
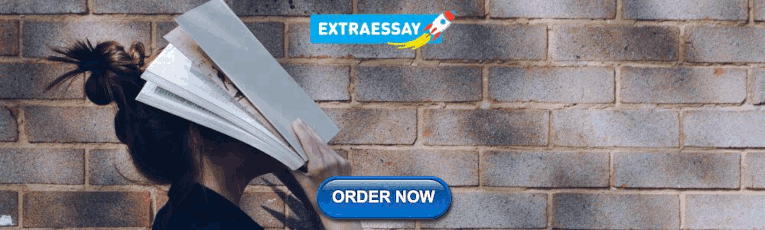
Future Directions for Electric Propulsion Research
Preserved fulltext.

Perspectives on the success of electric propulsion
- Open access
- Published: 09 September 2022
- Volume 1 , article number 9 , ( 2022 )
Cite this article
You have full access to this open access article
- John R. Brophy 1
3947 Accesses
5 Citations
3 Altmetric
Explore all metrics
Electric propulsion is now in both widespread use and active flight-implementation across a broad spectrum of commercial and government applications ranging from cubesats, LEO constellations, GEO comsats, deep space science missions, and even the human-tended Lunar Gateway. It has been my good fortune to witness, and even participate in, to some small extent, the transition of electric propulsion technology from laboratory development to its current wide-ranging acceptance. This paper summarizes my recollection on how this happened from the perspective of working on electric propulsion for 44 years, most of it at NASA’s Jet Propulsion Laboratory. Space limitations dictate that this summary cannot include all (or maybe even most) of the important details and developments of a story that spans more than 40 years. The objective was to identify the key factors that drove electric propulsion’s successes.
Similar content being viewed by others
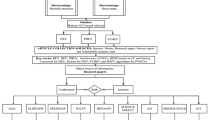
A comprehensive review on hybrid electric vehicles: architectures and components
Krishna Veer Singh, Hari Om Bansal & Dheerendra Singh
Tesla Energy
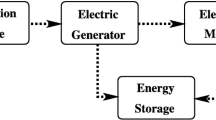
Aircraft Hybrid-Electric Propulsion: Development Trends, Challenges and Opportunities
Manuel A. Rendón, Carlos D. Sánchez R., … Alexandre H. Anzai
Avoid common mistakes on your manuscript.
Introduction
You know you’ve been around for a long time if you get asked to write a perspective on something. I started working in electric propulsion (EP) in 1978 and have been doing so ever since. This article gives my perspective on how electric propulsion went from a small, but enthusiastic band of technologists around the world to literally darkening the skies with EP satellites. The primary messages from the perspective that follow are that for new technologies to make the transition from development to wide spread application, the benefits have to be compelling and rock solid, perseverance is essential, and the exact timing of the adoption of any new technology can both be a matter of luck and dependent on developments outside of your control.
Fundamentals
Mission designers have long bemoaned the “tyranny of the rocked equation” (see [ 1 ] for example). For electric propulsion technologists, however, the rocket equation is your friend. Space is big and getting around in time scales of interest requires large velocity changes. This fundamental fact of nature inescapably drives the need for high exhaust velocities. But high exhaust velocities by themselves are not sufficient for a practical propulsion system.
The first EP conference I attended was the International Electric Propulsion Conference (IEPC) at Princeton, NJ in 1979. This was already the 14th IEPC, which at the time were held approximately every 18 months, indicating that dedicated EP conferences had been ongoing for over 20 years. My major impression from this conference was “holy cow” there are a lot of different electric propulsion concepts including: direct-current and radio frequency ion thrusters of various sizes and various propellants; solid-, liquid-, and gaseous-propellant magnetoplasmadynamic (MPD) thrusters; pulsed inductive thrusters (PIT); pulsed plasma thrusters (PPT); rail guns; and mass drivers. The market place would ultimately select a few of these concepts for wide-spread application. The technologies selected for application would change over time in response to new EP developments and changing market place needs. The technologies not selected would remain in the category of “advanced technologies” where “advanced” here means low on NASA’s Technology Readiness Level (TRL) scale [ 2 ]. NASA would continue to support research on many of these concepts, but as Earl Vanlandingham, who was an outstanding program manager for power and propulsion technologies at NASA Headquarters, once told me, “Advanced propulsion technology is like parsley, you want a little on your plate, but you don’t want too much.”
Power, lots of power
When I was doing my graduate research at Colorado State University in the late 1970’s, my advisor Professor Paul Wilbur would occasionally reflect on the fact that NASA had been supporting the development of EP technologies for 20 years but there still weren’t any real applications or even concrete plans to implement the technology. He used to tell me that, “we just need a mission.” But to “just get a mission” required power, lots of power, and for that the needs of electric propulsion would have to wait for available, affordable power in space catch up.
In the 1950’s, estimations of the power required for an EP mission took the following equation for the power per spacecraft mass, P / M S/C ,
and plugged in an acceleration of a = 0.01 g, an exhaust velocity of v ex = 100 km/s, and an efficiency of η = 0.7 to get a required power per unit mass of 7 kW/kg. Half a century later, NASA’s Dawn spacecraft that used an ion propulsion system to rendezvous with the giant main belt asteroid (4) Vesta and the dwarf planet (1) Ceres, had an initial wet mass of 1218 kg. According to the above calculation the Dawn spacecraft would need an estimated power level of order 8.5 MW. Furthermore, in 1958, the first solar-powered spacecraft, Vanguard ( https://en.wikipedia.org/wiki/Vanguard_1 ), was launched with an array of 6 solar cells that produced ~ 1 W of photovoltaic power. It is no wonder then that early expectations assumed practical EP systems would be nuclear powered. Footnote 1 It did not turn out that way.
The actual maximum input power to Dawn’s EP system was 2.5 kW provided by a photovoltaic solar array that produced slightly more than 10 kW of electrical power beginning of life at 1 AU [ 3 ]. This was possible because in the 1960’s, trajectory specialists determined that practical deep space missions could be accomplished with much lower vehicle accelerations than originally expected. Practical, missions could be performed with accelerations of order 10 − 5 g instead of the 10 − 2 g assumed above. In addition, exhaust velocities of roughly 30 km/s were found to be better suited to near-term missions of interest instead of 100 km/s. These two changes result in a factor of ~ 3300 reduction in the power required per unit mass, reducing 8.5 MW for a Dawn-sized vehicle to ~ 2.6 kW.
In parallel with improved low-thrust mission designs, the development of photovoltaic solar arrays for spacecraft advanced rapidly with the maximum solar array power doubling roughly every 4 years from the 1960’s through the early 2000’s. This resulted in the situation where by the 1990’s available, affordable power in space had caught up to the needs of electric propulsion resulting in the adoption of EP in various forms on commercial geosynchronous communication satellites. For example, beginning in 1997 the Hughes 601HP satellite used solar array power margin to operate gridded ion thrusters for station keeping. The use of EP on geosynchronous comsats significantly advanced the thruster technology in the US, Europe and Japan, and also increased customer acceptance of EP as a valuable cost-savings tool rather than a high-risk gadget.
An alternate illustration of the advance in solar array power and affordability is the Dawn spacecraft that was selected for flight implementation in 2001. Even though Dawn was a relatively small, cost-capped deep space robotic science mission, it could afford a higher-power solar array than that which flew on the human-tended Skylab space station from the 1970’s.
Propellants: many are called, few will serve
The first propellants used in early ion thrusters were mercury and cesium. These propellants were attractive because they had a large atomic mass, were easy to ionize, and were relatively easy to vaporize. They could also be stored as a liquid at low pressure and room temperature resulting in small tankage fractions. In spite of these desirable features and despite decades of research, no actual operational electric propulsion systems ever used them as propellants. The best these propellants could manage was a few flight experiments.
In the 1960s and 70’s, technologists demonstrated that you could operate ion thrusters and Hall thrusters on pretty much anything you could turn into a vapor. But this did not automatically indicate that any of these materials would be useful as a propellant in an operational propulsion system. In fact, a good way to tell that a new electric propulsion technology is both immature and in “sell mode” is the claim that it can be used with a wide variety of propellants.
To actually be an attractive propellant for electric thrusters requires consideration of factors other than those mentioned above, i.e., large atomic mass, easy ionization, easy vaporization, and low tankage fraction, which may be considered as an initial filter or acceptance criteria. Electric propulsion systems, of course, are part of a spacecraft, and have to operate for typically thousands to tens of thousands of hours. Therefore, the potential contamination of the spacecraft by the propellant is a critical consideration. Not all of the propellant injected into an electric thruster gets ionized and exhausted at high velocity. Some fraction, of order 10% or so, “leaks” out of the thruster unionized. This low velocity exhaust can get partially ionized and envelop the host spacecraft. No surface of the spacecraft can be guaranteed to be free from propellant deposition. Furthermore, the long operating times for electric thrusters requires extensive life testing in ground-based vacuum test facilities. Contamination of these increasingly expensive facilities by hazardous propellants is also a major consideration.
In the 1980s, NASA’s Lewis Research Center (now the Glenn Research Center) switched from mercury to xenon as the propellant of choice for ion thruster technology development. The Soviet Union, of course, had been using xenon in their Hall thrusters (or Stationary Plasma Thrusters) since the 1970s and NASA had been investigating the operation of ion thrusters on noble-gas propellants for many years before the switch to mercury.
The use of xenon has several key benefits as a propellant for spacecraft. Aside from meeting the acceptance criteria listed above, xenon is inert for all intents and purposes. It poses no contamination hazard to the host spacecraft or to ground test facilities. The flow rate of xenon can be precisely controlled using feed system components (valves, pressure regulators, etc.) that were developed and flight qualified for other applications. This enabled the straight forward and affordable development of propellant feed systems for ion thrusters.
Of course, there are always exceptions. In some cases, condensable propellants may be indispensably fundamental to the operation of a particular type of thruster. If the benefits of the thruster technology are sufficiently compelling, then the issues of spacecraft contamination and ground test facility contamination would have to be dealt with.
Thruster life
The power-limited, low-thrust nature of electric propulsion typically requires thrusters capable of operating for several thousand to tens of thousands of hours. Efforts to demonstrate adequate thruster life date back to the very beginnings of electric propulsion technology development. While there are many, many examples of life testing efforts (see for example Ref [ 4 ]). An illustrative example is the development of NASA’s 30-cm-diameter, J-Series mercury ion thruster. This thruster development began in 1970 and ended with the demise of NASA’s Solar Electric Propulsion Stage (SEPS) program in 1981. The thruster development went through ten design iterations beginning with the 100-series thruster, then 200-, 300-, and so on. When the program got to the tenth iteration, they did not want to call it the 10-hundred series, so instead called it the J-series, with J being the tenth letter in the alphabet. While this development program identified and solved many technical issues, its key challenge was demonstrating adequate thruster life. The goal to demonstrate thruster life drove many of the design changes generally resulting in the next thruster generation and corresponding higher thruster-series number. Typically, multiple thrusters of each generation were fabricated and tested. These thrusters were serialized, i.e., 101, 102 or 201, 202, etc. So important was the life demonstration goal that as soon as the latest series thruster was fabricated and acceptance tested, it was placed into a long-duration life test, i.e., thruster #101, or 201, 301, etc. This led Bob Bechtel—who worked at NASA LeRC (now GRC) and finished his career at MSFC—to state the unwritten rule of EP thruster development, “you always life test serial #001.”
It wasn’t until the NSTAR (NASA’s SEP Technology Applications Readiness) project in the 1990’s that an ion thruster intended for primary propulsion demonstrated adequate life to be useful for missions of interest. Footnote 2 Demonstration of the NSTAR thruster life began with a scheduled 2000-hr test at NASA LeRC. This test did not go well, revealing several accelerated erosion mechanisms, causing the test to be terminated after just 1000 hrs [ 5 ]. Straight forward fixes to the thruster were made and a second 1000-hr wear test was performed, this time at JPL [ 6 ]. This wear test demonstrated that the changes made after the first 1000-hr wear test successfully addressed the accelerated erosion issues setting the stage for an 8000-hr life demonstration test. The duration of 8000 hours was selected as the minimum time (and propellant throughput) that would enable useful deep space science missions with the NSTAR technology.
A vacuum test facility at JPL was created with the specific objective of being used for long-duration gridded ion thruster testing. At the time, demonstrating thruster life was one of the major outstanding technical issues impeding adoption of the ion propulsion technology and there were no facilities at NASA dedicated to addressing this issue. There were lots of test facilities at NASA GRC and JPL that were used for electric thruster testing, but there were also lots of demands on these facilities and none of them could be tied up for the multiple years necessary to qualify a single thruster for life. Somewhat unexpectedly, as soon as the facility at JPL was operational, it was used to demonstrate the life not of a gridded ion thruster, but of the SPT-100 thruster [ 7 ]. At the completion of the SPT-100 life test, the facility was dedicated to wear testing of the NSTAR gridded ion thruster for the next 8 years.
During the 1000-hr NSTAR thruster wear test at JPL, a flow rate calibration error led to discovery that efficient discharge chamber operation could be achieved at lower discharge voltages than previously believed [ 6 ]. Specifically, discharge voltages of around 24 V instead of the more typical 28 V were found to yield performance nearly as good as at 28 V. Operation at 24 V resulted in significant lower internal erosion rates making the trade off between thruster performance and thruster life lean heavily in favor of thruster life. This operating mode was carried over to the 8000-hr NSTAR life demonstration test (LDT) [ 8 ]. Not everyone involved in the NSTAR project, however, was convinced that the right choice was made in this tradeoff between performance and life. Consequently, for 100 hours nearly right in the middle of the LDT, the operating point was changed to the 28-V condition. This is indicated in Fig. 1 as the “100 Hour PAT Test.” These data indicate that the 28-V condition results in an increase in the double ion fraction in the ion beam from about 13% to 22%. Since most of the internal erosion in a gridded ion thruster is known to be caused by doubly-charged ions, this increase along with the higher discharge voltage would have had a disastrous effect on the thruster life. After 100 hours, the operating point was returned to the low discharge voltage condition to finish out the LDT. It was subsequently used in the 5-year-long extended life test (ELT) and in both the DS1 and Dawn missions.
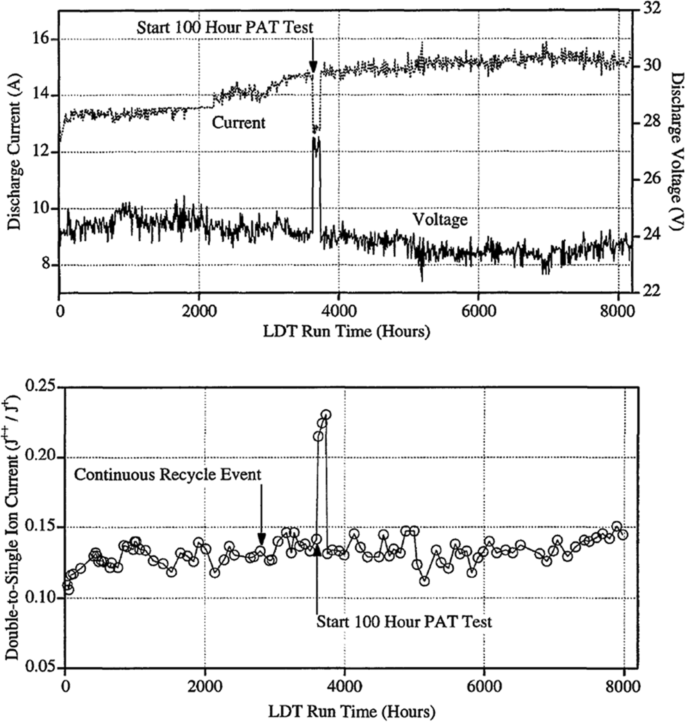
Discharge current and voltage (top) and double ion fraction (bottom) as a function of time during the 8000-hr Life Demonstration Test (LDT). The “100 Hour PAT Test” indicates the period of time in which the thruster flow rates were changed to what was perceived by some to be a more efficient operating point. The problem, as these figures clearly indicate, is that this operating point results in a significant increase in discharge voltage and double ion content, to the extent that the result would be a much shorter thruster life capability
The key to EP thruster life qualification is to identify all of the important wear-out failure modes and to understand their behavior. Unexpected results must be thoroughly investigated and understood. As Jay Polk from JPL would say, “if it seems weird, it is,” meaning it is not acceptable to discount weird results as “anomalous” and then ignore them.
The extended life test (ELT) was the final test in the NSTAR life qualification effort [ 9 ]. This test used the flight spare thruster from DS1 and served as the thruster life qualification test for the Dawn mission. The test spanned a duration of 5 years (from 1999 through 2003). At this same time, NASA began the NEXT (NASA’s Evolutionary Xenon Thruster) program to develop a bigger, better, higher-power, higher specific impulse, ion thruster. This set up a resource battle that lasted throughout the duration of the ELT. Every year of the ELT there was a serious threat to cancel the life test and redirect the resources to NEXT. These threats finally succeeded in the 5th year even though the test was still producing useful information about long-duration operation at low power. Cancellation of the test increased the risk for the already-selected Dawn mission whose mission design required extensive operation at lower. The ELT was cancelled in favor of supporting the NEXT technology, a technology which, as it turned out, would not fly for another 20 years, and even then only as an extremely limited technology demonstration ( https://en.wikipedia.org/wiki/Double_Asteroid_Redirection_Test ).
The ELT demonstrated over 30,000 hours of thruster operation, covered the full range of power throttling and provided sufficient data to enable the life qualification of the NSTAR thruster for the Dawn mission. It provided high confidence that the NSTAR thruster could perform the Dawn mission with a low risk of wear-out failure.
False starts
From the 1970s through the early 1990s, there were many attempts by NASA to establish flight projects that took advantage of the significant benefits enabled by electric propulsion. Some notable examples are the Comet Haley Rendezvous mission [ 10 , 11 ], the SEPS (Solar Electric Propulsion System) program [ 12 ], and the Space Exploration Initiative ( https://en.wikipedia.org/wiki/Space_Exploration_Initiative ).
