- Open access
- Published: 26 November 2022
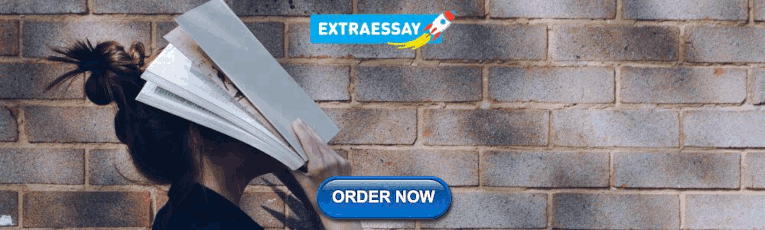
Recent biomedical advancements in graphene oxide- and reduced graphene oxide-based nanocomposite nanocarriers
- Naline Bellier 1 na1 ,
- Phornsawat Baipaywad 1 , 2 na1 ,
- Naeun Ryu 1 ,
- Jae Young Lee ORCID: orcid.org/0000-0003-4493-2494 3 &
- Hansoo Park 1
Biomaterials Research volume 26 , Article number: 65 ( 2022 ) Cite this article
6762 Accesses
34 Citations
1 Altmetric
Metrics details
Recently, nanocarriers, including micelles, polymers, carbon-based materials, liposomes, and other substances, have been developed for efficient delivery of drugs, nucleotides, and biomolecules. This review focuses on graphene oxide (GO) and reduced graphene oxide (rGO) as active components in nanocarriers, because their chemical structures and easy functionalization can be valuable assets for in vitro and in vivo delivery. Herein, we describe the preparation, structure, and functionalization of GO and rGO. Additionally, their important properties to function as nanocarriers are presented, including their molecular interactions with various compounds, near-infrared light adsorption, and biocompatibility. Subsequently, their mechanisms and the most appealing examples of their delivery applications are summarized. Overall, GO- and rGO-based nanocomposites show great promise as multipurpose nanocarriers owing to their various potential applications in drug and gene delivery, phototherapy, bioimaging, biosensing, tissue engineering, and as antibacterial agents.
Introduction
Graphene oxide (GO) is a two-dimensional (2D) nanomaterial comprising single-layer sheets of sp 2 hybridized carbons, sites of sp 3 hybridized carbons, and oxygenated groups, obtained from the oxidation and exfoliation of graphite [ 1 ]. First synthesized by British chemist B.C. Brodie in 1859, GO is obtained by chemical treatment of graphite flakes using strong oxidizers followed by dispersion and exfoliation in acidic mediums, a more refined method of which are commonly used today despite the production of resultant toxic gases [ 2 , 3 , 4 , 5 ]. However, current research in GO synthesis focuses on more cost-effective and eco-friendly development methods because interest in various applications of GO has increased owing to its attractive chemical and physical characteristics.
GO is hydrophilic and highly dispersible in water and polar organic solvents because of its oxygen-containing functionalities, such as hydroxyl, carboxyl, carbonyl, epoxide, phenol, lactone, and quinone groups [ 6 , 7 , 8 ]. Carboxylic groups are located on the edges of GO, whereas epoxide and hydroxyl groups are present on the basal plane of GO [ 9 , 10 , 11 ]. Furthermore, GO exhibits excellent and unique properties, including a 2D planar structure, large surface area, straightforward modification, chemical stability, good biocompatibility, and high mechanical strength [ 8 , 12 , 13 ]. In particular, GO can strongly interact with various small molecules and macromolecules (e.g. drugs, proteins, metals, biomolecules, and cells) via π-π stacking, covalent bonding, hydrophobic interactions, electrostatic forces, and hydrogen bonding [ 6 , 13 , 14 ]. Because of such unique characteristics, GO has great potential in nanomedicine and biomedical applications which are presented in Fig. 1 [ 14 ].
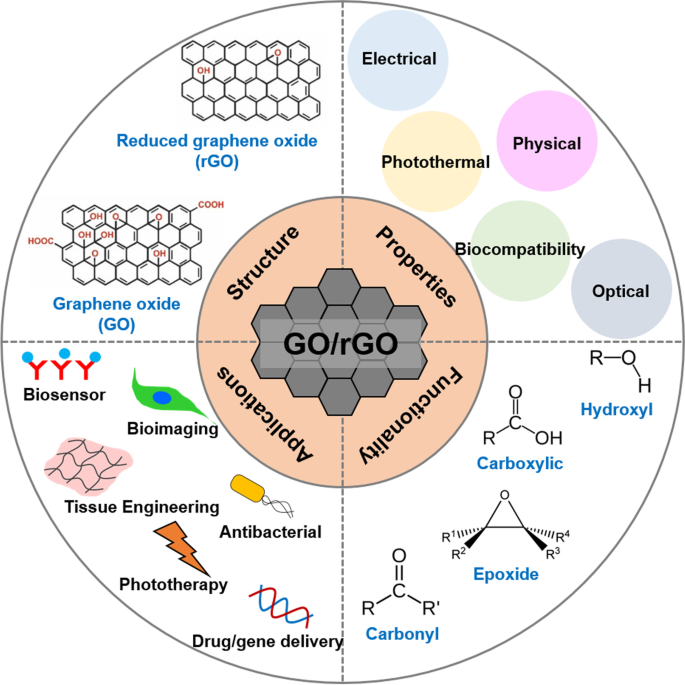
Graphical summary of recent biomedical advancements in graphene oxide- and reduced graphene oxide-based nanocomposite nanocarriers
Chemical reduction of GO is the most widely applied method for preparing reduced GO (rGO) [ 15 ]. Various chemical reductants, such as anhydrous hydrazine [ 16 ], hydrazine monohydrate, L-ascorbic acid, sodium borohydride [ 17 ], hydroquinone [ 18 ], birch [ 19 ], glucose [ 20 ], hydroxylamine [ 21 ], pyrrole [ 22 ], amino acids [ 23 ], strongly alkaline solutions [ 24 ], and urea [ 25 ] have been reported to remove the majority of oxygenated functional groups and partly restore sp 2 carbon bonds in graphene [ 26 , 27 , 28 ]. Chemical reactions increase the conductivity, hydrophobicity, and π-π stacking interactions, which are important for drug delivery applications [ 15 , 28 ]. Typically, hydrophobic anticancer drugs and small molecules can be loaded more efficiently onto rGO surfaces via π-π stacking and hydrophobic interactions compared to GO [ 17 , 29 ]. Additionally, rGO nanosheets have been widely studied for phototherapy owing to their large surface area, high light-adsorption ability, and excellent photothermal effect [ 30 , 31 , 32 ]. Because of these exceptional properties, rGO has been extensively explored as a promising material for multi-purpose nanocarriers.
