define that each of the forcing variables can colimit a plant’s resource uptake and growth analogous to Liebig’s law of the minimum and that allocation of the assimilated resources between resource sources and sinks is driven by transport resistance processes 32 . The model is run on a monthly time step using the ambient and the 2070 monthly climatology, respectively, which allows it to explicitly simulate how monthly fluctuations in the forcing variables colimit a plant’s resource uptake, allocation and growth. That is, the model simulates that the monthly biomass accumulation of a plant is colimited by the temperature, soil moisture, solar radiation and soil nutrients that a plant is exposed to each month of a simulation 33 .
The TTR-SDM version used here uses a Farquhar-type photosynthesis model 84 to describe how potential carbon assimilation rates are colimited by light, temperature and atmospheric CO 2 concentration and how this colimitation differs for C 3 and C 4 plants (details in ref. 24 ). We assume that each species uses either the C 3 or the C 4 photosynthetic pathway and use universal parameterizations of the C 3 and C 4 Farquhar models. Therefore, each grid cell has a universal maximum rate of monthly C 3 or C 4 carbon uptake that is determined by light, temperature and atmospheric CO 2 and this universal maximum rate can be further reduced by species-specific shoot-nitrogen and soil-water dependencies of carbon uptake (Supplementary Fig. 10 ).
The prescribed way in which environmental factors influence physiological processes (Supplementary Fig. 10 ), the simulation of monthly colimitation dynamics and the explicit consideration of CO 2 effects via a Farquhar-type carbon assimilation model are key conceptual differences to correlative species distribution models. These properties allow the model to extrapolate in physiologically plausible ways to novel data domains, thereby accommodating both novel data ranges and novel combinations of monthly values of climate variables. For example, a model comparison 33 , 85 between the TTR-SDM and the widely used correlative SDM Maxent 86 showed that, although the TTR-SDM has slightly lower ability to describe the species distribution data in the climate-data domain used to fit the models, it had a substantially better ability to describe independent species distribution data outside the climate-data domain used to fit the models. This model comparison provides confidence that the TTR-SDM can identify suitable climatic conditions.
To parameterize the model, one could measure the 18 model parameters shown in Supplementary Fig. 10 in the laboratory but this is not feasible when the goal is to parameterize the model for many species. The alternative is to infer the parameters from species distribution data and gridded climatologies. Conceptually, we achieve this as follows. First, we use the monthly climatic forcing data to simulate the biomass growth of a species at its presence and absence locations. Once the simulated biomass reaches equilibrium with the climate system forcing data, we use the natural log of this simulated biomass as the linear predictor in a logistic regression model that predicts the observed presences and absences of the species. The simulated biomass values are skewed and in such cases the complementary log-log link function is recommended. We therefore use the complementary log-log link function in this study. In practice, using logit or the complementary log-log often does not make a large difference 87 , even if there are theoretical reasons to prefer the complementary log-log. The inference process then uses an optimization algorithm to iteratively improve the likelihood of this regression model by optimizing the 18 parameters of the growth model (Supplementary Fig. 10 ), which are constrained by the prescribed trapezoidal and step functions defined by the model’s equations 32 . This optimization was performed using the Differential Evolution genetic algorithm 88 , a stochastic optimization method implemented in R in the DEoptim package 89 , 90 . We allowed the algorithm to iterate 1,000 times, which we found to be sufficient for generating stable parameterizations of the TTR-SDM.
We attempted to fit the TTR-SDM for species with at least seven presence data points. All presence points were used if less than 400 points were available. If more presence points were available, we took a random sample of presence points that conserved the proportions of the 20 environmental zones defined above (Supplementary Fig. 9 ) in the full set of presence points. For species with more presence records than environmental zones, we then sampled the same number of pseudo-absence points as presence points (the actual number varied slightly due to integer rounding). The probability of selecting a pseudo-absence point in an environmental zone was inversely proportional to the proportions of the zones in the presence point sample, which ensured that zones strongly represented in the presence point sample were less likely to be included in the pseudo-absence points sample. For species with less presence records than environmental zones, we used 20 pseudo-absence points to better constrain the parameter estimation. We found in a pilot study using the benchmarking dataset from ref. 33 that our sampling strategy for pseudo-absence points produced parameterizations of the TTR-SDM that had the highest ability to predict independent species distribution data. Other tested sampling strategies used the same stratified strategy as above but with two, five and ten times the number of pseudo-absence points as presence points, stratified sampling of pseudo-absence points without downweighting, random sampling of pseudo-absence points and the target-group approach 91 , each with two, five and ten times the number of pseudo-absence points as presence points. These alternative strategies were found to generate parameterizations of the TTR-SDM with marginally lower predictive accuracy.
Once the final parameterization is found, we use it to simulate the potential biomass of a species in the cells of a global grid using their monthly climatologies. The complementary log-log of the natural log of biomass is then used to calculate a suitability score (0–1). Last, to convert this suitability score into a binary prediction (0,1), we chose a threshold suitability score that maximizes the sum of true positives and true negatives in the presence and (pseudo-)absence data used to parameterize the model. The result is a map showing where the climate is suitable for that species.
The predictive accuracy of the model is then evaluated using the true skill statistic (TSS) calculated from a confusion matrix 92 that was computed using the abovementioned threshold. Models with low predictive accuracy (TSS ≤ 0.7) were removed. This resulted in fitted models for 135,153 species, consisting of 24,362 evergreen trees, 3,173 dry-deciduous trees, 1,270 cold-deciduous trees, 439 needleleaf trees, 24,853 evergreen shrubs, 2,074 dry-deciduous shrubs, 1,943 cold-deciduous shrubs, 21,903 therophytes, 8,297 geophytes, 23,538 forbs, 6,888 C 3 grasses, 2,814 C 4 grasses, 3,609 succulents and 9,990 climbers.
The parameters of the species models were estimated using the above-mentioned environmental forcing data interpolated to a 1 km grid. For subsequent data analyses these species models were projected onto a 25 km grid.