The Comet Haley Rendezvous mission study included a shoot-out between high-power solar electric propulsion and large solar sails. The SEP system proposed the use of an array of ten mercury ion thrusters with an Isp of 4750 s and a 60-kW concentrator solar array [ 10 ]. The solar sail system proposed the use of multikilometer-long blades in a “heliogyro sail configuration [ 11 ]. The SEP system won the competition, but it was a hollow victory, since the mission was not funded. In hindsight, both sides of the competition were significantly exaggerating their technology readiness and flight implementation of either system would have been extremely high risk.
Around 1979, NASA initiated the SEPS program [ 12 ]. The objective of this program was to develop a high-energy upper stage that could be used for multiple deep space science missions. The candidate missions included a comet rendezvous mission, a Saturn orbiter with probe mission, and a multiple main-belt asteroid rendezvous mission. The stage was to be based on the use of the 2.5-kW, J-Series, mercury ion thruster and a 15-kW solar array. The project funded two Phase-B contractor studies. The results of these studies were presented at the Marshall Space Flight Center (MSFC) at the end of 1980. The project was cancelled as part of federal budget considerations early in 1981 before the winning contractor was selected. This effectively ended all work at NASA on mercury-fueled ion thrusters.
In the early 1990’s, NASA briefly flirted with what became known as the Space Exploration Initiative (SEI) ( https://en.wikipedia.org/wiki/Space_Exploration_Initiative )—a plan to send humans back to the Moon, this time to stay and then go on to do human exploration of Mars. This stimulated a lot of studies into high-power nuclear electric propulsion (NEP) systems and technologies since NEP was one of the options, albeit not the leading option, for human missions to Mars. As EP technologists, we embraced this initiative wholeheartedly. The initiative, however, was short-lived and effectively cancelled in 1993 due mostly to the estimated price tag. This, of course, wasn’t the first round of NEP studies for human missions to Mars and it wouldn’t be the last.
Stationary plasma thrusters
At the start of the 1990s, the Soviet Union began marketing selected space technologies to the west. One of these technologies was the Stationary Plasma Thruster (SPT). The claimed performance for the SPT-100 was an 50% efficiency at an Isp of 1500 s. This was simultaneously attractive and the subject of some skepticism. In fact, the skepticism was sufficiently high that Len Caveny of the Ballistic Missile Defense Organization (BMDO) funded a small team that I led to travel to the Soviet Union in 1991 and experimentally verify the performance of the SPT-100. This team included: John Barnett, a former supervisor of JPL’s electric propulsion group; John Sankovic, an electric propulsion technologist at the Glenn Research Center; and David Barnhart, a propulsion technologist for the U.S. Air Force.
Doubts about the performance claims largely centered on the following (flawed) logic. The SPT-100 efficiency was higher that that of a gridded ion thruster. In gridded ion thrusters, the acceleration process is in practice greater than 99% efficient (depending, of course, on how you define this efficiency). In this case, it’s defined as the ratio of the ion beam current to the beam current plus the ion current to the accelerator grid). With such a high efficiency, the argument went, how could a Hall thruster be more efficient?
Performance verification in the Soviet Union
We brought three large containers of instruments from JPL to the Soviet Union. We used this equipment to verify calibration of the thrust stand, calibration of the propellant flow meters, the vacuum chamber pressure, and the makeup of residual gasses in the vacuum chamber. The engineers and technicians at both the Scientific-Research Institute of Thermal Processes in Moscow (NIITP, now the Keldysh Research Center) and Design Bureau Fakel in Kaliningrad, Russia were extremely helpful and efficient in adapting our equipment to theirs to enable these measurements. The result of the evaluation performed using a combination of U.S. and Soviet instrumentation was complete confirmation of the performance claims within the limitations of the instrumentation and facilities. The explanation for why the efficiency was higher than that of a gridded ion thruster is that the SPT-100 produces and extracts into the ion beam ions more efficiently than gridded ion thrusters. That is, the energy cost to produce ions (typically in eV/ion) that make it into the exhaust is lower in the SPT-100, and this becomes an increasingly important term in the efficiency calculation as the specific impulse is reduced to 1500 s where the SPT-100 is designed to operate.
SPT-100 life testing at JPL
As with gridded ion thrusters, the major remaining technical issue with Hall thruster technology in the early 1990s was thruster life. The higher plasma densities enabled by the non-space-charge-limited ion acceleration in Hall thrusters were expected to result in increased erosion rates and shorter thruster lifetimes. For this reason, BMDO funded JPL to perform a life test of the SPT-100 thruster. This test successfully demonstrated 5730 hours of operation and also identified a previously unknown wear-out failure mechanism—erosion of the non-operating cathode [ 7 ]. The combination of good performance and acceptable thruster life for the SPT-100 led to the widespread adoption of Hall thruster technology around the world.
Deep Space 1
By 1990, NASA had been supporting the development of 30-cm diameter gridded ion thruster technology for 20 years. This began with the mercury-fueled, divergent-field, 100-series thruster in 1970 [ 13 ] evolving eventually into a 5-kW, xenon-fueled, ring-cusp thruster by 1990 [ 14 ]. It was clear by 1990 that a flight test was needed to push the technology to completion. In a meeting at NASA HQ, I asserted that since they had been funding the development of 30-cm gridded ion thruster technology for 20 years, they should either finish it by developing flight hardware for a flight test on a U.S. Air Force satellite called ELITE (Electric Insertion Transfer Experiment) [ 15 ] or terminate the development. Fortunately, NASA’s response was to start the NSTAR project with the objective of developing the flight hardware.
The ELITE satellite was intended to flight-test a high-power, ammonia-fueled arc jet. As a result, it had the all-important power resources available that could be used to operate the NASA-provided ion thruster. The teaming arrangement between the Air Force and NASA was expected to improve the political prospects for ELITE, making it more likely to be funded to completion. This was not to be the case. In efforts to keep ELITE alive it was renamed SSTAR (Space Surveillance Track and Autonomous Reposition) and the corresponding NASA ion thruster development program was named NSTAR in a show of support, but ELITE/SSTAR was eventually cancelled. This left NASA with a program to develop the NSTAR flight hardware with nothing to fly it on.
At the beginning of the NSTAR program, the maximum thruster power level had to be selected. At the time, NASA GRC had been developing a 30-cm gridded ion thruster to operate at 5 kW. However, to make it easier to fly, engineers at JPL made a convincing case to reduce the maximum input power to 2.5 kW. This would reduce the size and cost of the solar array required to power the ion propulsion system. It also had critical side benefit of significantly improving the thruster life. This follows the well-known approach of trading thruster performance for improved thruster life. The next step along this path was the simplification of the thruster throttle capability.
The NSTAR ion thruster is capable of operating over a broad range of ion beam currents and voltages represented by an area on a graph of beam current vs beam voltage, with input power as a parameter as indicated in Fig. 2 . Roy Kakuda, a systems engineer at JPL, looked at the envelope of possible throttle points and said, “We cannot fly this. It will be impossible to flight qualify the thruster for operation at all these operating points.” We eventually settled on a much simpler throttling approach that maintained the specific impulse as high as possible for as long as possible as the input power was reduce. This was obtained by operating at a fixed beam voltage and reducing the beam current. When the limit of this approach was reached, the beam voltage was then reduced at a fixed low beam current. This throttling approach gave good performance over a range of deep space science missions of interest. Mission designers and thruster technologists quickly got used to it and the full throttling envelop was quietly forgotten.
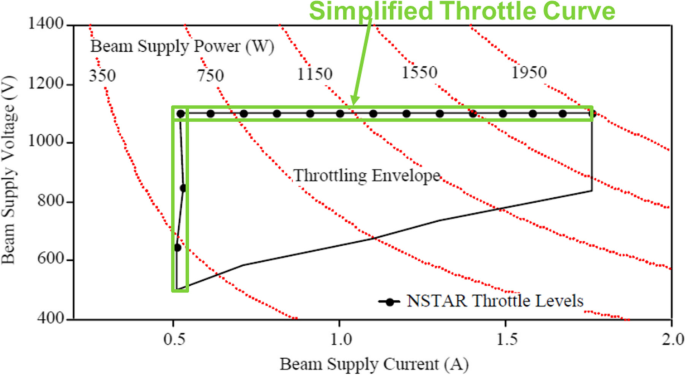
Throttling envelope capability for the NSTAR ion thruster and the simplified throttling curve used for flight
As luck would have it, just as SSTAR was being cancellation, NASA was initiating the New Millennium program designed specifically to test new technologies in space. The first of the New Millennium missions was designated Deep Space 1 (DS1 Footnote 3 ). Also, at this time the NASA Director Dan Goldin instituted what I refer to as the “Goldin Rule.” This rule states that NASA deep space science missions must account for the full life-cycle cost of the mission including the launch vehicle. Since one of the primary benefits of electric propulsion is to enable the use of smaller, less expensive launch vehicles, whether intended or not, the Goldin Rule immediately made electric propulsion an important technology at NASA. Prior to this, when I would talk to experienced project managers at JPL about the use of electric propulsion, the response was typically along the lines of, “Why would I want to risk my mission on your wacky new propulsion technology. I don’t care about it’s ability to enable the use of a smaller launch vehicle because I don’t pay for the launch vehicle anyway.”
With the Goldin Rule in place, Joel Sercel (who was at JPL at the time) and I approached the DS1 management team and informed them of the ongoing NSTAR project and how it could provide an ion propulsion system that DS1 could flight test. Since it was a separately funded project, DS1 would not have to pay for its development. We asserted that this would be the most important technology that DS1 could demonstrate. After some deliberation, the DS1 management came back to me and said they agreed that, “Ion propulsion is the most important technology we could demonstrate on DS1. Unfortunately, we can’t fly it because we can’t afford the large solar array it requires. However, if you give us a free solar array, we’ll fly the NSTAR ion propulsion system.”
What’s bad luck for some can be good luck for others. Around this time, BMDO launched the METEOR ( https://ntrs.nasa.gov/api/citations/20050177149/downloads/20050177149.pdf ) satellite to flight-test the linear concentrator solar array called SCARLET (Solar Concentrator Array with Refractive Linear Element Technology) they had been developing. Failure of the Conestoga launch vehicle on October 23, 1995 wound up plunging the satellite into the ocean. Recognizing that BMDO still had an interest in flight testing the SCARLET array, I approached Len Caveny and said they could get a free ride into space for the SCARLET array if they provided it to NASA’s DS1 mission. Len was skeptical that NASA would actually complete the NSTAR project and that failure to do so could result in the delay or cancellation of DS1. I suggested that if NASA runs into trouble with the NSTAR development, BMDO could step in and provide an SPT-100 system in its place. Len Caveny agreed to provide the SCARLET array for DS1. With a free solar array in hand, DS1 agreed to fly the NSTAR ion propulsion system. NASA successfully developed the NSTAR system on time and it ultimately operated for over 16,000 hours, providing a record-shattering ΔV to the spacecraft of 4.5 km/s.
Shortly after launch, however, it wasn’t clear that this would be the case. The first attempts to turn on the thruster failed due to a grid-to-grid short. Upon hearing this, Dan Goldin said, “Just turn on the other one.” A reasonable suggestion except there was only one NSTAR ion thruster on the spacecraft. The grid-to-grid short was cleared by changing the spacecraft attitude to alternately have the thruster face into and away from the sun, thermally cycling the grids.
DS1 demonstrated how to fly an SEP mission in deep space where the normal state of the spacecraft is thrusting with the ion propulsion system. This is very different from conventional spacecraft that coast the vast majority of the time. It identified the need for missed thrust margin and how to manage it. It identified and took advantage of the ability to exchange margins between mass, power, and missed thrust to minimize overall risk. It demonstrated how to navigate a low-thrust trajectory and it demonstrated that interactions between the IPS and the rest of the spacecraft including EMI, solar array current collection, and contamination from non-propellant efflux from the spacecraft were all manageable. Most importantly, it moved the perception of solar electric propulsion from an “advanced concept” to a legitimate option for deep space science missions.
NASA’s Dawn Footnote 4 mission was selected in the first Discovery Mission opportunity after the completion of the DS1 mission. It got off to a rocky start. As part of NASA’s competitive procurement process, each proposal team hosts a “Site Visit” for the review team to visit the proposer’s facility and have their outstanding questions addressed. For Dawn the site visit was to take place at Orbital Science’s (now Northup Grumman’s) facility in Dulles, WA. The site visit was scheduled on September 12, 2001. We were just starting a dress rehearsal on September 11, 2001 when the historic events of that day terminated the rehearsal and postponed the site visit for a few months. Since air traffic was grounded nationwide on September 11th, most of the JPL personnel at Orbital Sciences gathered together their largest rental cars and carpooled back to JPL in a 48-hr, non-stop trek across the country.
Ultimately, NASA informed Chris Russel, the Dawn Mission PI from UCLA, telling him, “The good news is that you’ve been selected. That bad news is that you have to wait a year before getting started.” This delayed the planned launch date from the summer of 2005 to the summer of 2006. Over the course of its implementation, Dawn would ultimately have three different project managers. The second manager, facing cost and schedule pressures, desperately wanted to eliminate the relatively expensive, complicated ion propulsion subsystem. In addition, Joe Makowski, the Orbital Sciences chief systems engineer would lament, “This propulsion system is so complicated, why do you want to go through all the trouble?” But, the rocket equation says that a dual main belt asteroid rendezvous mission is not practical without ion propulsion, and so it remained on the spacecraft.
Dawn was canceled twice due to projected cost overruns. The first time was at the confirmation review following the project’s preliminary design review (PDR). In acquiescence to the recommendation by NASA’s standing review board, the Dawn management team changed the mission design from a dual asteroid rendezvous mission to a single asteroid rendezvous plus a flyby of a second main belt asteroid in order to fit within the cost cap. At the confirmation review, the head of NASA’s Science Mission Directorate cancelled the mission saying, “You were selected because of the ability to rendezvous with two main belt asteroids. If you can’t do that, then the mission will not go forward.” To save the mission, Orbital Sciences agreed to give up their fee for the spacecraft development. This saved enough money that the Project could show that accomplishment of the dual asteroid rendezvous mission could be done within the available resources. The mission was then uncancelled 4 days after it was cancelled.
Dawn’s second cancellation was during Phase C/D, again due to projected cost overruns. JPL’s laboratory director, Charles Elachi, objected to the cancellation saying NASA did not follow its own process for cancelling the mission. A four-month investigation into the technical and financial status of the mission revealed that the mission had a high probability of successfully completing its development. Based on the findings from this review, NASA uncancelled Dawn. The delay, however, slipped the launch date from the summer of 2006 to June of 2007.
By June 2007, the Dawn spacecraft was fully fueled and sitting on top of its Delta II Heavy launch vehicle at Space Launch Complex 17-B (SLC 17-B) at the Cape Canaveral Air Force Station (now the Cape Canaveral Space Force Station). At the same time, NASA’s Phoenix spacecraft was on top of its Delta II launch vehicle at SLC 17-A (Fig. 3 ). The two launch complexes are so close together that NASA rules did not permit launching from one complex with a high-value payload on the other. Phoenix was headed for Mars and, consequently, had a tight constraint on its launch period. Dawn’s highly-capable ion propulsion system on the other hand provided much more flexibility in launch dates even though Dawn had to rendezvous with two main belt objects. The decision was made to take Dawn off the launch vehicle, launch Phoenix, then re-integrate Dawn with the launch vehicle. This slipped Dawn’s launch date to the end of September 2007.
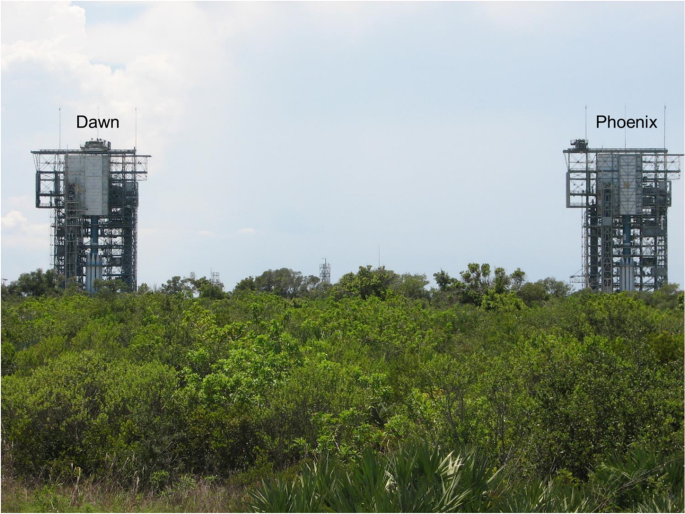
The Dawn spacecraft (SLC 17-B) and Phoenix spacecraft (SLC 17-A) at the Cape Canaveral Air Force Station in June 2007
One impact of this slip was that we had to do the final close-out of the ion propulsion system on top of the rocket twice, once in June 2007 and again in September 2007. In both cases, we performed these close-out activities from midnight to 3 am. One of the coolest things I have ever gotten to do was, instead of taking the elevator down from the white room (the room at the top that provides access to the spacecraft), we took the stairs down around the Delta-II Heavy rocket in the middle of the night.
The ion propulsion subsystem on Dawn was extremely successful. It operated for over 50,000 hours and provided a ΔV to the spacecraft of 11.5 km/s. It demonstrated without a doubt the long-awaited promise that solar electric propulsion could enable missions with characteristic velocities much higher than practical with chemical propulsion systems. Dawn demonstrated the ability to do orbit insertion with electric propulsion. It demonstrated orbit transfer using EP at bodies with initially poorly known gravity fields, and it was the first spacecraft ever to orbit to different target bodies.
SEP as a shiny new hammer