In addition to the physical and photothermal properties of GO and rGO, which allow for effective cancer treatment via drug and gene delivery and phototherapy, respectively, both materials have been widely explored for bioimaging, biosensing, tissue engineering, and antibacterial applications. This is because of other significant properties of GO/rGO, such as electrical conductivity, light absorbance and emission, and biological effects. Although other methods will be discussed, GO/rGO-based materials have been particularly popular in bioimaging because of their fluorescent emission under the right excitation wavelength [ 33 , 34 ]. Meanwhile, GO-/rGO-based biosensors use their fluorescence quenching abilities [ 35 , 36 ] although high electrical conductivity of rGO makes it a suitable candidate for electrochemical (EC) or electrochemiluminescence (ECL) assays [ 37 , 38 ]. Furthermore, GO/rGO is known to promote stem cell proliferation and differentiation, which has encouraged research in their use in tissue engineering, particularly that of cardiac and nerve tissues, which improves in the presence of a conductive material [ 39 , 40 , 41 ]. Finally, GO/rGO is known to be cytotoxic towards bacteria, which has prompted research in antibacterial applications [ 42 , 43 ].
Several review papers have focused on graphene and GO for biomedical applications; however, the discussion of rGO remains only a footnote in these [ 44 , 45 , 46 , 47 , 48 ] In this review, we provide a brief overview of the history and preparation of GO and rGO as well as their chemical structures, functionalization methods, and properties. Their mechanisms and applications in the form of nanocarriers in drug and gene delivery, phototherapy, bioimaging, biosensing, tissue engineering, and bacterial elimination, along with their potential as multipurpose nanocarriers, are also discussed.
Synthesis and structure of graphene oxide and reduced graphene oxide
British chemist B. C. Brodie first synthesized GO in the nineteenth century (1859) by treating graphite with a mixture of oxidizing agents (potassium chlorate (KClO 3 ) and fuming nitric acid (HNO 3 )) [ 2 ]. After oxidative treatments with four repeated reactions, an increase in the overall mass of the graphite flakes was observed, which was believed to result from the presence of additional carbon, hydrogen, and oxygen atoms in the product [ 49 ]. Another common technique, modified from the Brodie method, was described by Stuadenmaier in 1898. The acidity of the mixture was increased using concentrated sulfuric acid (H 2 SO 4 ) combined with fuming HNO 3 , followed by the addition of chlorate in multiple aliquots of KClO 3 solution throughout the reaction [ 2 , 50 ]. In 1957, chemists Hummers and Offeman developed another oxidation method [ 2 , 3 ], a safer, quicker, and more efficient process where graphite reacts with a mixture of H 2 SO 4 , sodium nitrate, and potassium permanganate [ 51 ]. The difference from previous methods lies in the use of H 2 SO 4 instead of HNO 3 [ 50 ]. Altogether, all the methods mentioned above require extensive oxidation of aromatic structures to weaken the van der Waals interaction between the graphene sheets for their exfoliation into single layers and dispersion in solutions [ 13 ] which can be further aided by sonication [ 52 ]. However, these oxidation procedures generate toxic gases such as nitrogen dioxide, dinitrogen tetroxide, or chlorine dioxide, the latter being explosive [ 2 ].
Recently, GO has also been synthesized using the “bottom-up” method with strong oxidizers. This process is safer, simpler, and more environmentally friendly than the “top-down” method [ 53 ]. For instance, Tang-Lau et al. [ 53 ] used glucose as the sole reagent and the bottom-up assembly technique to grow GO. Moreover, this method has an important advantage because the layer thickness can be controlled by adjusting the growth parameters. An EC alternative was explored by Pei et al. [ 54 ] using electrolytic oxidation by dipping graphite paper in H 2 SO 4 for EC intercalation, followed by exfoliation to obtain GO, which was also conducted via electrolysis. Excess H 2 SO 4 can be fully recycled, thereby presenting an environmentally friendly, efficient, and low-cost method of GO production.
Furthermore, GO can be reduced to acquire rGO. Reduction eliminates the majority of the carbonyl, carboxyl, hydroxyl, and epoxy groups on the GO sheets, as illustrated in Fig. 2 [ 55 , 56 , 57 ]. However, the reduction process cannot produce pristine graphene because of the presence of residual oxygen-functional groups and defects [ 58 ]. Moreover, rGO can be prepared using various methods. The most popular method is chemical reduction, although other methods are also available, including thermal reduction, electrochemical reduction, and photothermal reduction [ 28 , 59 , 60 , 61 , 62 , 63 , 64 ]. The partial reduction of GO can allow the tuning of rGO properties, such as molecular adsorption [ 65 ], electrical conductivity [ 66 , 67 ], and light adsorption [ 68 ], as needed.
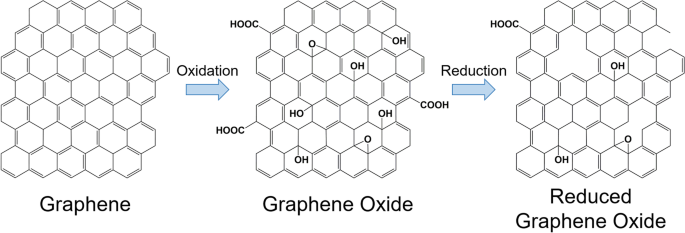
Chemical structures of graphene, graphene oxide, and reduced graphene oxide and their synthetic processes
Chemical reduction is the most popular method for the production of GO-/rGO-based nanocarriers, as it is relatively fast and easy [ 62 , 69 ]. Traditionally, the chemical reduction to prepare rGO involves hydrazine hydrate, which is highly effective. However, because of their high toxicity, many alternatives have been explored, including acids, alkalis, oxygen-containing reducing agents, amino acids, and microorganisms [ 60 , 70 ]. Generally, the reduction requires high temperature (maximum 100 °C), although the reaction time varies depending on the chosen reagent [ 60 , 70 ]. The type of reducing agent critically influences the reduction degree and properties of the prepared rGO [ 71 ].
Dékány’s model is a well-recognized structure for GO comprising two domains, including trans-linked cyclohexyl species interspersed with tertiary alcohols and 1,3-ethers, alongside a corrugated network of keto/quinoidal species [ 1 , 49 ]. The model suggests that the corrugating nature of the carbon network is interrupted by the trans-linked cyclohexyl regions and functionalized by tertiary alcohols and 1,3-ethers [ 49 ]. Different models of GO illustrate the variations in the degree of oxidation, structures, and properties depending on the starting materials (graphite source) and oxidation protocol [ 49 ]. Furthermore, all the GO structural models contain oxygen groups at the edges of the graphene sheets and above and below the basal plane [ 49 , 72 ].