DEoptim is a robust and efficient global optimization algorithm capable of finding optima on irregularly shaped likelihood surfaces 88 . The stochastic nature of the algorithm means that running DEoptim several times for the same species can produce different parameter estimates. This parameter uncertainty produces uncertainty about the potential ranges of individual species, which we use to calculate the proportion of species of each growth form that could grow in the grid cells (the growth-form suitability values shown Fig. 1 ). The large number of species used in this analysis, however, ensures that the described species-level uncertainty is a negligible source of uncertainty in the growth-form suitability values. Supplementary Fig. 11 shows that taking five random samples of 50% of the species of a growth form and calculating the suitability scores of the grid cells from each sample yields almost identical suitability scores. In most growth forms, even smaller subsets would produce identical results (Supplementary Fig. 8 ). The saturating curves in Supplementary Fig. 8 also show that the numbers of species used in this study were sufficient to characterize the climatic preferences of each growth form. An additional sensitivity analysis showed that the estimation of the ambient suitability surfaces was robust to excluding all species with less than 20 occurrence records (Supplementary Fig. 12 ).
Biases in the species distribution data and the use of modelled coarse (1 km resolution) climate forcing data may bias the parameterization of the TTR-SDM. Model comparisons, however, show that the TTR-SDM is less biased than correlative SDMs in predicting locations where the climate is suitable for a species 33 , 85 . Moreover, so long as these biases are not systematic, our procedure of averaging over many species models creates robust estimates of the growth-form suitability surfaces (Supplementary Figs. 8 , 11 and 12 ).
Data analysis
For each 25 km grid cell, we calculated the proportion of species of each growth form that can grow in the cell according to the fitted TTR-SDM. This proportion is interpreted as the climatic suitability of a grid cell for a growth form (Fig. 1 ).
To find groups of cells with similar suitabilities for different growth forms we used finite Gaussian mixture modelling as implemented in the R package mclust 93 . We refer to the geographic projection of these groups as phytoclimatic zones (Fig. 2a ). We estimated the Bayesian information criterion (BIC) for different variations of the mclust algorithm, which revealed that the option ‘VEV’, allowing ellipsoidal, equally shaped clusters, consistently performed better than the alternatives. We used this option to model the clusters.
Using the number of (terrestrial) biomes delimited by global biome maps to guide the optimal number of clusters, one might delimit for instance 13 (ref. 94 ), 14 (ref. 95 ), 20 (refs. 96 , 97 ), 21 (ref. 98 ) or 30 (ref. 99 ) clusters. We used 18 clusters to trade-off information content versus interpretability. The BIC of the VEV clustering models improved with the number of clusters used up to 30 clusters but indicated that the BIC of 18 clusters was not substantially lower than the maximum BIC at 30 clusters.
Our indices of novelty, disappearance and local change of phytoclimates, as well as the projections of shifts in phytoclimatic zones by 2070, are based on the multivariate Euclidean distances (ED) between ambient and future climatic suitabilities:
where a k , i and b k , j are the ambient and future suitabilities for growth form k in grid cells i and j , respectively. For within grid cell phytoclimatic change, i equals j . Note that the growth-form suitability values all range from 0 to 1. We calculated the novelty of the future phytoclimate in grid cell x by setting i = x and calculating ED( i , j ) for j = 1 to N , where N is the total number of grid cells in the global 25 km grid. The minimum of these EDs is the distance of a future phytoclimate to its closest ambient analogue (ED min ). A high ED min thus indicates a high degree of novelty. Analogously, to determine phytoclimatic disappearance, we set j = x and calculate ED( i , j ) for i = 1 to N and calculate the minimum of these EDs. Here, high values of ED min indicate that an ambient phytoclimate has no close future analogue. Extended Data Figs. 3 and 4 show phytoclimatic change, novelty and loss for each GCM separately. Figure 3 shows the median values across the five projections per RCP.
To generate projections of shifts in phytoclimatic zones by 2070, we calculated the minimum ED of a future phytoclimate to its closest ambient analogue and extracted the ambient phytoclimatic zone of this closest ambient analogue. The future phytoclimate was then assigned to this phytoclimatic zone. Extended Data Fig. 5 shows shifts in phytoclimatic zones for each combination of RCP and GCM. Figure 2b in the main text shows the projection of zone shifts based on the median growth-form suitability values across the five projections for RCP 2.6 and Fig. 2c shows the same for RCP 8.5.
Reporting summary
Further information on research design is available in the Nature Portfolio Reporting Summary linked to this article.
Data availability
No new datasets were generated during the current study. The species distribution data were downloaded from the non-public version of the BIEN database v.4.1 ( http://bien.nceas.ucsb.edu/bien/ ), which was made available to us upon request to BIEN. Plant trait and life-history data used to infer the growth forms of the species came from BIEN, GIFT 80 ( https://gift.uni-goettingen.de/home ), the Illustrated Handbook of Succulent Plants 77 , 78 , 79 and the global database of C 4 photosynthesis in grasses 76 . Temperature and rainfall data were downloaded from CHELSA v.1.2 ( https://chelsa-climate.org/ ), solar radiation data were downloaded from the Global Aridity and PET Database v.1 ( https://csidotinfo.wordpress.com/data/global-aridity-and-pet-database/ ). Data on soil field capacity and wilting point came from the Global Gridded Surfaces of Selected Soil Characteristics (IGBP-DIS) dataset 62 ( https://doi.org/10.3334/ORNLDAAC/569 ). Atmospheric CO 2 concentrations were taken from ref. 63 . Free vector data from www.naturalearthdata.com were used to create the background country maps.
Code availability
R code to execute the TTR-SDM and a tutorial are available on Zenodo https://doi.org/10.5281/zenodo.10362617 .
IPCC. Climate Change 2014: Synthesis Report (eds Core Writing Team, Pachauri, R. K. & Meyer L. A.) (IPCC, 2014).
Nolan, C. et al. Past and future global transformation of terrestrial ecosystems under climate change. Science 361 , 920–923 (2018).
Article ADS CAS PubMed Google Scholar
Trisos, C. H., Merow, C. & Pigot, A. L. The projected timing of abrupt ecological disruption from climate change. Nature 580 , 496–501 (2020).
Fisichelli, N. A., Schuurman, G. W. & Hoffman, C. H. Is ‘resilience’ maladaptive? Towards an accurate lexicon for climate change adaptation. Environ. Manag. 57 , 753–758 (2016).
Article ADS Google Scholar
Lynch, A. J. et al. Managing for RADical ecosystem change: applying the Resist-Accept- Direct (RAD) framework. Front. Ecol. Environ. 19 , 461–469 (2021).
Article Google Scholar
Williams, J. W., Ordonez, A. & Svenning, J.-C. A unifying framework for studying and managing climate-driven rates of ecological change. Nat. Ecol. Evol. 5 , 17–26 (2021).