The successes of Deep Space 1, Dawn, SMART-1 ( https://en.wikipedia.org/wiki/SMART-1 ), and Hayabusa 1 and 2 ( https://en.wikipedia.org/wiki/Hayabusa , https://en.wikipedia.org/wiki/Hayabusa2 ), resulted in SEP being regarded as a “shiny new hammer” and mission designers went about looking for other nails to pound in. In parallel, over the last 20 years at JPL there was a concerted effort to develop models and simulations of the important performance and life-limiting phenomena taking place in gridded ion and Hall thrusters. This work produced codes like CEX [ 16 ], ORCA2D [ 17 ], Hall2de [ 18 ], etc. These models not only explained how thrusters work but are also used to guide development and scaling to other power levels and specific impulses that could then enable new mission concepts.
Many of these mission concepts, as is typical of most new mission concepts, didn’t make it to flight. This included the nuclear-powered Jupiter Icy Moons Orbiter (JIMO) mission [ 19 ], another round of human missions to Mars studies [ 20 ], and the Asteroid Redirect Mission (ARM) [ 21 ]. One of the interesting new results that came out of this latest round of human missions to Mars studies was the development of SEP/Chem hybrid architectures that could provide the mass savings of all-SEP systems with trip times comparable to all chemical propulsion systems [ 20 ]. Given the ongoing rapid development of solar array technology that is continuing the process of making solar arrays larger, higher power, less massive, and less expensive, my expectation is that when people do actually go to Mars they will use a SEP/Chem architecture.
The Asteroid Redirect Mission, which NASA came to call the Asteroid Redirect Robotic Mission (ARRM), would have used a high-power SEP system to catch up to, capture, and return to lunar orbit an entire small near-Earth asteroid (NEA). Small, in this case, refers to an asteroid roughly 8 m in diameter with a mass of several hundred metric tons. Difficulty in identifying and adequately characterizing such small objects led NASA to redefine the mission to pick a roughly 2-m diameter boulder off the surface of a larger NEA and return it to lunar orbit. The original ARM concept would have been humanity’s first attempt at altering the solar system for our benefit. ARM went from a $50 K, NASA-sponsored study in 2011 to part way through Phase B of a flagship-class directed mission by 2016 before beging cancelled due to lack of congressional support. Future asteroid mining activities may find the ARM approach attractive, in which an asteroid of several hundred tons is delivered to the Earth-Moon system where material extraction and benefaction processes can be developed and tested relatively close to home.
On a personal note, ARRM marked the third SEP mission I worked on in my career that was cancelled in Phase B: SEPS in 1981, Dawn in 2004, and ARRM in 2016. Fortunately, Dawn, the only competitively-selected mission in this group, was uncancelled as discussed above.
Present and near future
Solar electric propulsion is now firmly in the mainstream of propulsion options for both commercial satellites and deep space science missions. As of this writing there are more than 190 GeoComsats using Hall or ion thrusters [ 22 ] and over 2500 LEO satellites, most of which make up the Starlink constellation (that use krypton-fueled Hall thrusters) ( https://en.wikipedia.org/wiki/Starlink ). BepiColombo ( https://www.esa.int/Science_Exploration/Space_Science/BepiColombo_overview2 ) launched in 2018 is using its ion propulsion system to rendezvous with Mercury in 2025. The Psyche mission ( https://www.nasa.gov/psyche ), with a possible launch in 2023, will use a 20-kW solar array and SPT-140 thrusters to rendezvous with the main belt asteroid (16) Psyche. Two Janus spacecraft ( https://nssdc.gsfc.nasa.gov/nmc/spacecraft/display.action?id=JANUS ) originally planned to be launched with Psyche would use Field Emission Electric Propulsion thrusters to fly by two binary asteroids: 1996 FG3 and 1991 VH. NASA’s Power and Propulsion Element (PPE) of the Lunar Gateway [ 23 ], scheduled for launch in late 2024, will use a 60-kW solar array and a combination of 13-kW Hall thrusters [ 24 ] and 6-kW Hall thrusters [ 25 ] to deliver the PPE along with the Habitation and Logistics Outpost (HALO), to lunar orbit. Since the Lunar Gateway will be a human tended spacecraft, the PPE will represent the first use of EP in a system with astronauts onboard. With PPE/Gateway, electric propulsion applications will have expanded to impact human exploration of space. Finally, the Mars Sample Return ( https://www.airbus.com/en/newsroom/press-releases/2021-06-earth-return-orbiters-first-step-to-mars ) program plans to use ion propulsion for the ESA-provided Earth Return Orbiter to return the Mars samples to Earth. This would represent electric propulsion’s entry into multibillion-dollar flagship robotic missions.
On the horizon
NASA’s latest decadal survey [ 26 ] identifies the following missions to be included in the New Frontiers 6 announcement of opportunity: Centaur orbiter and lander; Ceres sample return; Comet surface sample return; Enceladus multiple flyby; Lunar Geophysical Network; Saturn probe; Titan orbiter; and Venus In Situ Explorer. Of these missions, Ceres sample return and Comet surface sample return are known to benefit significantly from the use solar electric propulsion (see [ 27 ] for example for the Ceres Sample Return). SEP may provide benefits to other missions in this list, but that remains to be determined. The decadal survey also identifies planetary defense as an area of national importance and specifically identifies ion beam deflection [ 28 ] as a planetary defense technique that should be developed.
The decadal survey also identifies the next flagship missions to follow the current Mars Sample Return program. Topping the list are an Enceladus orbiter and lander mission, and a Uranus orbiter and probe. Typically, missions to these solar ranges of roughly 10 and 20 AU, respectively, would use plutonium-powered, radioisotope thermoelectric generators (RTGs). However, solar arrays for space applications have continuously gotten, larger, lighter, less expensive, and able to operate farther from the Sun. Solar-powered missions to 5 AU are no longer unusual [ 29 , 30 , 31 ] and solar cells have been successfully tested under conditions simulating solar ranges as far as 30 AU (equivalent to Neptune’s orbit). Advances in solar array technology may make solar-powered missions to 10 and 20 AU feasible and more affordable than RTG-powered missions. Coupling very large, lightweight solar arrays with electric propulsion may ultimately enable non-nuclear exploration throughout the solar system [ 32 ].
Farther in the future, asteroid mining has long been known to largely be a transportation problem [ 33 ]. Cost-effective transportation using some form of high-power solar electric propulsion will likely be required for viable asteroid mining operations.
Conclusions
The long-awaited promise of electric propulsion and its ability to reduce overall mission costs and enable high ΔV missions is being fulfilled. Electric propulsion is in the process of infiltrating every aspect of space exploration and exploitation from cubesats and constellations of LEO satellites to Geo comsats, competed and flagship robotic deep space science missions, and even human space exploration missions. This feat is the result of three key developments. First and foremost is the development of affordable solar arrays that provide the power needed by EP systems. Second is the development of EP thruster technologies that have sufficient life to accomplish missions of interest. Third is the demonstration that EP systems are compatible with the rest of the spacecraft in terms of EMI/EMC, contamination, and guidance, navigation, and control.
The fact that EP has infiltrated all aspects of space endeavors does not mean that its best days are behind it. Quite the contrary, the best days for electric propulsion are most certainly in the future. This is driven by the inescapable fact that the solar system is big and that there will always be a desire to get around it faster. This will drive missions to ever higher ΔV’s driving the need for significantly better EP systems. Accompanying this drive to better EP systems will be a corresponding development of ever larger, lighter, less expensive solar arrays to provide the required power.
Availability of data and materials
Not applicable.
There were also multimegawatt solar powered concepts, but these were not photovoltaic power sources, but rather assumed the collection of solar power to drive dynamic power conversion systems.
This decade also saw the demonstration of attractive service life capability for the SPT-100 (Hall) thruster and the 25-cm diameter XIPS (Xenon Ion Propulsion System) commercial ion thruster.
Deep Space 1 is abbreviated DS1 not DS-1. As Marc Rayman explained, this was in the time of faster, better, cheaper, and DS1 has 25% fewer key strokes than DS-1.
Dawn is not an acronym and should not be written in all caps, i.e. DAWN. The idea of the mission to visit (4) Vesta and (1) Ceres is that these objects contain information that dates back to the dawn of the solar system.
The tyranny of the rocket equation | Don Pettit | TEDxHouston (2013), https://www.youtube.com/watch?v=uWjdnvYok4I
Kimmel, W. M, et al., “Technology Readiness Assessment Best Practices,” SP-20205003605, 2020
Google Scholar
Thomas VC et al (2011) The Dawn spacecraft. Space Sci Rev 163:175–249. https://doi.org/10.1007/s1214-011-9852-2
Article ADS Google Scholar
Brophy JR et al (2008) Lifetime Qualification of Electric Thrusters for Deep-Space Missions, AIAA-2008-5184, 44 th AIAA/ASMESAE/ASEE Joint Propulsion Conference. American Institute of Aeronautics and Astronautics (AIAA), Hartford
Patterson MJ et al (1995) 2.3 kW Ion Thruster Wear Test, AIAA-95-2516, 31st Joint Propulsion Conference
Polk JE et al (1996) A 1000-hour Wear test of the NASA NSTAR ion thruster, AIAA-962717, 32nd Joint Propulsion Conference
Garner C et al (1995) A 5,730-hr Cyclic Endurance Test of the SPT-100, AIAA-95-2667, presented at the 31st AIAA/ASME/SAE/ASEE Joint Propulsion Conference, San Diego
Polk JE et al (1999) An Overview of the Results from an 8200 Hour Wear Test of the NSTAR Ion Thruster, AIAA-99-2446, presented at the 35th AIAA/ASME/SAE/ASEE Joint Propulsion Conference, Los Angeles
Sengupta A et al (2004) An overview of the results from the 30,000 Hr life test of deep space 1 flight spare ion engine, AIAA-2004-3608, presented at the 40th AIAA/ASME/SAE/ASEE joint propulsion conference and exhibit, Fort Lauderdale
Austin RE, Dod RE, Grim D (1978) Solar Electric Propulsion for the Halley’s Comet Rendezvous Mission: Foundation for Future Missions, AIAA-78-83, AIAA 16 th Aerospace Science Conference, Huntsville
Friedman L et al (1978) Solar Sailing—The Concept Made Realistic, AIAA-78-82, AIAA 16 th Aerospace Science Conference, Huntsville
Austin RE (1979) Solar Electric Propulsion System (SEPS): Tomorrow’s Propulsion System—Today, AIAA-79-2045, Princeton/AIAA/DGLR 14 th International Electric Propulsion Conference, Princeton
Bechtel RT (1969) Performance and control of a 30 cm diameter low impulse Kaufman thruster, paper no. 691238. AIAA, New York
Patterson MJ, Verhey TR (1990) 5kW Xenon Ion Thruster Lifetest, AIAA-90-2543, AIAA/DGLR/JSASS 21 st International Electric Propulsion Conference, Orlando
Jones JM, Einhorn RS (1993) The USAF Electric Propulsion System Activities. https://doi.org/10.2514/6.1993-1937
Book Google Scholar
Polk J et al (2021) Modeling grid Erosion in the NEXT ion thruster using the CEX2D and CEX3D codes. Springer Nature. https://doi.org/10.21203/rs.3.rs-1517801/v1
Mikellides I et al (2006) Toward the identification of the Keeper Erosion Cause(s): numerical simulations of the Plasma and Neutral Gas using the Global Cathode Model OrCa2D-II, 42 nd AIAA/ASMS/SAE/ASEE Joint Propulsion Conference and Exhibit, Sacramento
Mikellides I et al (2016) Hall2De Simulations with a First-principles Electron Transport Model Based on the Electron Cyclotron Drift Instability, AIAA 2016-4618. https://doi.org/10.2514/6.2016-4618
Randolph TM et al The Prometheus 1 Spacecraft Preliminary Electric Propulsion System Design, AIAA-2005-3889, 41 st AIAA/ASME/SAE/ASEE Joint Propulsion Conference, Tucson
Merrill RG, Strange N, Qu M, Hatten N (2015) Mars conjunction crewed missions with a reusable hybrid architecture, 2015 IEEE Aerospace Conference, pp 1–14. https://doi.org/10.1109/AERO.2015.7118956
Brophy JR, Friedman L, Culick F (2012) Asteroid retrieval feasibility, 2012 IEEE Aerospace Conference, pp 1–16. https://doi.org/10.1109/AERO.2012.6187031
Lev D et al (2019) The technological and commercial expansion of electric propulsion. Acta Astronaut 159(2019):213–227
Ticker R et al (2019) The Gateway Power and Propulsion Element: Setting the Foundation for Exploration and Commerce, AIAA Propulsion and Energy Forum, Indianapolis
Jackson J et al (2019) 13kW Advanced Electric Propulsion Flight System Development and Qualification, IEPC-2019-692, Presented at the 36th International Electric Propulsion Conference. University of Vienna, Vienna
Mullins C et al (2019) Development of a 5kW Class Hall Thruster, IEPC-2019-492, Presented at the 36th International Electric Propulsion Conference. University of Vienna, Vienna
National Academies of Sciences, Engineering, and Medicine 2022 (2023-2032) Origins, Worlds, and Life: A Decadal Strategy for Planetary Science and Astrobiology. The National Academies Press, Washington, DC. https://doi.org/10.17226/26522
Castillo-Rogez J et al (2022) Planet. Sci J 3:41
Abell P et al (2021) Planetary defense missions, rapid Mission architecture study, Planetary Science Decadal Survey, Mission Concept Study Report
Taylor MGGT, Altobelli N, Buratti BJ, Choukroun M (2017) The Rosetta mission orbiter science overview: the comet phase. Phil Trans R Soc A.3752016026220160262. https://doi.org/10.1098/rsta.2016.0262
Bolton SJ, Lunine J, Stevenson D et al (2017) The Juno Mission. Space Sci Rev 213:5–37. https://doi.org/10.1007/s11214-017-0429-6
Levison HF et al (2021) Lucy Mission to the Trojan asteroids: science goals. Planet Sci J 2:171
Article Google Scholar
Brophy J, Pellegrino S, Lubin P (2022) Non-Nuclear Exploration of the Solar System. Final Workshop Report for the W.M. Keck Institute for Space Studies (KISS), Pasadena. https://doi.org/10.7907/h62p-6328
Brophy JR, Oleson S (2012) Spacecraft Conceptual Design for Returing Entire Near-Earth Asteroids, AIAA-2012-4067, 48th AIAA/ASME/SAE/ASEE Joint Propulsion Conference & Exhibit and 10th International Energy Conversion Engineering Conference, Atlanta
Download references
Acknowledgements
Part of the research was carried out at the Jet Propulsion Laboratory, California Institute of Technology, under a contract with the National Aeronautics and Space Administration (80NM0018D0004). Dan Goebel’s review of a draft version of this paper and his helpful comments are gratefully acknowledged.
Code availability
Part of the research was carried out at the Jet Propulsion Laboratory, California Institute of Technology, under a contract with the National Aeronautics and Space Administration (80NM0018D0004).
Author information
Authors and affiliations.
Jet Propulsion Laboratory, California Institute of Technology, Pasadena, CA, 91109, USA
John R. Brophy
You can also search for this author in PubMed Google Scholar
Contributions
The author wrote and approved the version of the paper.
Corresponding author
Correspondence to John R. Brophy .
Ethics declarations
Ethics approval and consent to participate.
The author has read and agreed to its content and is accountable for all aspects of the accuracy and integrity of the manuscript in accordance with ICMJE criteria. The manuscript is original, has not already been published, and is not currently under consideration by another journal.
Consent for publication
Competing interests, additional information, publisher’s note.
Springer Nature remains neutral with regard to jurisdictional claims in published maps and institutional affiliations.
Rights and permissions
Open Access This article is licensed under a Creative Commons Attribution 4.0 International License, which permits use, sharing, adaptation, distribution and reproduction in any medium or format, as long as you give appropriate credit to the original author(s) and the source, provide a link to the Creative Commons licence, and indicate if changes were made. The images or other third party material in this article are included in the article's Creative Commons licence, unless indicated otherwise in a credit line to the material. If material is not included in the article's Creative Commons licence and your intended use is not permitted by statutory regulation or exceeds the permitted use, you will need to obtain permission directly from the copyright holder. To view a copy of this licence, visit http://creativecommons.org/licenses/by/4.0/ .
Reprints and permissions
About this article
Brophy, J.R. Perspectives on the success of electric propulsion. J Electr Propuls 1 , 9 (2022). https://doi.org/10.1007/s44205-022-00011-0
Download citation
Received : 01 June 2022
Accepted : 10 July 2022
Published : 09 September 2022
DOI : https://doi.org/10.1007/s44205-022-00011-0
Share this article
Anyone you share the following link with will be able to read this content:
Sorry, a shareable link is not currently available for this article.
Provided by the Springer Nature SharedIt content-sharing initiative
- Electric propulsion
- Ion propulsion
- Ion thruster
- Hall thruster
- Find a journal
- Publish with us
- Track your research
Future directions for electric propulsion research
The research challenges for electric propulsion technologies are examined in the context of s-curve development cycles. It is shown that the need for research is driven both by the application as well as relative maturity of the technology. For flight qualified systems such as moderately-powered Hall thrusters and gridded ion thrusters, there are open questions related to testing fidelity and predictive modeling. For less developed technologies like large-scale electrospray arrays and pulsed inductive thrusters, the challenges include scalability and realizing theoretical performance. Strategies are discussed to address the challenges of both mature and developed technologies. With the aid of targeted numerical and experimental facility effects studies, the application of data-driven analyses, and the development of advanced power systems, many of these hurdles can be overcome in the near future.
Duke Scholars
Altmetric Attention Stats
Dimensions citation stats, published in, publication date, related subject headings.
- 4001 Aerospace engineering
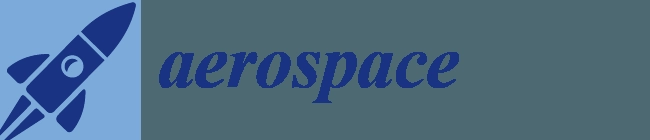
Future Directions for Electric Propulsion Research
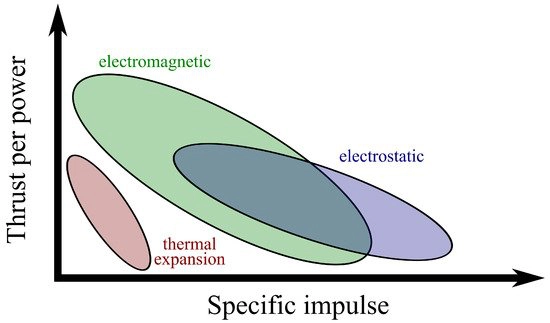
Publisher URL: https://www.mdpi.com/2226-4310/7/9/120
Open URL: https://www.mdpi.com/2226-4310/7/9/120/pdf
DOI: 10.3390/aerospace7090120
Keeping up-to-date with research can feel impossible, with papers being published faster than you'll ever be able to read them. That's where Researcher comes in: we're simplifying discovery and making important discussions happen. With over 19,000 sources, including peer-reviewed journals, preprints, blogs, universities, podcasts and Live events across 10 research areas, you'll never miss what's important to you. It's like social media, but better. Oh, and we should mention - it's free.