Moreover, rGO remains structurally similar to GO, with only the elimination of most oxygen-containing functional groups and an increase in the percentage of sp 2 hybridization being the main differences [ 57 ]. The elimination of oxygen-containing functional groups creates vacancies in the GO sheet structure, which is evident from the increase in the ratio of the D to G peak intensity in the Raman spectrum [ 57 , 73 ]. Second-order Raman scattering is represented by the 2D band where its intensity, width, and position relates to the stacking of GO and rGO sheets [ 74 , 75 ]. Finally, sp 3 -hybridisation is dependent on the relative intensity of the D band compared to that of the G band [ 76 ]. It should be noted that rGO is less susceptible to photodegradation than GO because it contains fewer oxygen-containing functional groups [ 72 ].
Properties of graphene oxide and reduced graphene oxide
Physical properties.
Initially, GO attracted interest in the nanocarrier field because of its good colloidal stability and large surface area. The 2D structure of GO lends itself to a large surface area, which results in a high loading capacity, which is a property shared by rGO [ 77 ]. However, unlike GO, rGO exhibits poor colloidal stability and readily aggregates within a few hours of dispersion in water [ 78 ]. The percentage of C-O and C = O bonds in rGO affects its colloidal stability. The better hydrophilicity of GO is attributed to the presence of abundant oxygen-containing functional groups in its structure compared to that of rGO [ 78 , 79 ]. Nevertheless, rGO with improved colloidal stability can be produced depending on the reducing agents and resulting surface properties [ 80 ]. Additionally, graphene-derived materials are known to have high mechanical strength and flexibility; monolayer GO and rGO have an effective elastic modulus of approximately 207.6 [ 81 ] and 250 GPa [ 82 ] respectively. Finally, rGO was shown to have more thermal stability due to its comparatively less deoxygenated state [ 83 ].
Electrical properties
With the possibility of counteracting its colloidal instability, rGO has attracted interest in the nanocarrier field owing to its high electrical conductivity. In addition, GO is considered an insulator because of its large defects in sp 2 carbon bonds, whereas rGO can display high electrical conductance resembling that of pristine graphene [ 79 ]. The change from an insulator to a highly conductive material has been ascribed to the reduction in oxygen functional groups and the high percentage of sp 2 hybridization [ 68 ]. An increase in the C/O ratio increased the conductivity, allowing the rGO conductivity to be tuned [ 66 ]. Furthermore, GO displays a negative differential resistance with varying results depending on the relative humidity, air pressure, and applied voltage [ 84 ].
Optical properties
Both GO and rGO benefit from the absorbance of visible and ultraviolet light, with an observed emission wavelength in the range of 350–650 nm [ 85 ]. The absorbance peaks of GO and rGO are approximately 230 [ 85 , 86 ] and 260 nm, respectively [ 87 , 88 ]; however, both have a wide absorbance in the range of 200–900 nm [ 85 , 87 , 88 ]. Depending on the excitation wavelength, a range of fluorescent emissions can be achieved [ 89 ]. Furthermore, the GO and rGO emission peaks can be further tuned based on the number and type of attached functional groups [ 89 , 90 ].
Photothermal properties
Both GO and rGO effectively absorb near-infrared (NIR) light, which is a biocompatible light source that penetrates tissues. Moreover, GO and rGO convert the absorbed NIR light energy to heat, increasing the temperature in GO and rGO and their surrounding media [ 85 , 88 , 91 , 92 ]. While both GO and rGO can absorb NIR, rGO is more effective [ 91 ] likely because of the red shift in the absorbance peak from approximately 230 to 260 nm [ 87 , 88 ].
Biocompatibility
Opinions on the cytotoxicity and biocompatibility of GO are contradictory because of the varying effects depending on the concentration used; specifically, GO is cytotoxic at higher concentrations. However, GO generally has low cytotoxicity at concentrations below 4 μg/mL [ 93 , 94 ]. Moreover, rGO is less cytotoxic than GO even at higher concentrations [ 94 , 95 ]. This cytotoxicity could be attributed to membrane damage caused by the sharp edges of the nanoparticles and induced oxidative stress [ 96 ]. Research has indicated that cytotoxicity of GO is also dependent on the particle size and level of aggregation [ 97 ]. Meanwhile, high carbon radical density has been associated with the increased toxicity of GO via lipid peroxidation and membrane damage [ 98 ]. Therefore, the level of cytotoxicity can be controlled by tuning all these factors. Genotoxicity of GO/rGO nanoparticles is also a concern, with research indicating that both direct and indirect mechanisms exist in DNA damage [ 99 ]. Although the surface functionalization of GO affects its eventual clearance, GO particles aggregate in organs, potentially causing structural damage [ 100 , 101 ]. Induced by GO, platelet aggregation causing thromboembolism is also a concern, although rGO causes significantly less platelet aggregation [ 102 ]. In vivo studies in mice [ 103 ] and fish [ 104 ] resulted in toxic effects, demonstrating that further studies on GO/rGO biocompatibility are needed. Notably, GO/rGO could stimulate the immune response by inducing cellular activation and cytokine production [ 105 ].
In addition, both GO and rGO can displayed antibacterial properties that may be attributed to the previously mentioned membrane damage and oxidative stress as the particles can aggregate on bacterial cells. The degree of such antibacterial effects depends on the oxidative capacity, size [ 96 ], concentration, and contact time of the GO or rGO particles with the bacteria [ 106 ]. A comparison between the cytotoxicity of GO/rGO sheets against bacterial and mammalian cells has been performed, proving that they are more cytotoxic to bacteria at similar concentrations [ 107 ]. However, the relative size ratios between the sheets and cells used in the study were not mentioned. Another study showed that a positive zeta potential of approximately 20 ± 2 mV was particularly effective in capturing gram-negative pathogens, such as E. coli, while being ineffective for gram-positive pathogens, such as S. Aureus [ 43 ]. Notably, research regarding the antibacterial properties of GO/rGO generally uses significantly higher concentrations than the 4 μg/mL, which is regarded as the maximum non-cytotoxic concentration [ 43 , 108 ].
Both GO and rGO have strong interactions with single-stranded DNA (ssDNA) through hydrophobic and π-π stacking interactions [ 109 , 110 , 111 ]. However, functionalization with positively charged molecules is necessary for interactions with double-stranded DNA (dsDNA) to allow electrostatic interactions [ 112 , 113 , 114 ]. Finally, the biodegradation of GO occurs under both aqueous [ 115 , 116 ] and enzymatic conditions [ 117 , 118 , 119 , 120 ]. Enzymatic conditions, including eosinophil peroxidase [ 117 ], myeloperoxidase [ 119 ], and lignin peroxidase [ 120 ] accelerate the process through enzymatic digestion. The effects of GO biodegradation can be observed within hours of exposure to enzymes [ 117 , 119 , 120 ]. Additionally, rGO is affected by enzymatic degradation, although at a slower rate, which might be due to its reduced level of oxidization [ 120 ]. Research indicates that GO degradation is mediated by neutrophils and macrophages, and that the resulting degradation products are neither cytotoxic nor genotoxic [ 119 ].