Article PubMed Google Scholar
Macgregor, N. A. & van Dijk, N. Adaptation in practice: how managers of nature conservation areas in eastern England are responding to climate change. Environ. Manag. 54 , 700–719 (2014).
Prober, S. M., Doerr, V. A. J., Broadhurst, L. M., Williams, K. J. & Dickson, F. Shifting the conservation paradigm: a synthesis of options for renovating nature under climate change. Ecol. Monogr. 89 , e01333 (2019).
Williams, J. W., Jackson, S. T. & Kutzbach, J. E. Projected distributions of novel and disappearing climates by 2100 AD. Proc. Natl Acad. Sci. USA 104 , 5738–5742 (2007).
Article ADS CAS PubMed PubMed Central Google Scholar
Garcia, R. A., Cabeza, M., Rahbek, C. & Araújo, M. B. Multiple dimensions of climate change and their implications for biodiversity. Science 304 , 1247579 (2014).
Ordonez, A., Williams, J. W. & Svenning, J.-C. Mapping climatic mechanisms likely to favour the emergence of novel communities. Nat. Clim. Change 6 , 1104–1109 (2016).
Mahony, C. R., Cannon, A. J., Wang, T. & Aitken, S. N. A closer look at novel climates: new methods and insights at continental to landscape scales. Glob. Change Biol. 23 , 3934–3955 (2017).
Williams, J. W. & Jackson, S. T. Novel climates, no-analog communities and ecological surprises. Front. Ecol. Environ. 5 , 475–482 (2007).
Hoffmann, S., Irl, S. D. H. & Beierkuhnlein, C. Predicted climate shifts within terrestrial protected areas worldwide. Nat. Commun. 10 , 4787 (2019).
Article ADS PubMed PubMed Central Google Scholar
Loarie, S. R. et al. The velocity of climate change. Nature 462 , 1052–1055 (2009).
Burrows, M. T. et al. The pace of shifting climate in marine and terrestrial ecosystems. Science 334 , 652–655 (2011).
Burrows, M. T. et al. Geographical limits to species-range shifts are suggested by climate velocity. Nature 507 , 492–495 (2014).
Sage, R. F. & Kubien, D. S. The temperature response of C 3 and C 4 photosynthesis. Plant Cell Environ. 30 , 1086–1106 (2007).
Article CAS PubMed Google Scholar
Mueller, K. E. et al. Impacts of warming and elevated CO 2 on a semi-arid grassland are non-additive, shift with precipitation and reverse over time. Ecol. Lett. 19 , 956–966 (2016).
Zhu, K., Chiariello, N. R., Tobeck, T., Fukami, T. & Field, C. B. Nonlinear, interacting responses to climate limit grassland production under global change. Proc. Natl Acad. Sci. USA 113 , 10589–10594 (2016).
Hansen, W. D. & Turner, M. G. Origins of abrupt change? Postfire subalpine conifer regeneration declines nonlinearly with warming and drying. Ecol. Monogr. 89 , e01340 (2019).
Peñuelas, J. et al. Shifting from a fertilization-dominated to a warming-dominated period. Nat. Ecol. Evol. 1 , 1438–1445 (2017).
Buermann, W. et al. Widespread seasonal compensation effects of spring warming on northern plant productivity. Nature 562 , 110–114 (2018).
Conradi, T. et al. An operational definition of the biome for global change research. New Phytol. 227 , 1294–1306 (2020).
Reu, B. et al. Future no-analogue vegetation produced by no-analogue combinations of temperature and insolation. Glob. Ecol. Biogeogr. 23 , 156–167 (2014).
Prentice, I. C. et al. in Terrestrial Ecosystems in a Changing World (eds Canadell, J. G. et al.) 175–192 (Springer, 2007).
Harrison, S. P. et al. Eco-evolutionary optimality as a means to improve vegetation and land-surface models. New Phytol. 231 , 2125–2141 (2021).
IPCC. Climate Change 2022: Impacts, Adaptation and Vulnerability (eds Pörtner, H.-O. et al.) (Cambridge University Press, 2022).
Dallmeyer, A., Claussen, M. & Brovkin, V. Harmonising plant functional type distributions for evaluating Earth system models. Climate 15 , 335–366 (2019).
Google Scholar
Higgins, S. I., Conradi, T., Kruger, L., O’Hara, B. & Slingsby, J. Limited climatic space for alternative ecosystem states in Africa. Science 380 , 1038–1042 (2023).
Thornley, J. H. Modelling shoot: root relations: the only way forward? Ann. Bot. 81 , 165–171 (1998).
Higgins, S. I. et al. A physiological analogy of the niche for projecting the potential distribution of plants. J. Biogeogr. 39 , 2132–2145 (2012).
Higgins, S. I., Larcombe, M. J., Beeton, N. J. & Conradi, T. Transferability of correlative and process-based species distribution models revisited: a response to Booth. Ecol. Evol. 11 , 13613–13617 (2021).
Article PubMed PubMed Central Google Scholar
Moncrieff, G. R., Bond, W. J. & Higgins, S. I. Revising the biome concept for understanding and predicting global change impacts. J. Biogeogr. 43 , 863–873 (2016).
Mucina, L. Biome: evolution of a crucial ecological and biogeographical concept. New Phytol. 222 , 97–114 (2019).
Köppen, W. in Handbuch der Klimatologie Vol. 1, Part C (eds Köppen, W. & Geiger, R.) 1–44 (Borntraeger, 1936).
Holdridge, L. R. Determination of world plant formations from simple climatic data. Science 105 , 367–368 (1947).
Suarez, F., Binkley, D., Kaye, M. W. & Stottlemyer, R. Expansion of forest stands into tundra in the Noatak National Preserve, northwest Alaska. Écoscience 6 , 465–470 (1999).
Tape, K., Sturm, M. & Racine, C. The evidence for shrub expansion in Northern Alaska and the Pan-Arctic. Glob. Change Biol. 12 , 686–702 (2006).
Harsch, M. A., Hulme, P. E., McGlone, M. S. & Duncan, R. P. Are treelines advancing? A global meta-analysis of treeline response to climate warming. Ecol. Lett. 12 , 1040–1049 (2009).
Terskaia, A., Dial, R. J. & Sullivan, P. F. Pathways of tundra encroachment by trees and tall shrubs in the western Brooks Range of Alaska. Ecography 43 , 769–778 (2020).