Researcher displays publicly available abstracts and doesn’t host any full article content. If the content is open access, we will direct clicks from the abstracts to the publisher website and display the PDF copy on our platform. Clicks to view the full text will be directed to the publisher website, where only users with subscriptions or access through their institution are able to view the full article.
share this!
May 10, 2021
A new era of spaceflight? Promising advances in rocket propulsion
by Gareth Dorrian and Ian Whittaker, The Conversation
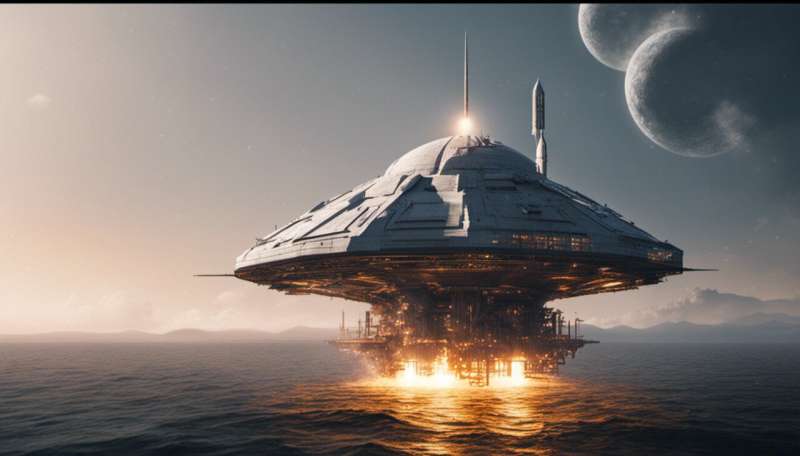
The US Defense Advanced Research Projects Agency (Darpa) has recently commissioned three private companies, Blue Origin, Lockheed Martin and General Atomics, to develop nuclear fission thermal rockets for use in lunar orbit.
Such a development, if flown, could usher in a new era of spaceflight. That said, it is only one of several exciting avenues in rocket propulsion . Here are some others.
Chemical rockets
The standard means of propulsion for spacecraft uses chemical rockets. There are two main types: solid fuelled (such as the solid rocket boosters on the Space Shuttle), and liquid fuelled (such as the Saturn V ).
In both cases, a chemical reaction is employed to produce a very hot, highly pressurized gas inside a combustion chamber. The engine nozzle provides the only outlet for this gas which consequently expands out of it, providing thrust.
The chemical reaction requires a fuel, such as liquid hydrogen or powdered aluminum, and an oxidiser (an agent that produces chemical reactions) such as oxygen. There are many other variables which ultimately also determine the efficiency of a rocket engine, and scientists and engineers are always looking to get more thrust and fuel efficiency out of a given design.
Recently, private company SpaceX has been conducting test flights of their Starship launcher prototype. This vehicle uses a "full-flow staged combustion (FFSC) engine," the Raptor , which burns methane for fuel and oxygen for oxidiser. Such designs were tested by the Russians in the 1960s and the US government in the 2000s, but as yet none has flown in space. The engines are much more fuel efficient and can generate a much higher thrust-to-weight ratio than traditional designs.
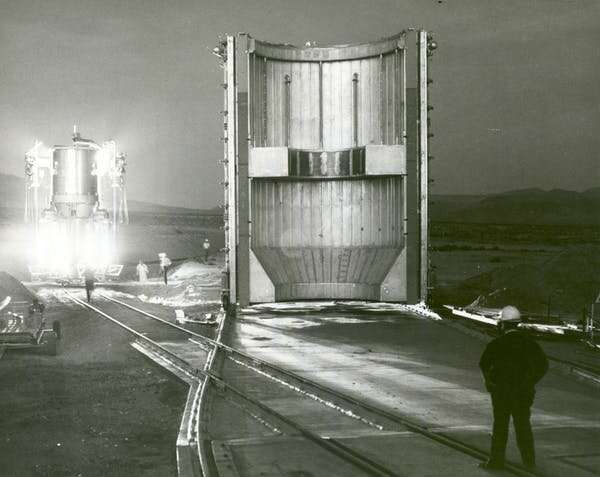
Fission thermal rockets
The nucleus of an atom consists of sub-atomic particles called protons and neutrons. These determine the mass of an element—the more protons and neutrons, the heavier it is. Some atomic nuclei are unstable and can be split into several smaller nuclei when bombarded with neutrons. This is the process of nuclear fission , and it can release an enormous amount of energy. As the nuclei decay, they also release more neutrons which go on to fissure more atoms—producing a chain reaction.
In a nuclear fission thermal rocket, a propellant gas, such as hydrogen, is heated by nuclear fission to high temperatures, creating a high pressure gas within the reactor chamber. Like with chemical rockets, this can only escape via the rocket nozzle, again producing thrust. Nuclear fission rockets are not envisaged to produce the kind of thrust necessary to lift large payloads from the surface of the Earth into space. Once in space though, they are much more efficient than chemical rockets—for a given mass of propellant, they can accelerate a spacecraft to much higher speeds.
Nuclear fission rockets have never been flown in space, but they have been tested on the ground. They should be able to shorten flight times between Earth and Mars from some seven months to about three months for future crewed missions. Obvious drawbacks, however, include the production of radioactive waste, and the possibility of a launch failure which could result in radioactive material being spread over a wide area.
A major engineering challenge is to sufficiently miniaturize a reactor so that it will fit on a spacecraft. There is already a burgeoning industry in the production of compact fission reactors, including the development of a fission reactor which is smaller than an adult human .
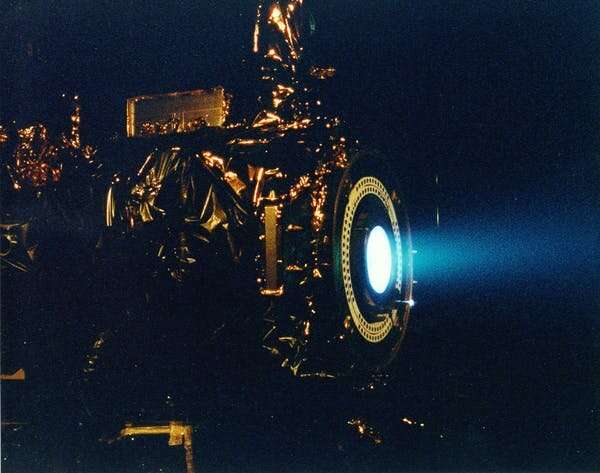
Electric propulsion
A staple of science fiction , real ion drives generate charged particles (ionization), accelerate them using electric fields and then fire them from a thruster. The propellant is a gas such as xenon, a fairly heavy element that can be easily electrically charged.
As the charged xenon atoms accelerate out of the thruster, they transfer a very small amount of momentum (the product of mass and velocity) to the spacecraft, providing gentle thrust. While slow, ion drives are among the most fuel-efficient of all spacecraft propulsion methods, so could get us further. Ion drives are commonly used for attitude control (changing which direction a spacecraft is facing) and have been considered for deorbiting old satellites .
Current ion engines are powered by solar cells , effectively making them solar powered, and requiring very little propellant. They have been used on Esa's SMART-1 mission to the Moon and Bepi-Colombo mission en-route to Mercury. Nasa are currently developing a high power electric propulsion system for the Lunar Gateway , an outpost which will orbit the Moon.
Solar sails
While propulsion usually requires propellant of some description, a more "green" method relying only on light from the Sun itself.
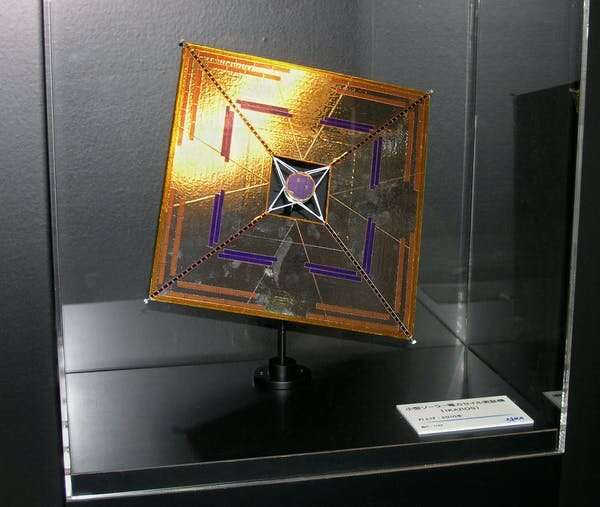
Sails rely on the physical property of conservation of momentum. On Earth, we are used to seeing this momentum as a dynamic pressure from air particles blowing into a sheet when sailing, propelling a vessel forwards . Light is comprised of photons , which have no mass, but they do have momentum and can transfer it to a sail. As the energies of individual photons are very small, an extremely large sail size is needed for any appreciable acceleration.
The speed gain will also depend on how far from the Sun you are. At Earth, the power received from sunlight is about 1.3 kW per square meter. If we had a sail the size of a football pitch, this would equate to 9.3 MW, providing a very low acceleration, even to a low mass object.
Solar sails have been tested by the Japanese IKAROS spacecraft which successfully flew by Venus, and the Planetary Society Lightsail-2 , which is presently in orbit around Earth.
A way of improving efficiency and reducing sail size is to use a laser to propel the spacecraft forward . Lasers produce very intense beams of photons which can be directed onto a sail to provide much higher acceleration, but would require being built in Earth orbit to avoid loss of intensity in the atmosphere. Lasers have also been proposed as a means of de-orbiting space junk—the light from the laser can slow down a piece of orbital junk, which would then fall out of orbit and burn up in the atmosphere.
The development of nuclear fission rockets may excite some and concern others. However, as private companies and national space agencies are increasingly committing to a sustained human presence in space, these alternative means of propulsion will become more mainstream and have the potential to revolutionize our nascent space-faring civilisation.
Provided by The Conversation
Explore further
Feedback to editors