Functionalizing graphene oxide and reduced graphene oxide
Solubility, biocompatibility, drug-loading capacity, and release efficiency are considered to enhance the functionality and reduce toxicity of graphene-based nanocarriers [ 121 , 122 ]. Recently, the surface functionalization of GO and rGO has been studied to improve their biological properties and enhance their potential efficiency for therapeutic use [ 123 ]. There are two main approaches for modifying the GO or rGO surfaces. First, covalent functionalization is typically carried out using chemical reactions with carboxylic, epoxy, and hydroxyl groups present on the GO surfaces using various coupling agents [ 124 ]. Second, noncovalent functionalization is usually carried out with inorganic nanoparticles and other molecules, such as polymers, drugs, proteins, and small molecules, on the GO or rGO surface through hydrophobic, van der Waals, electrostatic, and H-bonding interactions [ 121 ].
Covalent functionalization
Covalent functionalization is an approach for grafting polymers or immobilizing biomolecules onto GO sheets, based on different chemically reactive functionalities on the basal plane (epoxy and hydroxyl) and sheet edges (carboxylic acid) [ 123 , 125 ]. The surface modifications with stable covalent bonds improve the stability of immobilized proteins, enzymes, drugs, or small molecules in the system to improve GO properties, such as biocompatibility and loading stability [ 126 ]. A few studies have been conducted on the biocompatibility of functionalized GO for the delivery of a series of drugs, including synthetic compounds, proteins, antibodies, and genes, through covalent functionalization. In recent years, the application of GO as a carrier for small interfering RNA (siRNAs) has demonstrated great potential.
Wang et al. [ 127 ] prepared octaarginine (R8) and anti-HER2 antibody-functionalized GO using covalent conjugation (Fig. 3 ) as a novel gene delivery system for tumor therapy. In addition, R8 was modified onto GO surfaces as a cell-penetrating peptide to enhance the effect of siRNA delivery, whereas anti-HER2 was labeled together to bind with HER2. Furthermore, GO-R8/anti-HER2/survivin-siRNA is a potentially efficient gene-silencing carrier for siRNA delivery in cancer therapy in vitro and in vivo.
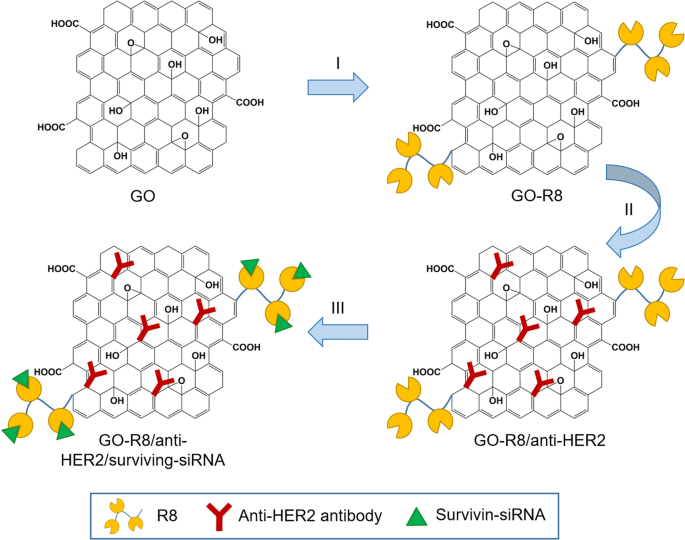
Schematic illustration of graphene oxide (GO) functionalization with octaarginine (R8) and anti-HER2 antibody. Notes: Adapted from Wang X, Sun Q, Cui C, Li J, Wang Y. Anti-HER2 functionalized graphene oxide as a survivin-siRNA delivery carrier inhibits breast carcinoma growth in vitro and in vivo. Drug Des Devel Ther. 2018;12:2841–2855 [ 127 ]
In a study by Li et al. [ 128 ] a novel nanogene delivery system into HeLa cells was prepared by functionalizing GO with R8 and cRGDfV peptides, which could increase the stability, electropositivity, transfection efficiency, cytocompatibility, and tumor inhibition [ 128 ]. In addition, Jana et al. [ 123 ] successfully achieved dual covalent chemical functionalization of GO with tris-[nitrilotris(acetic acid)] and biotin. This functionalized GO served as a carrier for cellular delivery of oligohistidine- and biotin-tagged biomolecules such as proteins.
Functionalization of GO with polymers can improve the drug release efficiency at tumor sites when the modified carriers reach the target cells, resulting in more effective therapy. For example, Gao et al. [ 129 ] developed a GO-modified polysebacic anhydride (GO/PSA) composite as a drug carrier to improve controlled release properties. GO/PSA composites were synthesized via Steglich esterification, which occurred between PSA and the suspended hydroxyls in GO to yield esters. The GO to PSA ratio affected the drug release duration, and the composites at the optimal ratio exhibited a long-term release of up to 80 days. The effective drug release rate exceeded 95%.
Similarly, de Sousa et al. [ 122 ] produced nanocarriers consisting of GO functionalized with folic acid (FA) for drug delivery (Fig. 4 ). In this system, FA was linked to polyethylene glycol (PEG) and coupled to the GO surface. The dynamic release of drugs from the nanocarrier was examined under two physiological conditions using sink conditions and camptothecin (CPT) as a model drug. Toxicity screening of the nanocarrier was performed in vitro for two tumor cell models that promoted tumor cell death by apoptosis.