Lloyd, A. H., Rupp, T. S., Fastie, C. L. & Starfield, A. M. Patterns and dynamics of treeline advance on the Seward Peninsula, Alaska. J. Geophys. Res. 107 , 2–15 (2002).
Malhi, Y. et al. Exploring the likelihood and mechanism of a climate-change-induced dieback of the Amazon rainforest. Proc. Natl Acad. Sci. USA 106 , 20610–20615 (2009).
Charney, N. D. et al. A test of species distribution model transferability across environmental and geographic space for 108 Western North American tree species. Front. Ecol. Evol. 9 , 689295 (2021).
Schwalm, C. R., Glendon, S. & Duffy, P. B. RCP8.5 tracks cumulative CO 2 emissions. Proc. Natl Acad. Sci. USA 117 , 19656–19657 (2020).
Hansen, W. D., Fitzsimmons, R., Olnes, J. & Williams, A. P. An alternate vegetation type proves resilient and persists for decades following forest conversion in the North American boreal biome. J. Ecol. 109 , 85–98 (2021).
Conradi, T., Henriksen, M. V. & Svenning, J.-C. Global change, novel ecosystems and the ecological restoration of post-industrial areas: the case of a former brown coal mine in Søby, Denmark. Appl. Veg. Sci. 24 , e12605 (2021).
Bond, W. J. & Midgley, G. F. Carbon dioxide and the uneasy interactions of trees and savannah grasses. Philos. Trans. R. Soc. B 367 , 601–612 (2012).
Article CAS Google Scholar
Hoegh-Guldberg, O. et al. Assisted colonization and rapid climate change. Science 321 , 345–346 (2008).
Heller, N. E. & Zavaleta, E. S. Biodiversity management in the face of climate change: a review of 22 years of recommendations. Biol. Conserv. 142 , 14–32 (2009).
Hobbs, R. J. et al. Novel ecosystems: theoretical and management aspects of the new ecological world order. Glob. Ecol. Biogeogr. 15 , 1–7 (2006).
Wilsey, B. Restoration in the face of changing climate: importance of persistence, priority effects and species diversity. Restor. Ecol. 29 , e13132 (2021).
Zhu, K., Woodall, C. W. & Clark, J. S. Failure to migrate: lack of tree range expansion in response to climate change. Glob. Change Biol. 18 , 1042–1052 (2012).
Björkman, L. & Bradshaw, R. The immigration of Fagus sylvatica L. and Picea abies (L.) Karst. into a natural forest stand in southern Sweden during the last 2000 years. J. Biogeogr. 23 , 235–244 (1996).
Dullinger, S. et al. Extinction debt of high-mountain plants under twenty-first-century climate change. Nat. Clim. Change 2 , 619–622 (2012).
De Frenne, P. et al. Global buffering of temperatures under forest canopies. Nat. Ecol. Evol. 3 , 744–749 (2019).
Corlett, R. T. & Westcott, D. A. Will plant movements keep up with climate change? Trends Ecol. Evol. 28 , 482–488 (2013).
Svenning, J.-C. et al. Science for a wilder Anthropocene: synthesis and future directions for trophic rewilding research. Proc. Natl Acad. Sci. USA 113 , 898–906 (2016).
Perino, A. et al. Rewilding complex ecosystems. Science 364 , eaav5570 (2019).
Williams, J. W., Shuman, B. N. & Webb III, T. Dissimilarity analyses of late-Quaternary vegetation and climate in eastern North America. Ecology 82 , 3346–3362 (2001).
Karger, D. N. et al. Climatologies at high resolution for the earth’s land surface areas. Sci. Data 4 , 170122 (2017).
Global Soil Data Task Group. Global Gridded Surfaces of Selected Soil Characteristics (IGBP-DIS) https://doi.org/10.3334/ORNLDAAC/569 (ORNL DAAC, 2000).
Meinshausen, M. et al. The RCP greenhouse gas concentrations and their extensions from 1765 to 2300. Clim. Change 109 , 213–241 (2011).
Article ADS CAS Google Scholar
Gent, P. R. et al. The community climate system model version 4. J. Clim. 24 , 4973–4991 (2011).
Voldoire, A. et al. The CNRM-CM5.1 global climate model: description and basic evaluation. Clim. Dynam. 40 , 2091–2121 (2013).
Li, L. et al. The Flexible Global Ocean-Atmosphere-Land System Model, Grid-Point Version 2: FGOALS-g2. Adv. Atmos. Sci. 30 , 543–560 (2013).
Watanabe, S. et al. MIROC-ESM 2010: model description and basic results of CMIP5-20c3m experiments. Geosci. Model. Dev. 4 , 845–872 (2011).
Giorgetta, M. A. et al. Climate and carbon cycle changes from 1850 to 2100 in MPI-ESM simulations for the Coupled Model Intercomparison Project phase 5. J. Adv. Model. Earth Syst. 5 , 572–597 (2013).
Knutti, R., Masson, D. & Gettelman, A. Climate model genealogy: generation CMIP5 and how we got there. Geophys. Res. Lett. 40 , 1194–1199 (2013).
van Vuuren, D. P. et al. The representative concentration pathways: an overview. Clim. Change 109 , 5 (2011).
Jombart, T., Devillard, S. & Balloux, F. Discriminant analysis of principal components: a new method for the analysis of genetically structured populations. BMC Genet. 11 , 94 (2010).
Maitner, B. S. et al. The BIEN R package: a tool to access the Botanical Information and Ecology Network (BIEN) database. Methods Ecol. Evol. 9 , 373–379 (2018).
GBIF.org. GBIF Occurrence Download https://doi.org/10.15468/dl.yubndf (17 May 2018).
Fegraus, E. Tropical Ecology Assessment and Monitoring Network (TEAM network). Biodivers. Ecol. 4 , 287–287 (2012).
Zizka, A. et al. CoordinateCleaner: standardized cleaning of occurrence records from biological collection databases. Methods Ecol. Evol. 10 , 744–751 (2019).
Osborne, C. P. et al. A global database of C 4 photosynthesis in grasses. New Phytol. 204 , 441–446 (2014).
Eggli, U. & Hartmann, H. E. K. Illustrated Handbook of Succulent Plants Vols I–VI, 1st edn (Springer, 2001–2003).
Eggli, U. & Nyffeler, R. (eds) Monocotyledons . Illustrated Handbook of Succulent Plants 2nd edn (Springer, 2020).