Study reveals how humanity could unite to address global challenges
2 hours ago

CO₂ worsens wildfires by helping plants grow, model experiments show
3 hours ago

Surf clams off the coast of Virginia reappear and rebound
4 hours ago

Yellowstone Lake ice cover unchanged despite warming climate
5 hours ago

The history of the young cold traps of the asteroid Ceres

Researchers shine light on rapid changes in Arctic and boreal ecosystems

New benzofuran synthesis method enables complex molecule creation

Human odorant receptor for characteristic petrol note of Riesling wines identified

Uranium-immobilizing bacteria in clay rock: Exploring how microorganisms can influence the behavior of radioactive waste

Research team identifies culprit behind canned wine's rotten egg smell
Relevant physicsforums posts, recommendations for international research competitions, will we ever communicate with extraterrestial life in a reasonable time frame.
6 hours ago
Our Beautiful Universe - Photos and Videos
10 hours ago
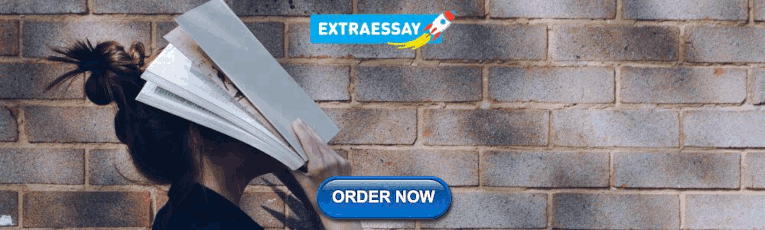
How to use the Binary Mass Function for exoplanets?
17 hours ago
U.S. Solar Eclipses - Oct. 14, 2023 (Annular) & Apr. 08, 2024 (Total)
Apr 14, 2024
How fast were distant galaxies moving?
More from Astronomy and Astrophysics
Related Stories

To safely explore the solar system and beyond, spaceships need to go faster—nuclear-powered rockets may be the answer
May 20, 2020

SpaceX vs NASA: Who will get us to the moon first? Here's how their latest rockets compare
Jan 29, 2021

Earth to Mars in 100 days: The power of nuclear rockets
Jul 1, 2019

Flight by Light: Mission accomplished for LightSail 2
Aug 1, 2019

A CubeSat will test out water as a propulsion system
Jan 27, 2021

NASA Reignites Program for Nuclear Thermal Rockets
Aug 14, 2017
Recommended for you

NASA confirms mystery object that crashed through roof of Florida home came from space station

NASA is seeking a faster, cheaper way to bring Mars samples to Earth

NASA unveils probe bound for Jupiter's possibly life-sustaining moon
Apr 12, 2024

A new type of seismic sensor to detect moonquakes

A total solar eclipse races across North America as clouds part along totality
Apr 8, 2024