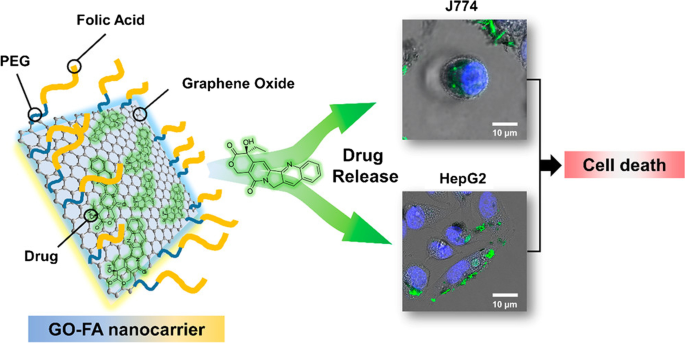
Schematic of graphene oxide (GO) functionalization with folic acid (FA)-linked polyethylene glycol (PEG) and preclinical screening tests for two tumor cell models. Notes: Reprinted with permission from de Sousa, Visani de Luna, Fonseca, Giorgio, and Alves. Folic-acid-functionalized graphene oxide nanocarriers: Synthetic approaches, characterization, drug delivery study, and antitumor screening. ACS Appl Nano Mater. 2018;1(2):922–932. [ 122 ] Copyright 2018, American Chemical Society
Bao et al. [ 126 ] reported the use of a facile amidation process to synthesize the GO covalently functionalized with chitosan (CS) for drug and gene delivery (Fig. 5 ). Grafting CS onto GO sheets improves the solubility and biocompatibility of GO. Moreover, inorganic nanoparticles, such as iron oxide, have been conjugated to the GO surface to enhance T 2 -weighted magnetic resonance (MR) imaging contrasts.
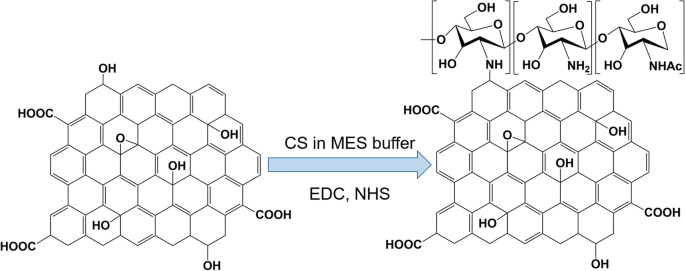
Synthesis of chitosan (CS)-functionalized graphene oxide. MES buffer is 2-(N-morpholino)ethanesulfonic acid buffer, EDC is 1-Ethyl-3-(3-dimethylaminopropyl)carbodiimide, and NHS is N-Hydroxysuccinimide. Note: Adapted from Bao H, Pan Y, Ping Y, et al. Chitosan-functionalized Graphene Oxide as a nanocarrier for drug and gene delivery. Small. 2011;7(11):1569–1578 [ 126 ]
Ma et al. [ 130 ] reported a multifunctional superparamagnetic GO-iron oxide hybrid nanocomposite (IONP) that was further functionalized with biocompatible PEG, which displayed increased drug loading capacity and strong T 2 -weighted MR contrast in a mouse tumor and liver. Specifically, GO-IONP-PEG was synthesized by the chemical deposition of IONPs onto GO sheets and the subsequent functionalization of GO with branched PEG through amide bonds, as illustrated in Fig. 6 . However, covalent functionalization is not popular for immobilizing biomolecules onto rGO surfaces because of the lack of oxygen-containing functional groups on the surface of rGO.
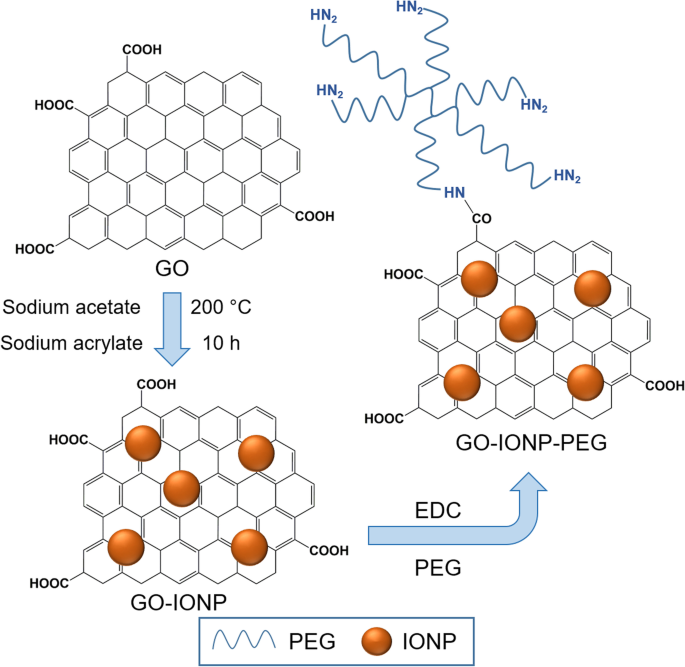
Schematic illustration of synthesis of graphene oxide (GO) and iron oxide (IONP) nanocomposite functionalized with polyethylene glycol (PEG). Notes: Adapted from Ma X, Tao H, Yang K et al. Functionalized graphene oxide-iron oxide nanocomposites for magnetically targeted drug delivery, photothermal therapy, and magnetic resonance imaging. Nano Res. 2012;5(3):199–212 [ 130 ]
Noncovalent functionalization
In general, the noncovalent functionalization of GO and rGO involves van der Waals forces, π-π interactions, hydrogen bonding, and electrostatic interactions with polymers or biomolecules [ 131 ]. Noncovalent interaction is a simple approach for functionalization with various molecules without impairing the internal structure and affecting important properties, such as electrical conductivity and mechanical strength, of GO or rGO after functionalization with other materials [ 132 ].
An example of functionalization with inorganic nanoparticles as carriers for anticancer applications in the form of silver (Ag) nanoparticles was reported by Kavinkumar et al. [ 133 ]. The GO/rGO-Ag nanoparticle composites were obtained by a chemical route using vitamin C as the reducing agent (Fig. 7 ), demonstrating significant cytotoxicity toward A549 cells. Therefore, this approach has been suggested for cancer prevention and treatment. Usually, noncovalent functionalization of the GO surface with polymers, biomolecules, and drugs can be achieved by either wrapping or absorption, mostly via π-π interactions. The most popular biocompatible polymer used to modify the GO surface is PEG, as it can be easily connected with various anticancer drugs and has continuous release behaviors.