Anderson, E. F. & Eggli, U. (eds) Das große Kakteen-Lexikon 2nd edn (Ulmer, 2011).
Weigelt, P., König, C. & Kreft, H. GIFT—a global inventory of floras and traits for macroecology and biogeography. J. Biogeogr. 47 , 16–43 (2020).
Zotz, G., Weigelt, P., Kessler, M., Kreft, H. & Taylor, A. EpiList 1.0: a global checklist of vascular epiphytes. Ecology 102 , e03326 (2021).
Kottek, M., Grieser, J., Beck, C., Rudolf, B. & Rubel, F. World map of the Köppen–Geiger climate classification updated. Meteorol. Z. 15 , 259–263 (2006).
Boyle, B. et al. The Taxonomic Name Resolution Service: an online tool for automated standardization of plant names. BMC Bioinforma. 14 , 16 (2013).
Von Caemmerer, S. Biochemical Models of Leaf Photosynthesis (CSIRO, 2000).
Higgins, S. I., Larcombe, M. J., Beeton, N. J., Conradi, T. & Nottebrock, H. Predictive ability of a process-based versus a correlative species distribution model. Ecol. Evol. 10 , 11043–11054 (2020).
Phillips, S. J., erson, R. P. & Schapire, R. E. Maximum entropy modeling of species geographic distributions. Ecol. Model. 190 , 231–259 (2006).
Phillips, S. J., erson, R. P., Dud´ık, M., Schapire, R. E. & Blair, M. E. Opening the black box: an open-source release of maxent. Ecography 40 , 887–893 (2017).
Price, K. V., Storn, R. M. & Lampinen, J. A. Differential Evolution—A Practical Approach to Global Optimization (Springer, 2006).
Ardia, D., Boudt, K., Carl, P., Mullen, K. M. & Peterson, B. G. Differential Evolution with DEoptim: an application to non-convex portfolio optimization. R J. 3 , 27–34 (2011).
Mullen, K., Ardia, D., Gil, D., Windover, D. & Cline, J. DEoptim: an R package for global optimization by Differential Evolution. J. Stat. Softw. 40 , 1–26 (2011).
Phillips, S. J. et al. Sample selection bias and presence-only distribution models: implications for background and pseudo-absence data. Ecol. Appl. 19 , 181–197 (2009).
Allouche, O., Tsoar, A. & Kadmon, R. Assessing the accuracy of species distribution models: prevalence, kappa and the true skill statistic (TSS). J. Appl. Ecol. 43 , 1223–1232 (2006).
Scrucca, L., Fop, M., Murphy, T. B. & Raftery, A. E. mclust 5: clustering, classification and density estimation using Gaussian finite mixture models. R J. 8 , 289–317 (2016).
Olson, D. et al. Terrestrial ecoregions of the world: a new map of life on Earth. BioScience 51 , 933–938 (2001).
Schultz, J. The Ecozones of the World (Springer, 2005).
Whittaker, R. H. Communities and Ecosystems (Macmillan Publishing, 1975).
Hengl, T. et al. Global mapping of potential natural vegetation: an assessment of machine learning algorithms for estimating land potential. PeerJ 6 , e5457 (2018).
Allen, J. R. M. et al. Global vegetation patterns of the past 140,000 years. J. Biogeogr. 47 , 2073–2090 (2020).
Pfadenhauer, J. & Klötzli, F. Global Vegetation (Springer, 2020).
Download references
Acknowledgements
We acknowledge the herbaria that contributed data to this work (see https://bien.nceas.ucsb.edu/bien/data-contributors/herbaria/ for full names): A, AAH, AAS, AAU, ABH, ACAD, ACOR, AD, ADW, AFS, AHUC, AIMS, AJOU, AK, AKPM, ALCB, ALT, ALTA, ALU, AMD, AMES, AMNH, AMO, ANA, ANGU, ANSM, ANSP, ANUC, ARAN, ARC, ARIZ, ARM, AS, ASDM, ASU, ATCC, AUG, AUT, B, BA, BAA, BAB, BACP, BAF, BAFC, BAI, BAJ, BAL, BARC, BAS, BBB, BBS, BC, BCF, BCMEX, BCN, BCRU, BEREA, BG, BH, BHCB, BHO, BILAS, BIO, BISH, BLA, BM, BO, BOCH, BOG, BOL, BOLV, BONN, BOUM, BPI, BR, BRA, BREM, BRI, BRIT, BRIU, BRLU, BRM, BSB, BSIP, BSN, BTN, BUL, BULU, BUT, C, CALI, CAMU, CAN, CANB, CANL, CAS, CAY, CBG, CBM, CBS, CEN, CEPEC, CESJ, CGE, CHAM, CHAP, CHAPA, CHI, CHL, CHR, CHRB, CIB, CICY, CIIDIR, CIMI, CINC, CIQR, CLEMS, CLF, CM, CMC, CMMEX, CNHM, CNPO, CO, COA, COAH, COCA, CODAGEM, COFC, COL, COLO, CONC, CORD, CP, CPAP, CPUN, CR, CRAI, CRP, CS, CSU, CTES, CTESN, CU, CUVC, CUZ, CVRD, CWU, DAO, DAOM, DAV, DBN, DES, DLF, DMNH, DMU, DNA, DR, DS, DUKE, DUSS, E, EA, EAC, EBH, EBUM, ECH, ECU, EIF, EIU, EKY, EM, EMMA, ENCB, ENS, ERA, ESA, ESS, F, FAA, FAU, FB, FBCS, FCME, FCO, FCQ, FEN, FH, FHO, FI, FLAS, FLOR, FM, FR, FRP, FTG, FUEL, FURB, G, GB, GDA, GDAC, GE, GENT, GEO, GES, GH, GI, GJO, GLM, GMNHJ, GOET, GUA, GZU, H, HA, HAC, HAJB, HAL, HAM, HAMAB, HAO, HAS, HAST, HASU, HAW, HB, HBG, HBR, HCIB, HEID, HGI, HIP, HKU, HNHM, HNT, HO, HPL, HRCB, HRP, HSS, HSU, HU, HUA, HUAA, HUAL, HUAZ, HUEFS, HUEM, HUFU, HUSA, HUT, HXBH, HYO, IA, IAA, IAC, IAL, IAN, IB, IBGE, IBUG, ICEL, ICN, IEB, IFO, ILL, ILLS, IMSSM, INB, INEGI, INIF, INM, INPA, IPA, IPRN, ISC, ISL, ISTC, ISU, ITCV, ITMH, IZAC, IZTA, JACA, JBAG, JE, JEPS, JOE, JUA, JYV, K, KANU, KIEL, KMN, KMNH, KOELN, KOR, KPM, KSC, KSTC, KSU, KTU, KU, KUO, KYO, L, LA, LAE, LAF, LAM, LCR, LD, LE, LEB, LEMA, LG, LI, LIL, LINN, LISE, LISI, LISU, LKHD, LL, LM, LOJA, LOMA, LP, LPAG, LPB, LPD, LPS, LSU, LTR, LY, LYJB, LZ, M, MA, MAF, MAIC, MAK, MAN, MARY, MASS, MB, MBK, MBM, MBML, MCM, MCN, MCNS, MEL, MEN, MERL, MEXU, MFA, MFU, MG, MGC, MICH, MIL, MIN, MISS, MJG, MMMN, MNHM, MNHN, MO, MOL, MOR, MOSS, MPU, MPUC, MRSN, MSB, MSC, MSE, MSTR, MSUN, MT, MTMG, MU, MUB, MUCV, MVFA, MVFQ, MVJB, MVM, MY, N, NA, NCSC, NCU, ND, NE, NEB, NHM, NHMC, NHT, NLH, NLU, NMB, NMC, NMCR, NMNL, NMR, NMSU, NMW, NO, NOU, NRCC, NSPM, NSW, NT, NUM, NWOSU, NY, O, OC, OCLA, ODU, OHN, OKL, OKLA, OMA, OS, OSA, OSC, OSH, OSN, OULU, OWU, OXF, P, PACA, PAR, PE, PEL, PENN, PERTH, PEUFR, PFC, PH, PI, PKDC, PLAT, PMA, PMNH, PNH, POLL, POM, PORT, PR, PRC, PRE, PTBG, PVNH, PY, QCA, QCNE, QFA, QM, QMEX, QRS, QUE, R, RAS, RB, RBR, REG, RENO, RFA, RIOC, RM, RNG, ROST, RPM, RSA, RYU, S, SALA, SAM, SAN, SANT, SAPS, SASK, SBT, SD, SEL, SEV, SF, SFSU, SGO, SI, SIM, SING, SIU, SJRP, SLPM, SMB, SMDB, SMF, SNM, SOM, SP, SPF, SPSF, SQF, SRFA, STL, STU, SUVA, SVG, SZU, TAES, TAI, TAIF, TAMU, TAN, TEF, TENN, TEPB, TEX, TFC, TFM, TI, TKPM, TNS, TO, TRA, TRH, TROM, TRT, TRTC, TRTE, TRTS, TS, TSM, TTRS, TU, TULS, TUR, U, UADY, UAM, UAMIZ, UARK, UAS, UAT, UB, UBA, UBC, UC, UCAM, UCBG, UCR, UEC, UESC, UFG, UFMA, UFMT, UFP, UFRJ, UFRN, UFS, UGDA, UH, UI, UJAT, ULM, ULS, UME, UMO, UNA, UNB, UNCC, UNEX, UNL, UNM, UNR, UNSL, UPCB, UPEI, UPNA, UPNG, UPS, US, USAS, USJ, USM, USNC, USON, USP, USZ, UT, UTC, UTEP, UTMC, UV, UVIC, UVSC, UWO, V, VA, VAL, VALD, VDB, VEN, VM, VMSL, VT, W, WAG, WAT, WELT, WFU, WII, WIN, WIS, WMNH, WOH, WRSL, WS, WTU, WU, XAL, Y, YA, YAM, YU, Z, ZMT, ZSS, ZT. S.I.H. acknowledges funding for the BMBF SPACES project EMSAfrica (grant no. 01LL1801A).
Open access funding provided by Universität Bayreuth.
Author information
Authors and affiliations.
Plant Ecology, University of Bayreuth, Bayreuth, Germany
Timo Conradi & Steven I. Higgins
Sukkulenten-Sammlung Zürich, Grün Stadt Zürich, Zürich, Switzerland
Biodiversity, Macroecology & Biogeography, University of Göttingen, Göttingen, Germany
Holger Kreft & Patrick Weigelt
Centre of Biodiversity and Sustainable Land Use (CBL), University of Göttingen, Göttingen, Germany
Campus-Institute Data Science, Göttingen, Germany
Institute of Landscape and Plant Ecology, Department of Plant Ecology, University of Hohenheim, Stuttgart, Germany
Andreas H. Schweiger
You can also search for this author in PubMed Google Scholar
Contributions
T.C. and S.I.H. designed the study, developed the methods and wrote the manuscript. T.C. compiled the data, performed the analyses and led the writing. U.E., H.K., A.H.S. and P.W. contributed data and helped refine the study design. A.H.S., P.W., S.I.H., U.E. and H.K. reviewed and edited the manuscript.
Corresponding author
Correspondence to Timo Conradi .
Ethics declarations
Competing interests.
The authors declare no competing interests.
Peer review
Peer review information.
Nature Ecology & Evolution thanks Tongli Wang and the other, anonymous, reviewer(s) for their contribution to the peer review of this work.
Additional information
Publisher’s note Springer Nature remains neutral with regard to jurisdictional claims in published maps and institutional affiliations.
Extended data
Extended data fig. 1 climatic suitability for major plant growth forms in 2070 under rcp 2.6..
Suitability is expressed as the proportion of plant species of a growth form for which the cells’ climate is suitable according to an ecophysiological plant growth model. The values are means across climatologies projected by five Global Circulation Models. Note that the range of the legend is slightly larger than that in Fig. 1 in the main text.
Extended Data Fig. 2 Climatic suitability for major plant growth forms in 2070 under RCP 8.5.
Extended data fig. 3 (a) change, (b) novelty and (c) disappearance of phytoclimates by 2070 under rcp 2.6 for five different global circulation models..
The phytoclimate of the cells is the suitability of the local climate for 14 plant growth forms that characterize the structure of terrestrial ecosystems. ( a ) Local change in phytoclimate. ( b ) Novelty of the projected phytoclimate in 2070, expressed as the Euclidean distance of a cell’s future phytoclimate to its closest ambient analogue. ( c ) Risk of disappearance of the existing phytoclimate, expressed as the Euclidean distance of a cell’s ambient phytoclimate to its closest future analogue.