What do scientists hope to learn from total solar eclipse in US?
Apr 7, 2024
Let us know if there is a problem with our content
Use this form if you have come across a typo, inaccuracy or would like to send an edit request for the content on this page. For general inquiries, please use our contact form . For general feedback, use the public comments section below (please adhere to guidelines ).
Please select the most appropriate category to facilitate processing of your request
Thank you for taking time to provide your feedback to the editors.
Your feedback is important to us. However, we do not guarantee individual replies due to the high volume of messages.
E-mail the story
Your email address is used only to let the recipient know who sent the email. Neither your address nor the recipient's address will be used for any other purpose. The information you enter will appear in your e-mail message and is not retained by Phys.org in any form.
Newsletter sign up
Get weekly and/or daily updates delivered to your inbox. You can unsubscribe at any time and we'll never share your details to third parties.
More information Privacy policy
Donate and enjoy an ad-free experience
We keep our content available to everyone. Consider supporting Science X's mission by getting a premium account.
E-mail newsletter
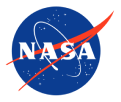
Suggested Searches
- Climate Change
- Expedition 64
- Mars perseverance
- SpaceX Crew-2
- International Space Station
- View All Topics A-Z
Humans in Space
Earth & climate, the solar system, the universe, aeronautics, learning resources, news & events.
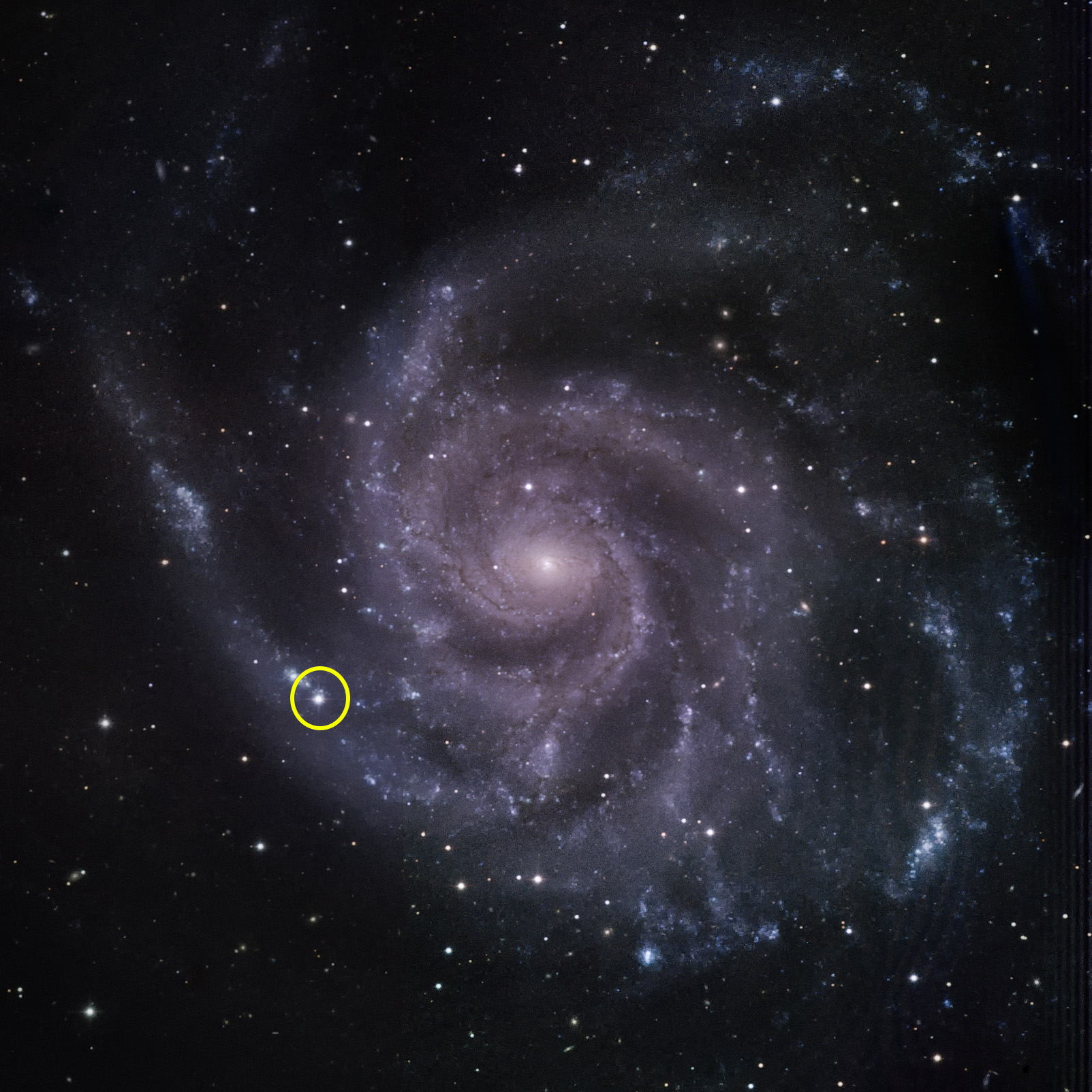
NASA’s Fermi Mission Sees No Gamma Rays from Nearby Supernova
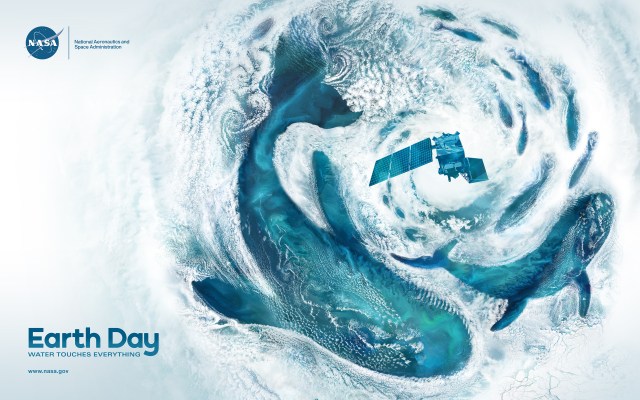
The Ocean Touches Everything: Celebrate Earth Day with NASA
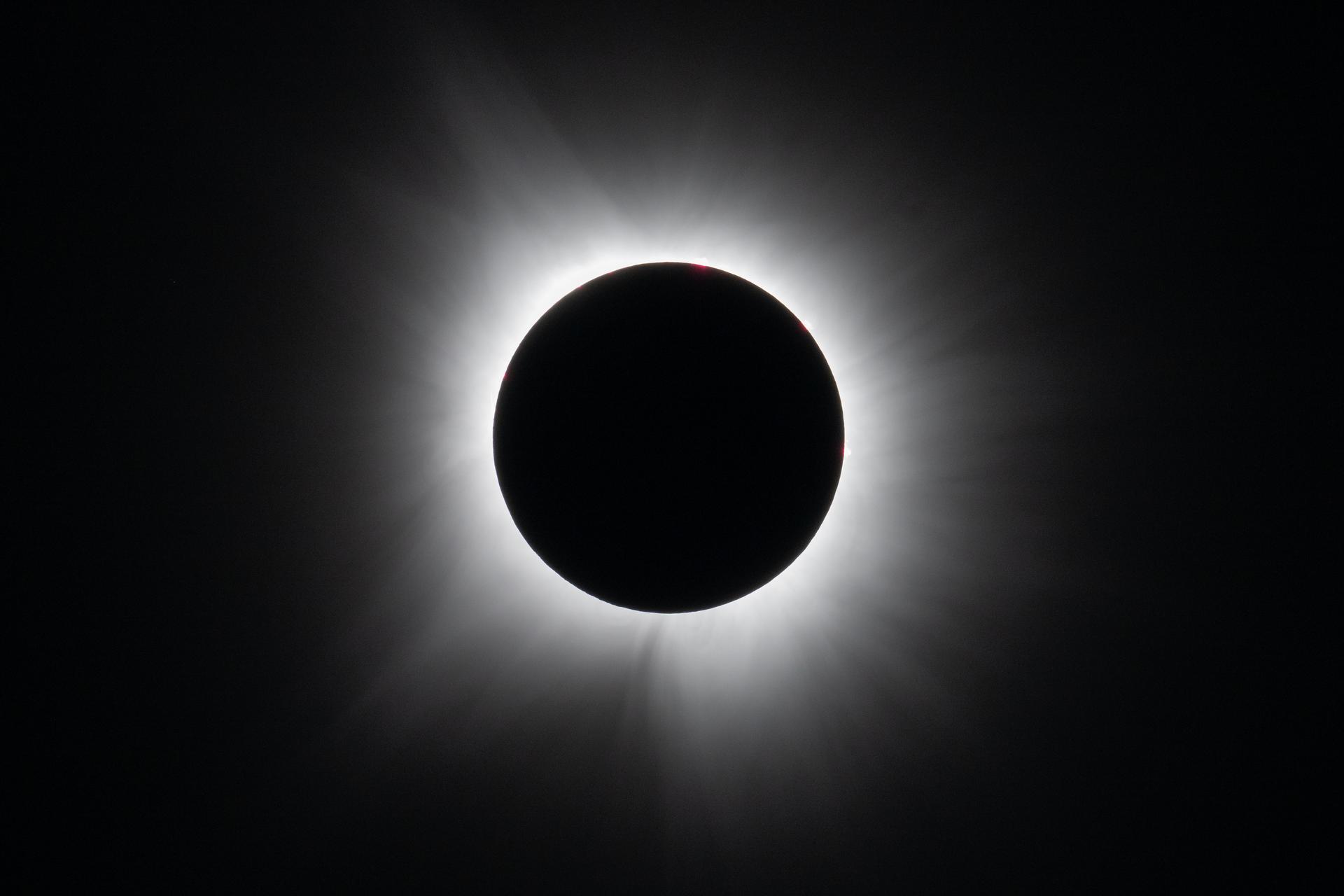
The April 8 Total Solar Eclipse: Through the Eyes of NASA
- Search All NASA Missions
- A to Z List of Missions
- Upcoming Launches and Landings
- Spaceships and Rockets
- Communicating with Missions
- James Webb Space Telescope
- Hubble Space Telescope
- Why Go to Space
- Astronauts Home
- Commercial Space
- Destinations
- Living in Space
- Explore Earth Science
- Earth, Our Planet
- Earth Science in Action
- Earth Multimedia
- Earth Science Researchers
- Pluto & Dwarf Planets
- Asteroids, Comets & Meteors
- The Kuiper Belt
- The Oort Cloud
- Skywatching
- The Search for Life in the Universe
- Black Holes
- The Big Bang
- Dark Energy & Dark Matter
- Earth Science
- Planetary Science
- Astrophysics & Space Science
- The Sun & Heliophysics
- Biological & Physical Sciences
- Lunar Science
- Citizen Science
- Astromaterials
- Aeronautics Research
- Human Space Travel Research
- Science in the Air
- NASA Aircraft
- Flight Innovation
- Supersonic Flight
- Air Traffic Solutions
- Green Aviation Tech
- Drones & You
- Technology Transfer & Spinoffs
- Space Travel Technology
- Technology Living in Space
- Manufacturing and Materials
- Science Instruments
- For Kids and Students
- For Educators
- For Colleges and Universities
- For Professionals
- Science for Everyone
- Requests for Exhibits, Artifacts, or Speakers
- STEM Engagement at NASA
- NASA's Impacts
- Centers and Facilities
- Directorates
- Organizations
- People of NASA
- Internships
- Our History
- Doing Business with NASA
- Get Involved
- Aeronáutica
- Ciencias Terrestres
- Sistema Solar
- All NASA News
- Video Series on NASA+
- Newsletters
- Social Media
- Media Resources
- Upcoming Launches & Landings
- Virtual Events
- Sounds and Ringtones
- Interactives
- STEM Multimedia
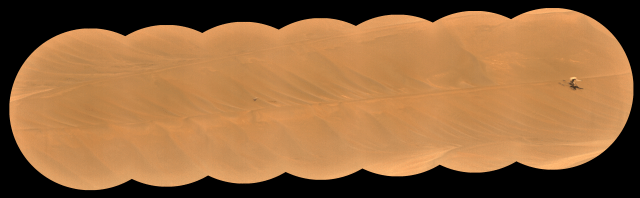
NASA’s Ingenuity Mars Helicopter Team Says Goodbye … for Now
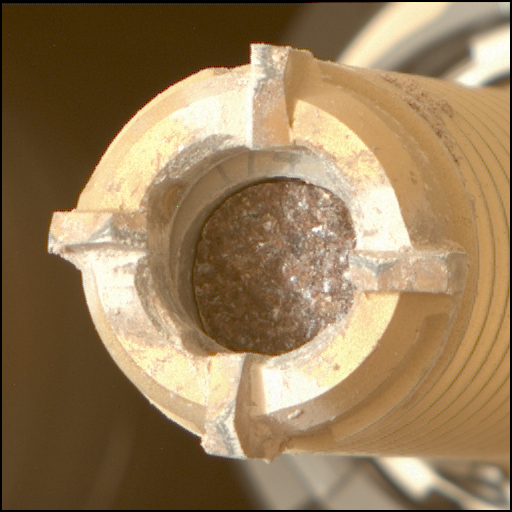
Comet Geyser: Perseverance’s 24th Rock Core
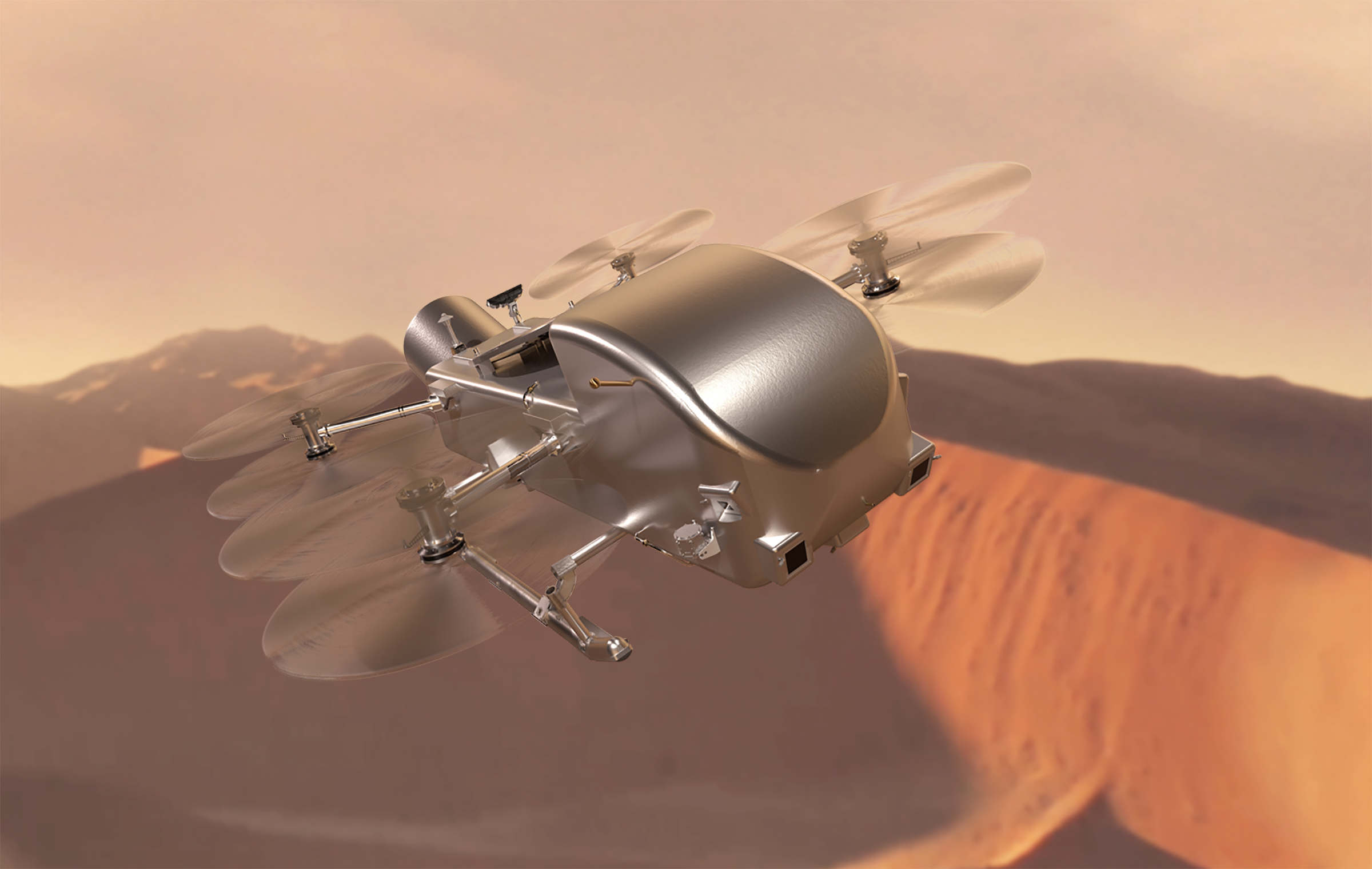
NASA’s Dragonfly Rotorcraft Mission to Saturn’s Moon Titan Confirmed
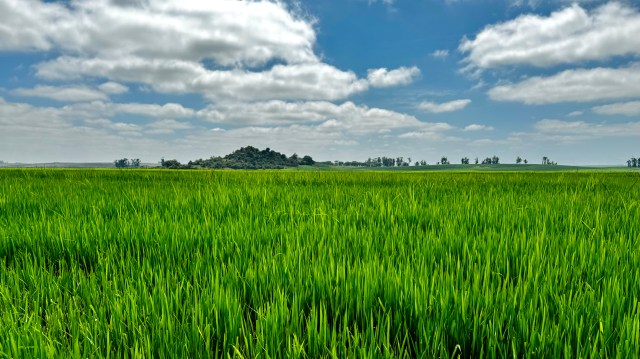
NASA Open Science Initiative Expands OpenET Across Amazon Basin
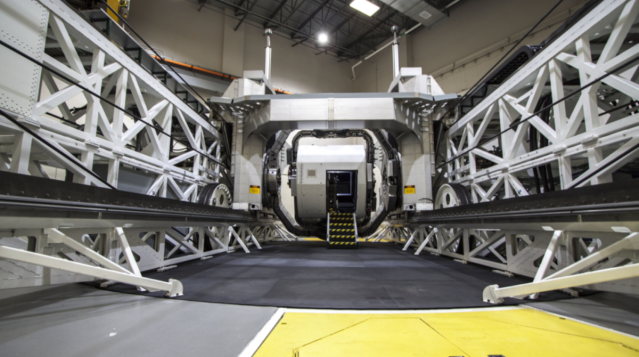
NASA Motion Sickness Study Volunteers Needed!
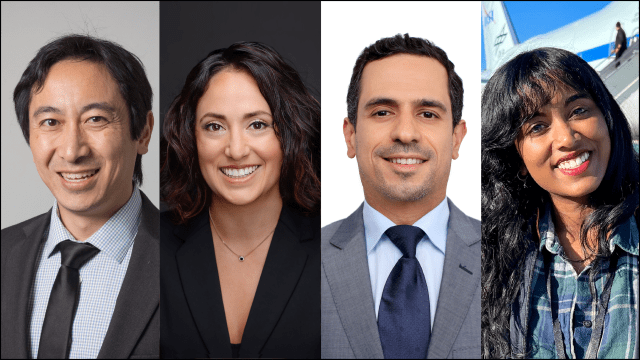
NASA Selects New Crew for Next Simulated Mars Journey
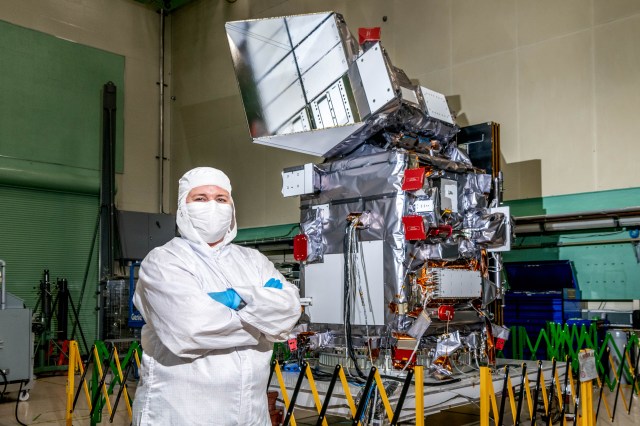
Kate A. McGinnis: Ready to “Go” with PACE Testing
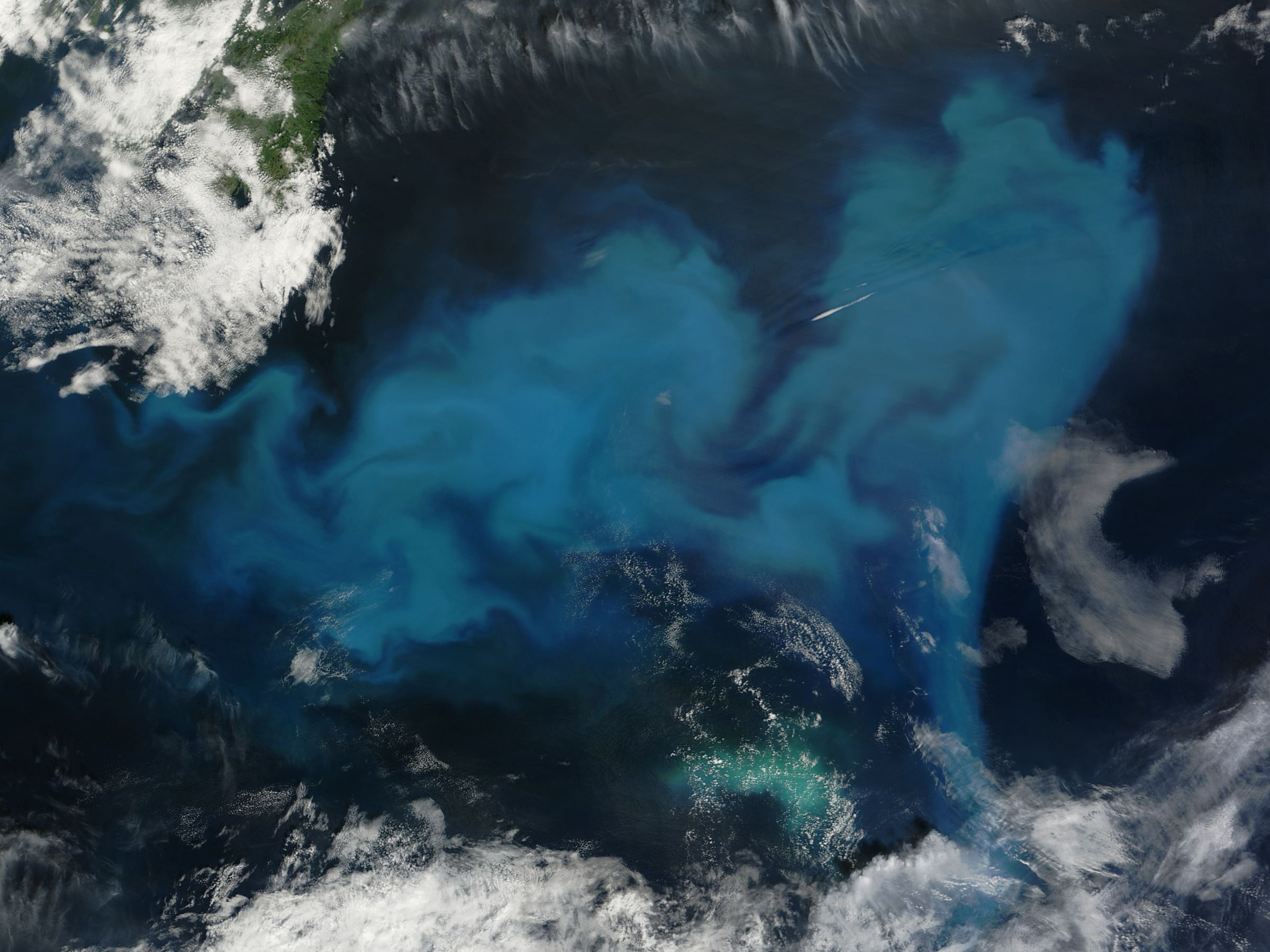
A.3 Ocean Biology and Biogeochemistry Inclusion Plan Correction
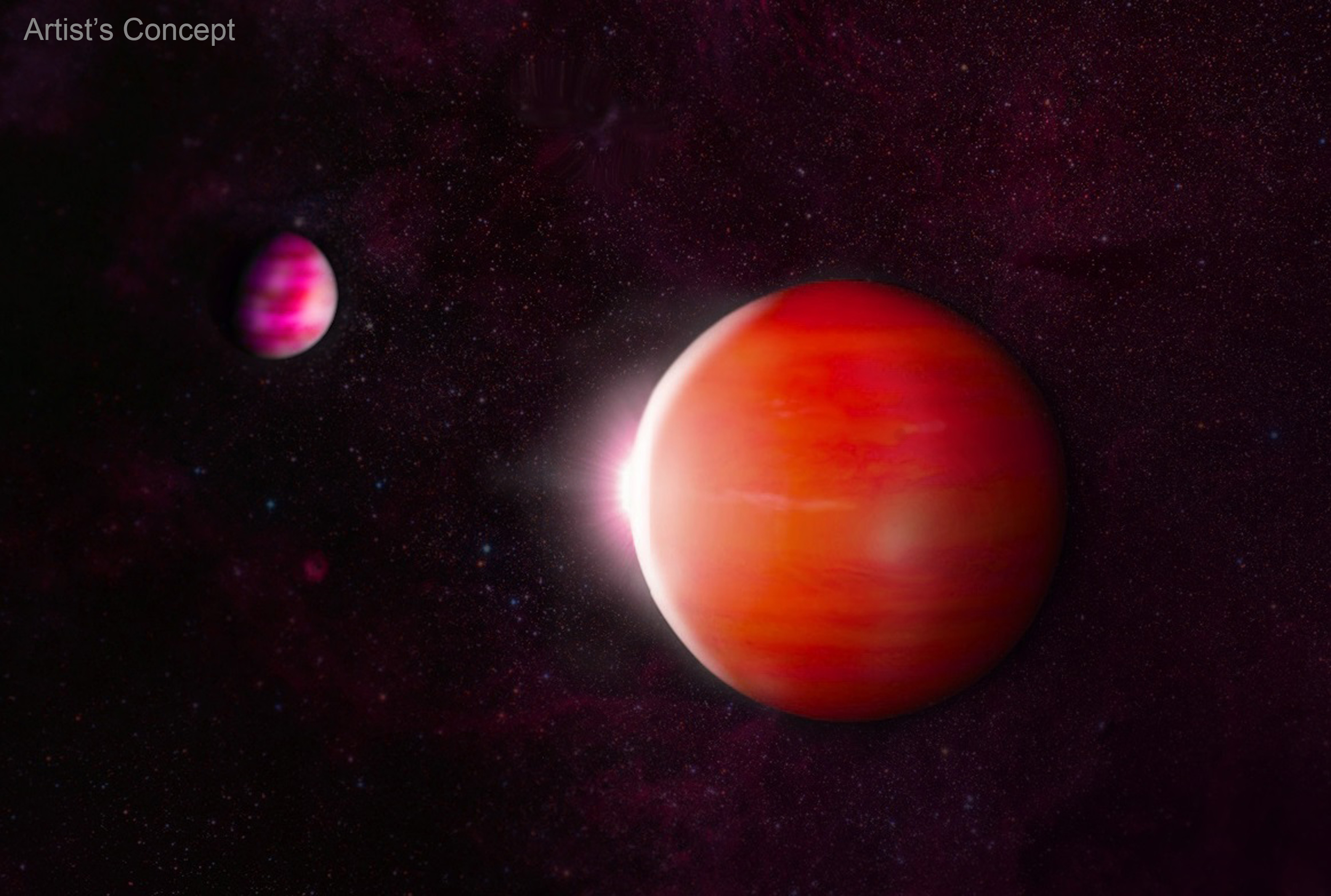
A Solar Neighborhood Census, Thanks to NASA Citizen Science
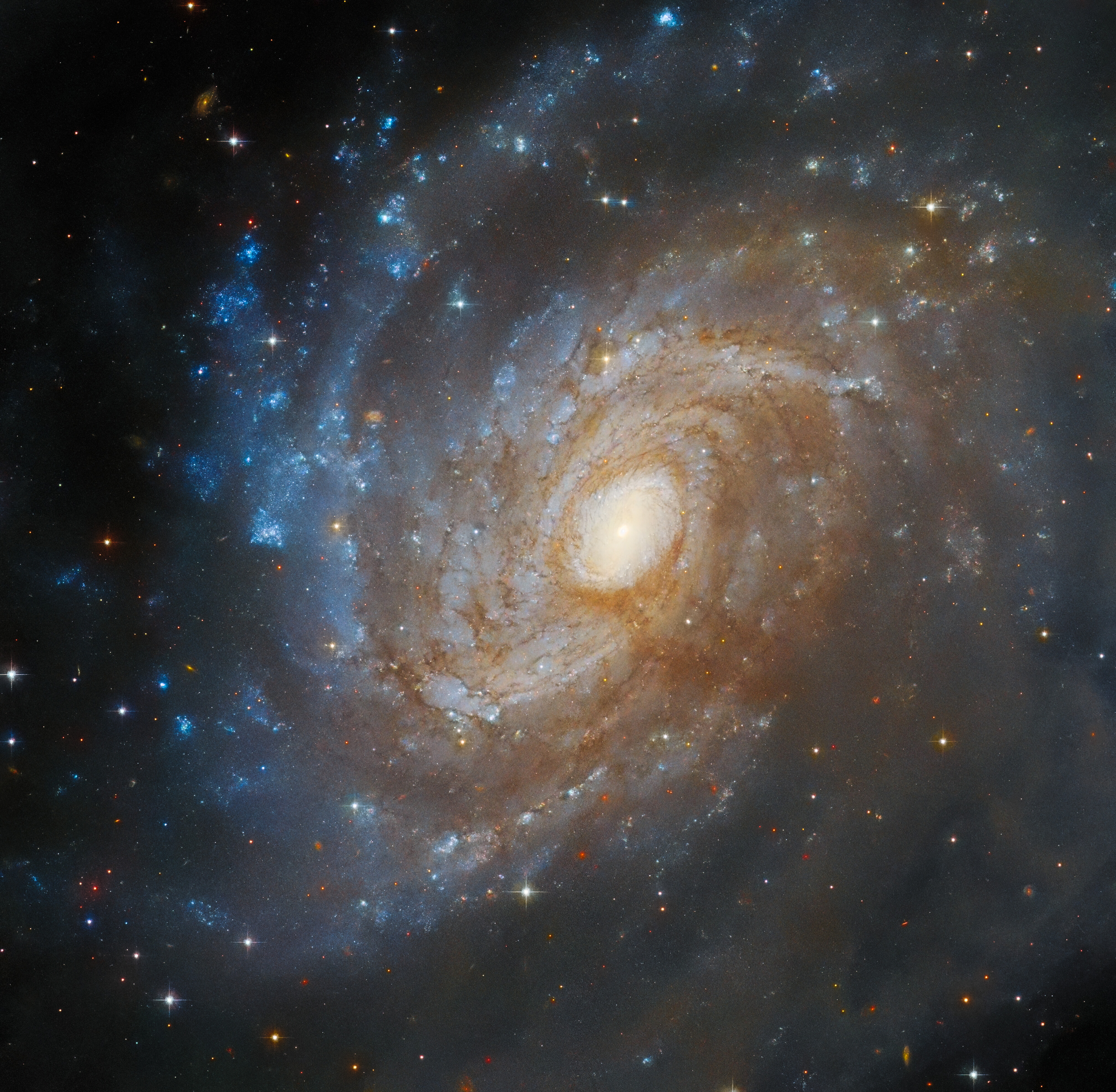
Hubble Spots a Galaxy Hidden in a Dark Cloud
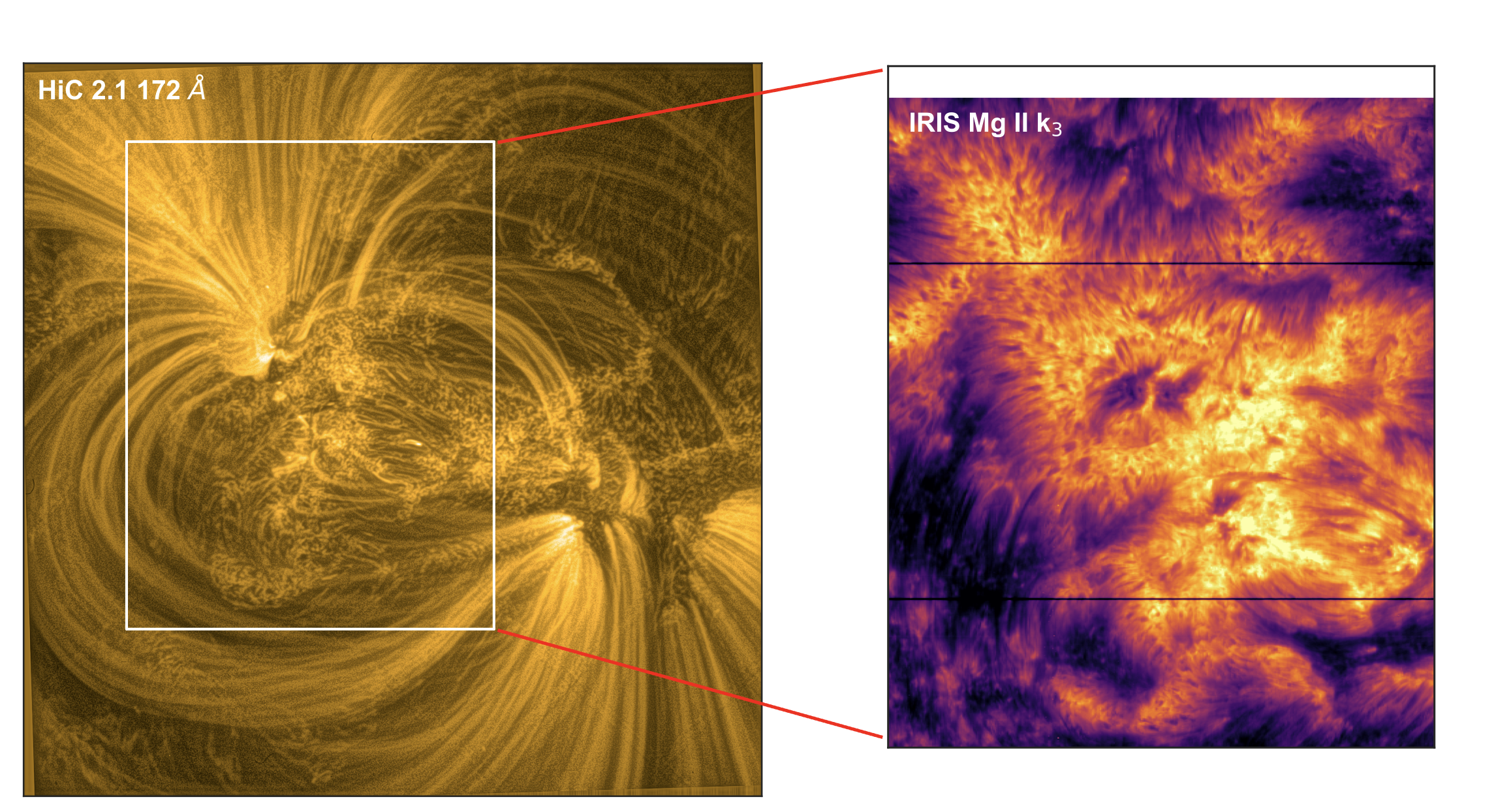
NASA Observations Find What Helps Heat Roots of ‘Moss’ on Sun
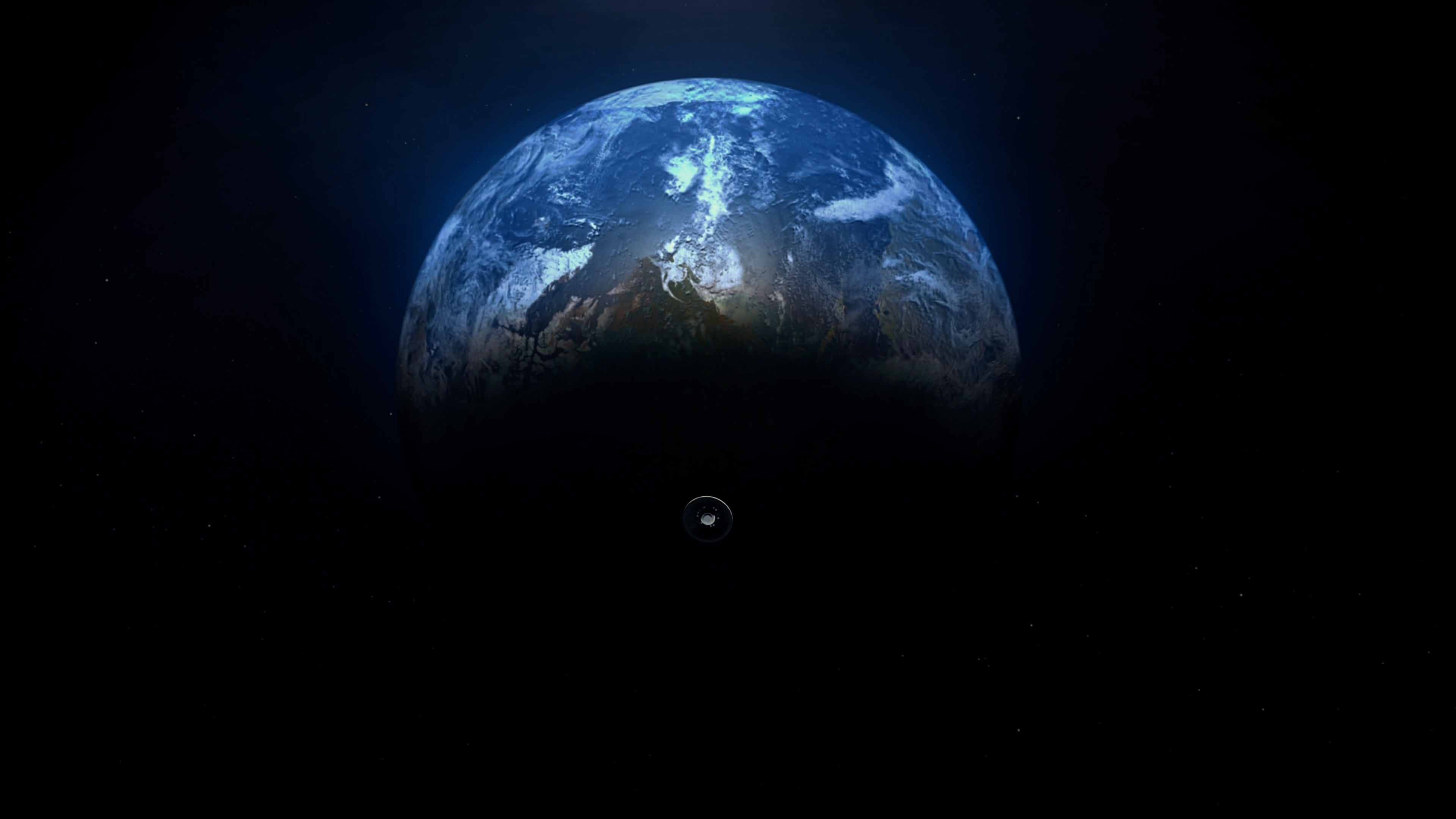
Amendment 9: New Opportunity: C.26 Rapid Mission Design Studies for Mars Sample Return
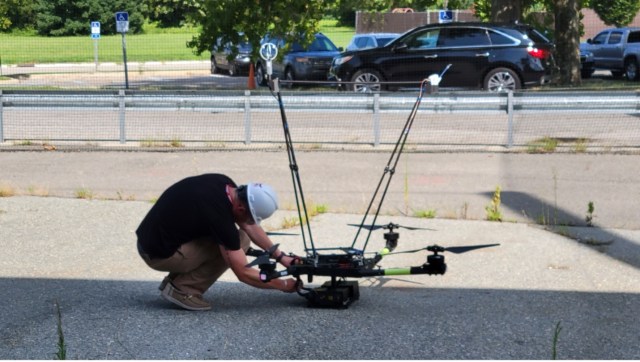
NASA Langley Team to Study Weather During Eclipse Using Uncrewed Vehicles
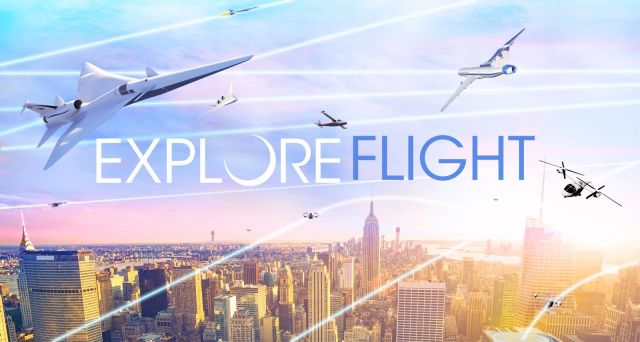
ARMD Solicitations
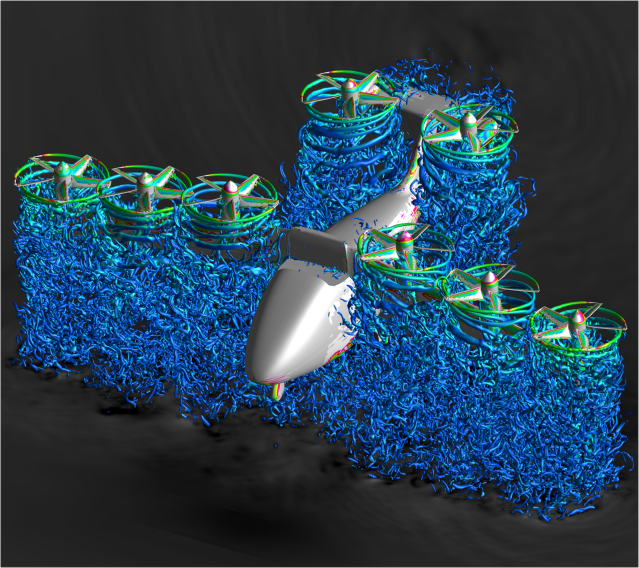
NASA Noise Prediction Tool Supports Users in Air Taxi Industry
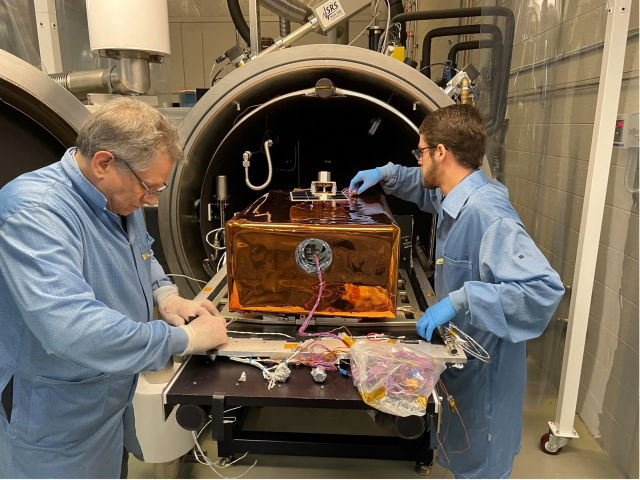
NASA Goddard to Build Quake Detector for Artemis III Moon Landing
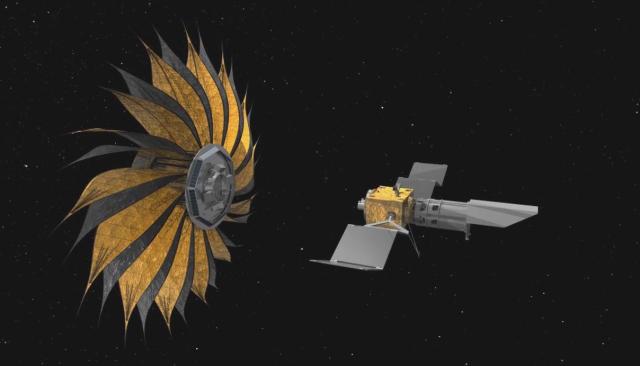
Tech Today: Folding NASA Experience into an Origami Toolkit
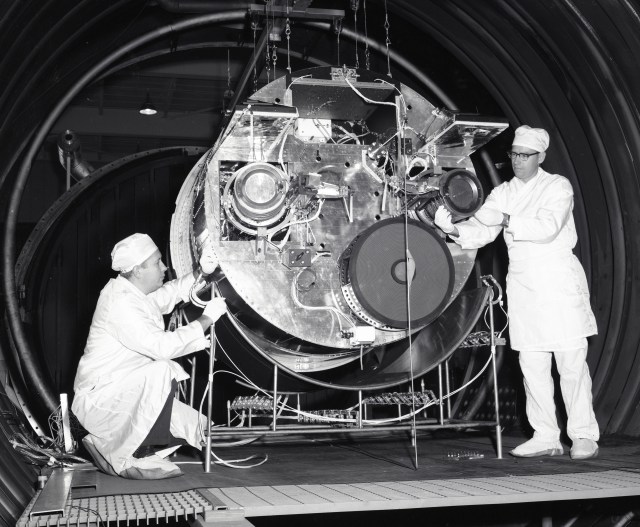
NASA’s SERT II: ‘A Genuine Space Success Story’
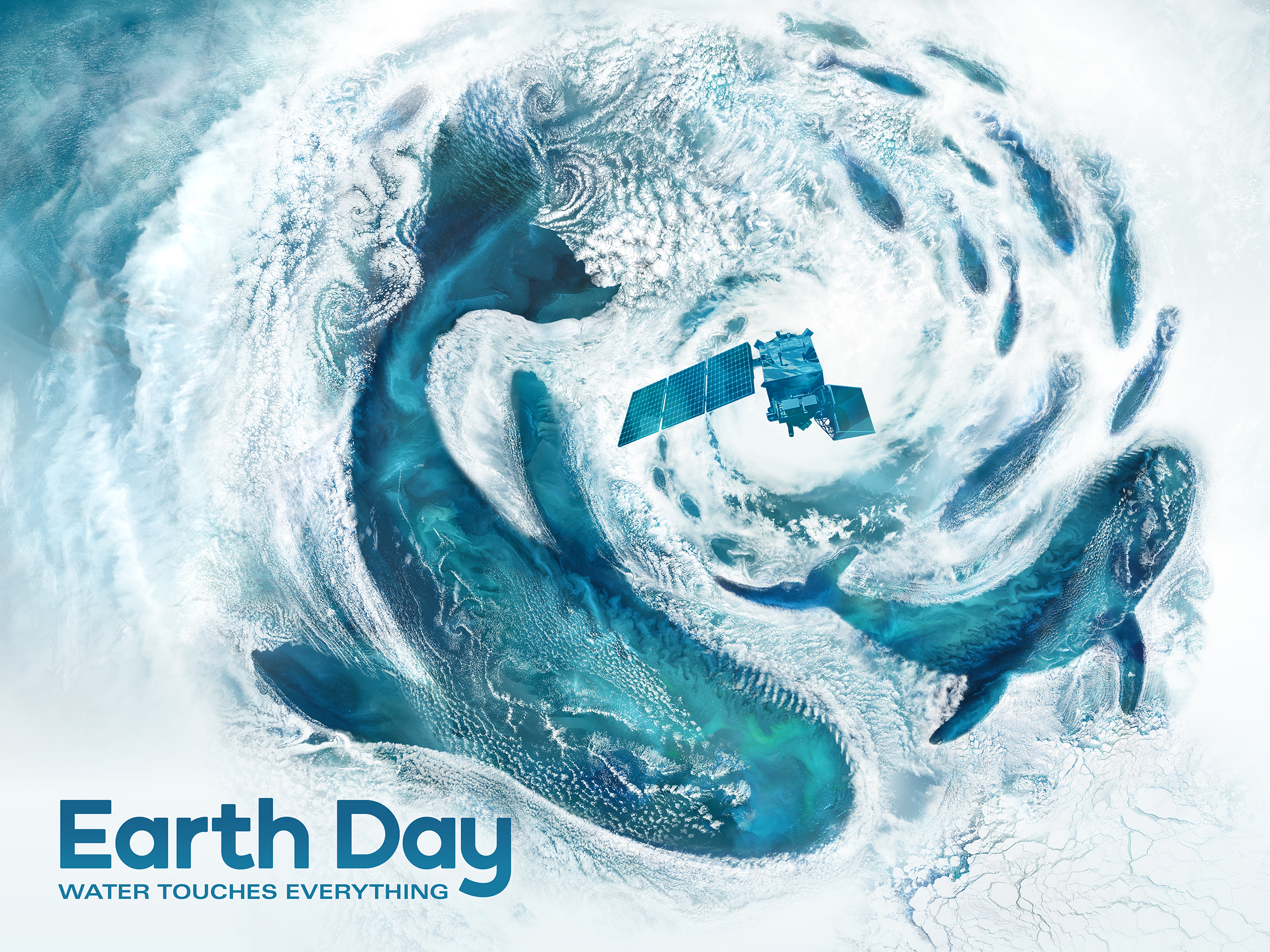
Earth Day 2024: Posters and Virtual Backgrounds
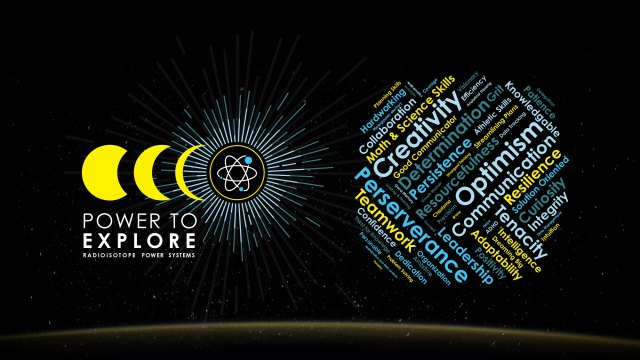
NASA Names Finalists of the Power to Explore Challenge
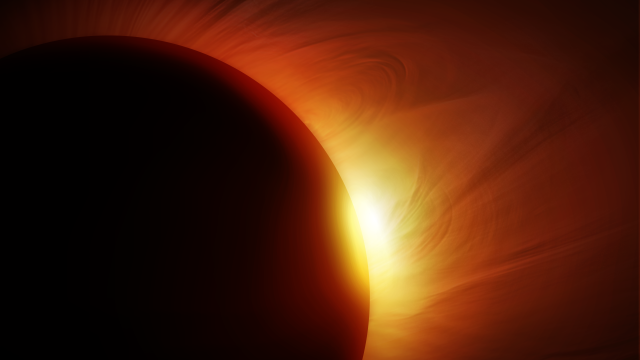