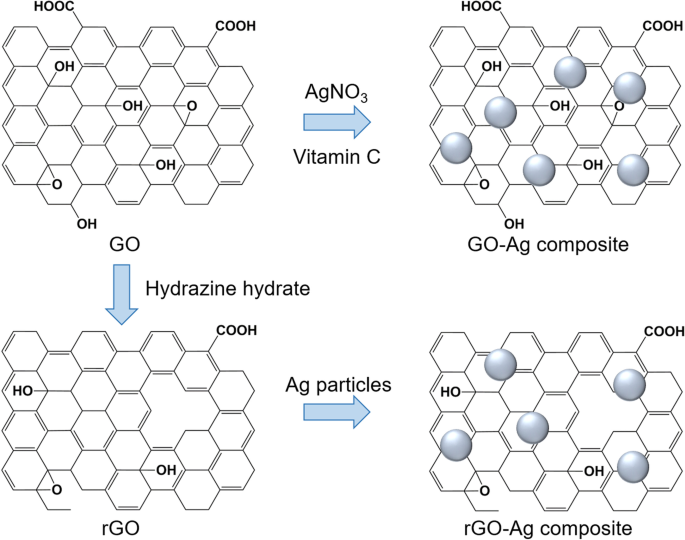
Schematic illustration of synthesis of graphene oxide (GO)/reduced graphene oxide (rGO) and silver (Ag) composite. Notes: Adapted from Kavinkumar T, Varunkumar K, Ravikumar V, Manivannan S. Anticancer activity of graphene oxide-reduced graphene oxide-silver nanoparticle composites. J Colloid Interface Sci. 2017;505:1125–1133 [ 133 ]
Kazempour et al. [ 124 ] studied the release profile of doxorubicin (DOX) at two different pH levels from a biocompatible carrier of PEG-functionalized GO (GO-PEG). They found that the GO-PEG hybrid exhibited high drug loading and more release at acidic pH (5.8) because of two kinds of possible H-bonding between the drug and carrier, whereas at neutral pH (7.4), four kinds of H-bonding existed between the drug and carrier; hence, negligible release occurred.
Several studies have reported a new class of GO-based carriers that use a layer-by-layer (LbL) technique involving the alternate deposition of oppositely charged polyelectrolytes on GO sheets via electrostatic interactions for surface functionalization. For example, Xie et al. [ 134 ] chose two natural linear polymers (positively charged CS and negatively charged dextran) as oppositely charged polyelectrolytes to prepare polyelectrolyte-stabilized GO nanocomposites for drug delivery (Fig. 8 ). Li et al. [ 135 ] used an LbL assembly to synthesize GO nanoassemblies with different types of polyelectrolytes, including poly-L-lysine (PLL), polystyrene sulfonate, PLL-PEG, poly(lactic-co-glycolic acid)-PEG, and DNA oligonucleotides.
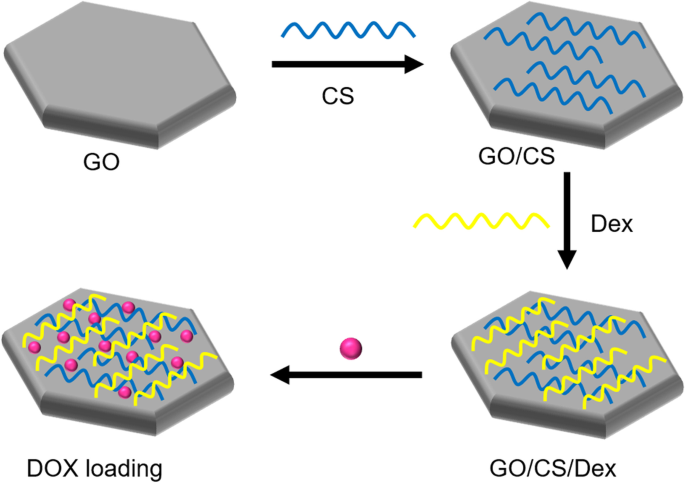
Schematic illustration of synthesis of graphene oxide (GO) nanosheets functionalized with chitosan (CS) and dextran (Dex) and loaded with doxorubicin hydrochloride (DOX). Notes: Adapted from Xie M, Lei H, Zhang Y, et al. Noncovalent modification of graphene oxide nanocomposites with chitosan/dextran and its application in drug delivery. RSC Adv. 2016;6(11):9328–9337 [ 134 ]
Drugs and other biomolecules can be functionalized onto GO and rGO surfaces via noncovalent conjugation. Functionalization of GO nanocolloids with bovine serum albumin protein was reported by Sima [ 121 ] for antitumor drug delivery to melanoma cells. This type of functional bioplatform presents high potential as a miniaturized high-throughput platform for drug screening and testing cancer cell responses to different drugs and drug doses in precision medicine applications. Tan et al. [ 125 ] synthesized immobilized glutaryl-7-aminocephalosporanic acid acylase onto GO as a carrier to enhance the stability of the immobilized enzyme as a catalyst. Mu et al. [ 136 ] elucidated cellular uptake mechanisms by investigating the cellular uptake of protein-coated GO nanosheets. These findings provide fundamental information that sheet-shaped GO nanostructures with protein coatings can adhere to cell surfaces and undergo size-dependent internalization, facilitating nanomedicine and nanotoxicity studies.
A challenging issue for loading hydrophobic drugs onto graphene-based nanocarriers has been addressed for advanced drug delivery systems. Hashemi et al. [ 137 ] suggested paclitaxel (PAC) drug loading on R9 peptide-rGO through hydrophobic interactions (Fig. 9 ) as a green and simple method of achieving an applicable graphene-based drug delivery system to improve the transportation of hydrophobic anticancer drugs. Moreover, a few studies have reported conjugation of DOX, as a model drug, on the rGO surface via strong π-π stacking interactions [ 138 , 139 ] in drug delivery systems. In addition to DOX, different chemotherapeutic drugs, such as CPT [ 140 , 141 ], PAC [ 137 , 142 ], and mitoxantrone [ 143 ] could be loaded onto rGO by hydrophobic and π-π stacking interactions to inhibit the growth rates of cancer cells.
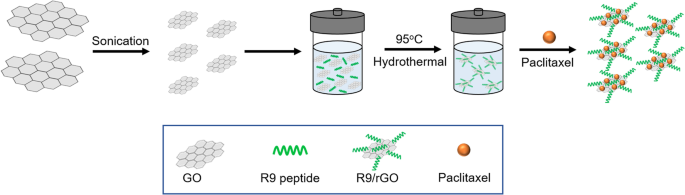
Schematic illustration of reduced graphene oxide (rGO)-based nanocarrier grafted with R9 peptide and loaded with Paclitaxel. Notes: Adapted from: Hashemi M, Yadegari A, Yazdanpanah G, Jabbehdari S, Omidi M, Tayebi L. Functionalized R9–reduced graphene oxide as an efficient nano-carrier for hydrophobic drug delivery. RSC Adv. 2016;6(78):74,072–74,084 [ 137 ]
Applications of graphene oxide- and reduced graphene oxide-based composites
The biomedical applications of GO-/rGO-based composites can be classified into six groups: drug and gene delivery, phototherapy, bioimaging, biosensing, tissue engineering, and antibacterial applications. Each application uses one or several properties of GO and rGO as presented in Fig. 10 , whereas some properties are useful for multiple applications. Thus, GO- and rGO-based nanocarriers have high potential to function as multipurpose carriers that can be applied in any combination of the listed applications. This function is particularly attractive because it can reduce the number of steps required in diagnosis/treatment, creating a more efficient and streamlined process.