Extended Data Fig. 4 (A) Change, (B) novelty and (C) disappearance of phytoclimates by 2070 under RCP 8.5 for five different Global Circulation Models.
Extended data fig. 5 projected distribution of phytoclimatic zones in 2070 under different climate change scenarios..
Shown is the projected distribution of phytoclimatic zones in 2070 under (A) RCP 2.6 and (B) RCP 8.5 when using climate data from five different Global Circulation Models.
Extended Data Fig. 6 Agreement on phytoclimatic zone in 2070 between five projections that used 2070 climate data from different Global Circulation Models.
A value of 5/5 means that all five projections made the same 2070 phytoclimatic zone prediction. A value of 2/5 means that only two of five projections made the same 2070 phytoclimatic zone prediction.
Extended Data Fig. 7 Change in climatic suitability of grid cells for plant growth forms between ambient and 2070 climatologies under RCP 2.6.
Change is expressed as change in proportion of species of a growth form that can grow in a cell according to our simulations. Values are medians across five projections that used 2070 climate data from different Global Circulation Models.
Extended Data Fig. 8 Change in climatic suitability of grid cells for plant growth forms between ambient and 2070 climatologies under RCP 8.5.
Supplementary information, supplementary information.
Supplementary Figs. 1–12 and Tables 1–3.
Reporting Summary
Rights and permissions.
Open Access This article is licensed under a Creative Commons Attribution 4.0 International License, which permits use, sharing, adaptation, distribution and reproduction in any medium or format, as long as you give appropriate credit to the original author(s) and the source, provide a link to the Creative Commons licence, and indicate if changes were made. The images or other third party material in this article are included in the article’s Creative Commons licence, unless indicated otherwise in a credit line to the material. If material is not included in the article’s Creative Commons licence and your intended use is not permitted by statutory regulation or exceeds the permitted use, you will need to obtain permission directly from the copyright holder. To view a copy of this licence, visit http://creativecommons.org/licenses/by/4.0/ .
Reprints and permissions
About this article
Cite this article.
Conradi, T., Eggli, U., Kreft, H. et al. Reassessment of the risks of climate change for terrestrial ecosystems. Nat Ecol Evol (2024). https://doi.org/10.1038/s41559-024-02333-8
Download citation
Received : 26 May 2023
Accepted : 17 January 2024
Published : 26 February 2024
DOI : https://doi.org/10.1038/s41559-024-02333-8
Share this article
Anyone you share the following link with will be able to read this content:
Sorry, a shareable link is not currently available for this article.
Provided by the Springer Nature SharedIt content-sharing initiative
Quick links
- Explore articles by subject
- Guide to authors
- Editorial policies
Sign up for the Nature Briefing newsletter — what matters in science, free to your inbox daily.

Climate Change Essay for Students and Children
500+ words climate change essay.
Climate change refers to the change in the environmental conditions of the earth. This happens due to many internal and external factors. The climatic change has become a global concern over the last few decades. Besides, these climatic changes affect life on the earth in various ways. These climatic changes are having various impacts on the ecosystem and ecology. Due to these changes, a number of species of plants and animals have gone extinct.

When Did it Start?
The climate started changing a long time ago due to human activities but we came to know about it in the last century. During the last century, we started noticing the climatic change and its effect on human life. We started researching on climate change and came to know that the earth temperature is rising due to a phenomenon called the greenhouse effect. The warming up of earth surface causes many ozone depletion, affect our agriculture , water supply, transportation, and several other problems.
Reason Of Climate Change
Although there are hundreds of reason for the climatic change we are only going to discuss the natural and manmade (human) reasons.
Get the huge list of more than 500 Essay Topics and Ideas
Natural Reasons
These include volcanic eruption , solar radiation, tectonic plate movement, orbital variations. Due to these activities, the geographical condition of an area become quite harmful for life to survive. Also, these activities raise the temperature of the earth to a great extent causing an imbalance in nature.
Human Reasons
Man due to his need and greed has done many activities that not only harm the environment but himself too. Many plant and animal species go extinct due to human activity. Human activities that harm the climate include deforestation, using fossil fuel , industrial waste , a different type of pollution and many more. All these things damage the climate and ecosystem very badly. And many species of animals and birds got extinct or on a verge of extinction due to hunting.
Effects Of Climatic Change
These climatic changes have a negative impact on the environment. The ocean level is rising, glaciers are melting, CO2 in the air is increasing, forest and wildlife are declining, and water life is also getting disturbed due to climatic changes. Apart from that, it is calculated that if this change keeps on going then many species of plants and animals will get extinct. And there will be a heavy loss to the environment.
What will be Future?
If we do not do anything and things continue to go on like right now then a day in future will come when humans will become extinct from the surface of the earth. But instead of neglecting these problems we start acting on then we can save the earth and our future.

Although humans mistake has caused great damage to the climate and ecosystem. But, it is not late to start again and try to undo what we have done until now to damage the environment. And if every human start contributing to the environment then we can be sure of our existence in the future.
{ “@context”: “https://schema.org”, “@type”: “FAQPage”, “mainEntity”: [ { “@type”: “Question”, “name”: “What is climate change and how it affects humans?”, “acceptedAnswer”: { “@type”: “Answer”, “text”: “Climate change is a phenomenon that happens because of human and natural reasons. And it is one of the most serious problems that not only affect the environment but also human beings. It affects human in several ways but in simple language, we can say that it causes many diseases and disasters that destroy life on earth.” } }, { “@type”: “Question”, “name”: “Can we stop these climatic changes?”, “acceptedAnswer”: { “@type”: “Answer”, “text”: “Yes, we can stop these climatic changes but for that, every one of us has to come forward and has to adapt ways that can reduce and control our bad habits that affect the environment. We have to the initiative and make everyone aware of the climatic changes.” } } ] }
Customize your course in 30 seconds
Which class are you in.