NASA Partnerships Bring 2024 Total Solar Eclipse to Everyone
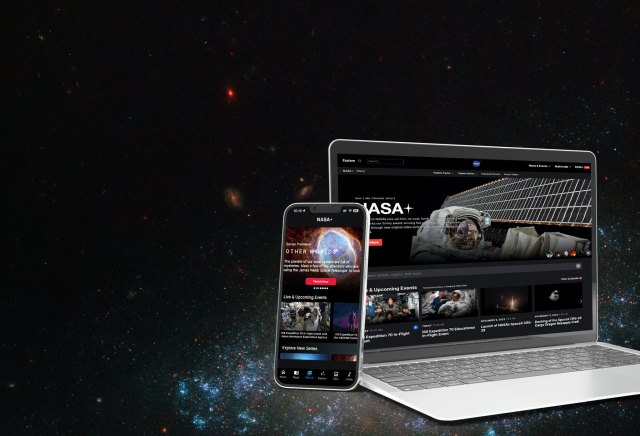
NASA Receives 13 Nominations for the 28th Annual Webby Awards
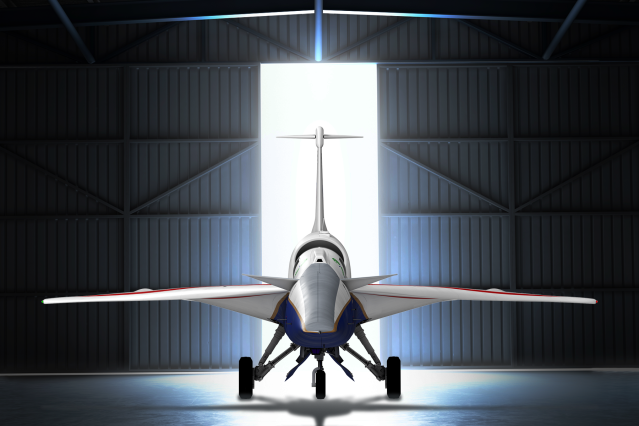
La presentación del X-59 de la NASA personifica la tradición aeronáutica
Nasa gives solar ionic propulsion a monster boost.
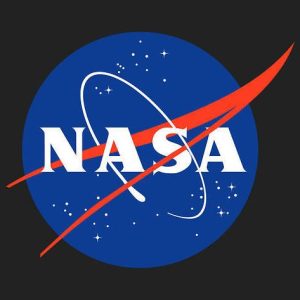
NASA this week took a giant step toward using solar electric power for future space missions by awarding a $67 million to Aerojet Rocketdyne to develop an advanced electric propulsion system.
Such a system would deploy large solar arrays that can be used to convert sunlight into electrical power that ionizes atoms of xenon which is the propellant for the spacecraft’s thrusters. The thrust of such a power plant isn’t huge but its ability to provide increasing, continuous power over a long period of time is what makes it so attractive for long-duration spaceflights.
In addition, such a power plant could potentially increase spaceflight fuel efficiency by 10 times over current chemical propulsion technology and more than double thrust capability compared to current electric propulsion systems, NASA said.
Specifically Aerojet Rocketdyne will develop and deliver an integrated electric propulsion system – known as the Advanced Electric Propulsion System (AEPS) — consisting of a thruster, power processing unit (PPU), low-pressure xenon flow controller, and electrical harness. NASA has developed and tested a prototype thruster and PPU that the company can use as a reference design, the space agency stated.
NASA has long experimented and used different forms of electronic electric propulsion technology. NASA said the first successful ion electric propulsion thruster was developed at Glenn Research Center in the 1950s. The first operational test of an electric propulsion system in space was Glenn’s Space Electric Rocket Test 1, which flew on July 20, 1964. Since then, NASA has increasingly relied on solar electric propulsion for long-duration, deep space robotic science and exploration missions the most recent being NASA’s Dawn mission which surveyed the giant asteroid Vesta and the protoplanet, Ceres, between 2011 and 2015.
NASA has also experimented with ion propulsion technology known as a Hall Thruster which trap electrons in a magnetic field and then use them to ionize propellant, which generate thrust, NASA said.
Interestingly a Hall Thruster was onboard the AirForce’s secretive X-37B spacecraft last year. The Air Force Research Laboratory said at the time: The Hall thruster that will fly on the X-37B experiment is a modified version of the units that have propelled Space and Missile Systems Center’s first three Advanced Extremely High Frequency military communications spacecraft. A Hall thruster is a type of electric propulsion device that produces thrust by ionizing and accelerating a noble gas, usually xenon. While producing comparatively low thrust relative to conventional rocket engines, Hall thrusters provide significantly greater specific impulse, or fuel economy. This results in increased payload carrying capacity and a greater number of on-orbit maneuvers for a spacecraft using Hall thrusters rather than traditional rocket engines. The experiment will include collection of telemetry from the Hall thruster operating in the space environment as well as measurement of the thrust imparted on the vehicle. The resulting data will be used to validate and improve Hall thruster and environmental modeling capabilities, which enhance the ability to extrapolate ground test results to actual on-orbit performance.
The AEPS is the next step in NASA’s overarching Solar Electric Propulsion project, which the space agency says is developing critical technologies to extend the range and capabilities of ambitious new science and exploration missions such as NASA’s proposed Asteroid Redirect Mission (ARM). ARM, if it gets funded, will rendezvous with the target asteroid, land a robotic spacecraft on the surface, grab a 4 meter or so sized boulder and begin a six-year journey to redirect the boulder into orbit around the moon for exploration by astronauts
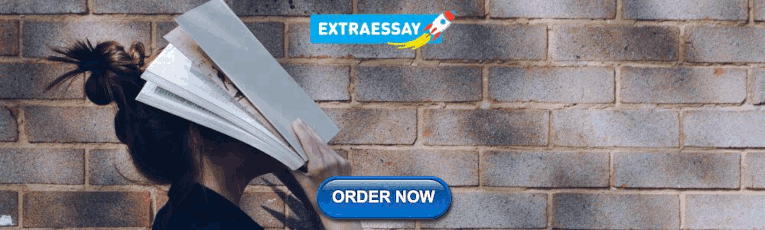
IMAGES
VIDEO
COMMENTS
The research challenges for electric propulsion technologies are examined in the context of s-curve development cycles. It is shown that the need for research is driven both by the application as well as relative maturity of the technology. For flight qualified systems such as moderately-powered Hall thrusters and gridded ion thrusters, there are open questions related to testing fidelity and ...
4. Challenges for Electric Propulsion Development The future of electric propulsion is mainly pushing in two directions: increasing the specific impulse and longevity of high-power technologies and improving the efficiency and reliability of low-power technologies. In the former, thrusters with longer lifespans and greater "fuel economy" will
Future Directions for Electric Propulsion Research Ethan Dale * , Benjamin Jorns and Alec Gallimore Department of Aerospace Engineering, University of Michigan, Ann Arbor , MI 48105, USA;
The research challenges for electric propulsion technologies are examined in the context of s-curve development cycles and it is shown that the need for research is driven both by the application as well as relative maturity of the technology. The research challenges for electric propulsion technologies are examined in the context of s-curve development cycles.