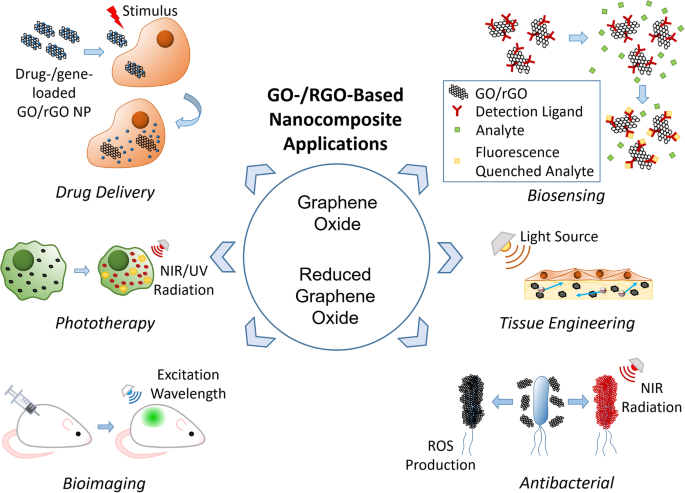
Schematic illustrations of biomedical applications of graphene oxide-/reduce graphene oxide-based nanocarriers
Drug and gene delivery
As described earlier, GO has been explored as a drug and gene carrier owing to its colloidal stability, relatively low toxicity, large surface area, and high loading stability. As rGO shares the same traits with GO except for its colloidal stability, it has also been explored as a carrier; however, its instability issue can be easily rectified with correct functionalization [ 114 , 144 ]. Additionally, functionalization can be used to improve carrier cell targeting abilities [ 33 , 145 , 146 ]. Furthermore, both GO and rGO nanocarriers are pH-responsive [ 33 , 145 , 147 , 148 ] and photo-responsive [ 114 , 141 , 146 , 149 ], allowing for controlled/smart drug release. Drug and gene delivery systems using GO/rGO and their properties are listed in Table 1 .
Vinothini et al. [ 141 ] created an rGO nanocarrier decorated with magnetic nanoparticles and CPT, which was also cross-linked with 4-hydroxycoumarin (HC) using allylamine to explore the rate of release of CPT and 4-HC under various pH conditions. The rate of release increased with lowering of pH. Liu et al. [ 153 ] created a DOX-loaded mesoporous silica-coated rGO composite to release DOX under acidic conditions of pH 5.0 with the addition of NIR irradiation at 808 nm. NIR irradiation significantly increased the release rate of DOX, resulting in a highly effective nanocarrier for controlled drug release. For gene delivery, Assali et al. [ 166 ] designed a cationic GO-based nanocarrier which carried miRNA-101 which suppressed Stathmin1 protein in cancer cells, thereby inducing apoptosis and downregulating autophagy. Furthermore, the particles were covalently decorated with PEG and poly-L-arginine to increase internalization and cause reduction at the surface of the GO nanocarriers, finally increasing their NIR absorption, and making them suitable for phototherapy.
Phototherapy
Phototherapy generally involves two forms: photodynamic and photothermal therapy, both of which use the light-absorbent properties of GO and rGO. Photodynamic therapy relies on a light source to induce singlet oxygen radical generation [ 141 , 167 ], and photothermal therapy relies on NIR as an energy source for heat emission [ 153 , 168 ]. Among the examples listed in Table 2 , a clear preference for using rGO for photothermal therapy exists, likely because of its higher NIR absorbance, making it more effective in treatment. Phototherapy is likely to be used in conjunction with other therapies, specifically drug delivery, for effective cancer treatment.
Gulzar et al. [ 167 ] used both photodynamic and photothermal therapies against cancer cells by conjugating Chlorin e6 to upconversion nanoparticles that were then conjugated to GO. Singlet oxygen was generated alongside an increase in temperature under 808 nm irradiation which was successfully used in in vivo tumor treatment. The resulting upconversion luminescence was also used for imaging, making the particles a useful theranostic tool. Zhang et al. [ 170 ] followed the same strategy of using rGO nanosheets loaded with a PEG-modified Ru(II) complex (PEG-Ru) to target lysosomes in cancer cells for photodynamic and photothermal therapies, which were accomplished by applying 450 nm and 808 nm irradiation, respectively. Thermal-responsive release of the photosensitizer and the imaging agent PEG-Ru was also achieved.
Bioimaging generally uses natural fluorescence emission of GO and rGO, both in vivo and in vitro, for optical imaging, because both emit intense fluorescence at the appropriate excitation wavelength as shown in Table 3 [ 33 , 34 ]. However, they have also been used for carrying contrast agents [ 175 ] for photoacoustic imaging [ 173 , 176 ], MR imaging [ 177 , 178 ], and single-photon emission computed tomography (SPECT) [ 177 ]. Additionally, GO has been used for Raman imaging [ 179 ]. While most research centers on cellular imaging, some groups have used GO for subcellular imaging of organelle-targeted cancer therapy [ 170 , 171 ]. By tracking the GO/rGO nanoparticles, investigation of drug activation pathways involving cellular and organelle interactions can be achieved.
Yogesh et al. [ 181 ] employed pure GO by incubating the cells with nanoparticles for 6 and 24 h and testing the fluorescence at two wavelengths, 405 and 488 nm, resulting in blue luminescence near the nuclear membrane and green luminescence at the 24-h mark. Mosaiab et al. [ 161 ] created a dual-responsive fluorescent GO nanoparticle that reacted to temperature and pH, where boron-dipyrromethene acted as the fluorescent dye, dimethylacetamide acted as the pH-responsive element, and N-isopropylacrylamide acted as the thermoresponsive element. The results indicated that a particle displayed fluorescence under lower pH and temperature (25 °C) and negligible fluorescence under physiological pH and temperature (37 °C) when excited with ultraviolet light at 365 nm. Qian et al. [ 177 ] designed a unique rGO-based nanoparticle capable of multimodal imaging combined with radioisotope therapy and chemotherapy for cancer theranostics. Manganese ferrite was grown in situ on the surface of rGO nanosheets and then functionalized with PEG. The resulting particle proved to be a good MR contrast agent, showing T1 and T2 weighted images. By labeling the nanocomposite with radionuclides 125/131 I, SPECT was achieved alongside radioisotope therapy in conjunction with DOX loading for chemotherapy.
Biosensors containing either GO or rGO generally exhibit fluorescence and fluorescence-quenching properties [ 35 , 36 ]. However, high conductivity of rGO makes it useful for EC or ECL assays [ 37 , 38 ]. Moreover, GO is often used in nucleotide detection owing to its strong interactions with ssDNA, allowing for detection of specific sequences [ 36 , 182 ]. Although several applications involving GO/rGO in biosensing exist, those that uses of them as nanocarriers are limited [ 183 , 184 ]. Applications of GO and rGO in which they were used in biosensors in the form of nanocarriers are listed in Table 4 .