- Travelling Essay
- Picnic Essay
- Our Country Essay
- My Parents Essay
- Essay on Favourite Personality
- Essay on Memorable Day of My Life
- Essay on Knowledge is Power
- Essay on Gurpurab
- Essay on My Favourite Season
- Essay on Types of Sports
Leave a Reply Cancel reply
Your email address will not be published. Required fields are marked *
Download the App

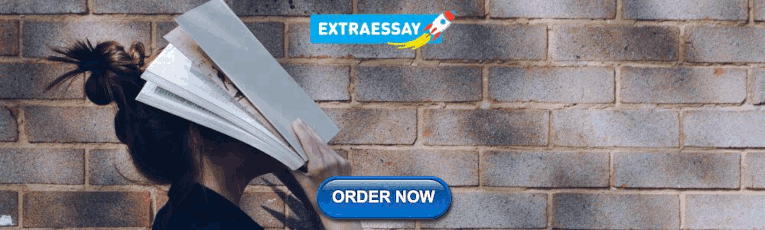
IMAGES
VIDEO
COMMENTS
Climate change causes shifts in ecosystems. Rising temperatures cause shifting ecosystems, either expanding or decreasing the geographical range of specific types of habitats, or changing the timing of seasons. For example, a study of European butterflies found that populations had shifted north by 114 km between 1990-2008 due to increasing ...
In our introduction we outline the themes, introduce the papers in the thematic issue, and conclude with a synthesis of the main findings of the Forum. In doing so, we emphasize the research needed to better understand threats, opportunities and solutions regarding climate change and ecosystems. ... They highlight key evidence for nature's role ...
This article is part of the theme issue 'Climate change and ecosystems: threats, opportunities and solutions'. Keywords: adaptation, mitigation, biosphere, nature-based solutions, resilience, climate change impacts. (ii) identify and address the key yet tractable knowledge gaps in ecosystem science.
1 Introduction The worldâ s climate is changing, and it will continue to change throughout the 21st century and beyond. Rising temperatures, new precipitation patterns, and other changes are already affecting many aspects of human society and the natural world. Climate change is transforming ecosystems at extraordinary rates and scales.
Climate fundamentally controls the distribution of ecosystems, species ranges, and process rates on Earth. As a component of the US National Climate Assessment, to be released in 2014, a group of over 60 ecological experts from academic, governmental, and nongovernmental organizations assessed the state of knowledge about how climate change has affected and will affect species, biodiversity ...
The project focused on "climate change impacts to Tsleil-Waututh, also known as Burrard Inlet IR#3, which is the current village site for the Nation. In the future, TWN hopes to expand the assessment to consider the vulnerability of other areas within its traditional territory" (Tsleil-Waututh Nation, 2019).
Climate change has altered and will continue to alter the provision, timing, and location of ecosystem functions across landscapes. Ecosystem functions, such as nutrient recycling in soil and the timing and volume of water flows, become ecosystem services when humans translate them into valuable processes, materials, and commodities. For example, crop production, which takes advantage of the ...
Frontiers in Ecology and the Environment is an environmental science journal ... nongovernmental organizations, and the private sector assessed the current and projected impacts of climate change on ecosystems, biodiversity, and ecosystem services. Here, we introduce and provide context for the papers included in this Special Issue, drawing ...
Climate-change ecology is the study of the effects of anthropogenic climate change on any aspect of ecology. It includes the effects of altered temperature and precipitation on the distribution ...
Earth faces a climate emergency which renders conservation goals largely obsolete. Current conservation actions are inadequate because they (i) underplay biodiversity's role in maintaining human civilisation, which contributes to its marginalisation, and (ii) rely on false assumptions of how to catalyse transformative change. We suggest a paradigm shift from biodiversity conservation to ...
Understanding the interactions between climate and biodiversity is a complex challenge to science. With contributions from 60 key researchers, this book exam-ines the ongoing impact of climate change on the ecology and diversity of life on earth. It discusses the latest research within the fields of ecology and systematics, highlighting the ...
Climate change is impacting nutritional security from seafood. Global warming and overfishing are impacting fish species distribution, total catch and aquaculture viability, limiting our ability ...
However, ecological transformation can occur gradually, as incremental changes in dominant species populations accumulate and species ranges shift under changing climate. Developing a science of transformational ecology is timely in view of ongoing and imminent changes. In the face of transformation, natural-resource managers have three options ...
Climate change contributes to the loss of biodiversity 1,2,3,4,5,6, highlighting a pressing need to identify how populations respond to climate change and to use this information to conserve species.
Climate change is also changing the way species and populations interact with the environment and one another. These impacts can be felt throughout an entire ecosystem. For example, climate change is increasing the spread of invasive species in some areas. 11 An invasive species is one that is not native to an area.
Analysis of Warren et al. ( 2018) on a global scale on the effects of climate change on the distribution of insects, vertebrates and plants indicated that even with 2 °C temperature increase, approximately 18% of insects, 16% of plants and 8% of vertebrates species are projected to loose > 50% geographic range; this falls to 6% for insects, 8% ...
The ecological impacts of climate change are broad and diverse, altering species' range limits, plant phenology and growth, carbon and nutrient cycling, as well as biodiversity and extinction risk. Over the past year, PLOS has published a wide range of research on the ecological impacts of climate change. The breadth of topics includes everything from impacts of climate change on the immune ...
The Paris Agreement expands on the Convention by bringing all nations together for the first time in a single cause to undertake ambitious measures to prevent climate change and adapt to its impacts, with increased funding to assist developing countries in doing so. As so, it marks a turning point in the global climate fight.
The impacts identified in the reviewed papers represent only some of the climate change effects documented in the literature. This is likely related to the limited science production in the target region and our requirement to identify studies that inform about both impacts and management and policy responses.
Climate-change ecology. Forecasting the risks of climate change for species and ecosystems is necessary for developing targeted conservation strategies. Previous risk assessments mapped the ...
Climate change refers to the change in the environmental conditions of the earth. This happens due to many internal and external factors. The climatic change has become a global concern over the last few decades. Besides, these climatic changes affect life on the earth in various ways. These climatic changes are having various impacts on the ...
This essay is about ecology and the climate, and the relationship between the two, within the context of sustainability, which is the capacity of the planet to keep up itself despite the natural occurring changes within the Indian Society and the rest of the world. (Smith, T.M., Smith, R.L. (2012)). ... Climate change impacts are not new issues ...
Bahçeşehir College is committed to increasing students' awareness of the changing world we live in. This climate change essay competition saw many students submitting well thought out pieces of writing. These essays were marked on their format, creativity, organisation, clarity, unity/development of thought, and grammar/mechanics.
Climate change and pollution were among the major drivers of biodiversity loss for many other understudied taxa, including aquatic invertebrates and microbes (Figure 3c). While demonstrating that land- and sea-use change is essential to address, our results also indicate that comprehensively conserving biodiversity will require tackling many ...