• NASA has been making investments since ~2015 in technologies related to electric aircraft propulsion. These investments span all-electric with our four passenger X-plane and electric vertical lift studies, to regional flight demonstrators and targeted technology maturation programs. These latter two areas are focused ultimately
The research challenges for electric propulsion technologies are examined in the context of s-curve development cycles. It is shown that the need for research is driven both by the application as well as relative maturity of the technology. ... Alec Gallimore. "Future Directions for Electric Propulsion Research." Aerospace (Basel) 7.9 (2020 ...
Electric propulsion is now in both widespread use and active flight-implementation across a broad spectrum of commercial and government applications ranging from cubesats, LEO constellations, GEO comsats, deep space science missions, and even the human-tended Lunar Gateway. It has been my good fortune to witness, and even participate in, to some small extent, the transition of electric ...
The research challenges for electric propulsion technologies are examined in the context of s-curve development cycles. It is shown that the need for research is driven both by the application as well as relative maturity of the technology. For flight qualified systems such as moderately-powered Hall thrusters and gridded ion thrusters, there are open questions related to testing fidelity and ...
Future directions for electric propulsion research. Publication, Journal Article. Dale, E; Jorns, B; Gallimore, A Published in: Aerospace. ... The research challenges for electric propulsion technologies are examined in the context of s-curve development cycles. It is shown that the need for research is driven both by the application as well as ...
My Research and Language Selection My Research Sign into My Research Create My Research Account English; Help and support Help and support. Support Center Find answers to questions about products, access, use, setup, and administration. Contact Us Have a question, idea, or some feedback? We want to hear from you.
MW Class Electric Machine Testing Problem Efficient (>96%), high specific power density (>13 kW/kg) MW electric machines are an enabling technology for electric propulsion. NRA developed concepts which have shown great promise on paper are now being tested. These cutting edge machines, built at the University of
NASA's Glenn Research Center is the lead center for the agency's electric propulsion efforts. Its researchers and engineers are developing and demonstrating high-power electric propulsion systems as well as supporting technologies, including high-voltage power management and distribution and spaceflight diagnostics for measuring system ...
Integrated with electric propulsion systems to support both service and propulsion loads by electricity, All-electric ships (AESs) ... Suggestions on critical issues and future research directions are proposed in Section 5, and the paper concludes in Section 6. 2.
The research challenges for electric propulsion technologies are examined in the context of s-curve development cycles. It is shown that the need for research is driven both by the application as well as relative maturity of the technology. ... Future Directions for Electric Propulsion Research. Ethan Dale, Benjamin Jorns, Alec Gallimore ...
Gale Academic OneFile includes Future Directions for Electric Propulsion Research by Ethan Dale, Benjamin Jorns, and Alec Ga. Click to explore.
The center of attention is an electric machine that can work either as an electric motor to turn propellers or large engine fans, or a generator to produce one million watts of power - enough to power 760 average U.S. households. NASA's collaborators in this research include the University of Illinois at Urbana-Champaign (UIUC) and Collins ...
Promising advances in rocket propulsion. The US Defense Advanced Research Projects Agency (Darpa) has recently commissioned three private companies, Blue Origin, Lockheed Martin and General ...
This Special Issue on "Electrical Propulsion Technology" is open, but not limited, to recent findings in novel and possible future applications of plasmas in electrical propulsion technologies. Papers providing fundamental insights into the understanding of plasmas and detailed analysis of electrical discharges, pushing forward cutting-edge ...
The Field Emission Electric Propulsion (FEEP) technology based on Liquid Metal Ion Sources (LMIS) has been developed for over two decades by FOTEC Forschungs-und Tech-nologietransfer GmbH, the ...
NASA gives solar ionic propulsion a monster boost. NASA. Apr 28, 2016. Article. NASA this week took a giant step toward using solar electric power for future space missions by awarding a $67 million to Aerojet Rocketdyne to develop an advanced electric propulsion system. Such a system would deploy large solar arrays that can be used to convert ...
Electrospray thruster life and mission performance are strongly influenced by grid impingement, the extent of which can be correlated with emission modes that occur at steady-state extraction voltages, and thruster command transients. Most notably, we experimentally observed skewed cone-jet emission during steady-state electrospray thruster operation, which leads to the definition of an ...
Feature papers represent the most advanced research with significant potential for high impact in the field. A Feature Paper should be a substantial original Article that involves several techniques or approaches, provides an outlook for future research directions and describes possible research applications.