Xia et al. [ 36 ] detected single nucleotide polymorphisms (SNPs) by embedding them in SYBR Green I (SG) before adding GO particles. Subsequently, fluorescence from SG in unstable SNPs was highly quenched by GO within 3 min, whereas fluorescence from perfectly complementary dsDNA was comparatively high. Yuan et al. [ 37 ] designed a pseudobienzyme aptasensor with polyamidoamine-rGO as a nanocarrier conjugated with a hemin/G-quadruplex as nicotinamide adenine dinucleotide oxidase and horseradish peroxidase-mimicking DNA enzyme to detect thrombin. Cyclic voltammetry and differential pulse voltammetry revealed that the particle was capable of highly sensitive and selective detection of thrombin.
Tissue engineering
Both GO and rGO encourage stem cell proliferation and differentiation while functioning as scaffolds or parts of a scaffold, making them ideal for tissue engineering and regeneration, the various applications of which are listed in Table 5 . While GO and rGO nanoparticles can be incorporated into scaffolds for general tissue engineering, such as skin [ 188 , 189 , 190 ], cartilage [ 191 , 192 ], bone [ 189 , 193 , 194 , 195 ], and muscle tissue [ 196 , 197 ] rGO is commonly incorporated into nanofibers to enhance their electroconductivity, which is a significant factor in cardiac and nerve tissue regeneration [ 39 , 40 , 41 ]. Tissue engineering applications can also implement GO/rGO for morphological [ 198 ] and photoelectric [ 199 ] stimulations to encourage cell proliferation/differentiation.
Wang et al. [ 204 ] incorporated polyethylenimine-modified GO into an electrospun poly(D,L-lactic-co-glycolic acid) scaffold, which was then loaded with plasmid DNA (pDNA) to improve the growth and differentiation of mesenchymal stem cells via solid-phase gene delivery. Loading the nanofibers with pDNA nearly doubled the transfection efficiency compared to simply mixing it into the medium, improving it from 12.1% to 23.6%. Fang et al. [ 41 ] created an electrospun gelatin methacryloyl/polycaprolactone scaffold with rGO interspersed throughout to act as a nerve guidance conduit. The addition of low concentrations of rGO (0.25 and 0.5 wt%) increased the electroconductivity of the scaffold and improved nerve tissue regeneration.
Antibacterial applications
Both GO and rGO possess antibacterial properties that are ideal for antibacterial applications, as listed in Table 6 . Nanocarriers are generally directly applied to bacteria-containing media at high concentrations [ 42 , 43 ] or incorporated into a membrane [ 205 , 206 ]. The cells were inactivated as GO/rGO nanosheets aggregated and caused oxidative stress [ 96 , 106 ]. They can also be used in conjunction with photothermal therapy for cell ablation, increasing its effectiveness [ 43 , 108 ].
Halouane et al. [ 43 ] created rGO particles conjugated with nitrodopamine-coated magnetic nanoparticles (MP ND ) and pyrene-PEG with antifimbrial antibodies immobilized on the surface. The MP ND served to capture the pathogens, and NIR irradiation at a wavelength of 980 nm ablated the captured pathogens at temperatures up to 75 °C. Matharu et al. [ 210 ] generated poly(methyl 2-methylpropenoate) fiber meshes with dispersed GO nanosheets. Fibers with 8 wt% concentration of GO were most effective at bacterial reduction, with killing efficacy reaching 85 ± 1.4%, with the cytotoxic mechanism being attributed to the production of oxidative stress.
In summary, GO exhibits excellent properties suitable for various biomedical applications, including a high colloidal stability, good biocompatibility, and antibacterial properties. In particular, GO can be a good nanocarrier because it can be conjugated, embedded, or loaded with drugs, proteins, metals, and biomolecules. Moreover, it can be reduced to obtain highly conductive rGO at the expense of colloidal stability, which may be beneficial for biosensors and tissue engineering. In this review, we discuss the properties of GO and rGO, and the potential methods of functionalization with polymers and other molecules through covalent and noncovalent bonding. Through functionalization, GO and rGO have been engineered to be specific and functional nanocarriers of therapeutic biomolecules, such as anticancer drugs and genes, or modified for phototherapy, bioimaging, biosensing, tissue engineering, and antibacterial applications.
Despite the good biocompatibility of GO and rGO at lower concentrations, several mechanisms that may induce cytotoxicity and genotoxicity have been identified, including the aggregation of cell membrane damage and oxidative stress. Notably, there was a difference in cytotoxicity between mammalian cells and bacteria, where an increase in GO/rGO nanosheet size increased cytotoxicity in both, but the effect was more significant in bacteria. However, the comparison did not take into account the inherent size difference between mammalian cells and bacteria, and therefore, the relative size of the GO/rGO sheets as compared to the cells. Future studies should consider the cytotoxicity of GO/rGO as a function of the nanoparticle-to-cell size ratio. Furthermore, GO and rGO tended to aggregate in certain organs, even when functionalized. This is a cause for concern because while in vivo studies have deemed the use of these nanoparticles to be generally non-lethal, some toxic effects have been identified. With the long-term effects remaining largely unexplored, it is currently difficult to extend the use of GO/rGO as an in vivo nanocarrier in clinical trials. As such, GO- and rGO-based nanocomposite nanocarriers have great potential in biomedical applications; however, further studies on their effects in vivo are still necessary to advance the field.
Availability of data and materials
Not applicable.
Abbreviations
Two-dimensional
Camptothecin
Doxorubicin
Double-stranded DNA
Electrochemical
Electrochemiluminescence
Graphene oxide
Sulfuric acid
4-Hydroxycoumarin
Nitric acid
Iron oxide hybrid nanocomposite
Potassium chlorate
Layer-by-layer
Magnetic nanoparticles
Magnetic resonance
Near-infrared
Plasmid DNA
Polyethylene glycol
Poly-L-lysine
Polysebacic anhydride
Octaarginine
Reduced graphene oxide
SYBR Green I
Small interfering RNA
Single nucleotide polymorphism
Single-photon emission computed tomography
Single-stranded DNA
Makharza S, Cirillo G, Bachmatiuk A, Ibrahim I, Ioannides N, Trzebicka B, et al. Graphene oxide-based drug delivery vehicles: functionalization, characterization, and cytotoxicity evaluation. J Nanopart Res. 2013;15(12):2099.
Article Google Scholar
Marcano DC, Kosynkin DV, Berlin JM, Sinitskii A, Sun Z, Slesarev A, et al. Improved Synthesis of Graphene Oxide. ACS Nano. 2010;4(8):4806–14.
Article CAS Google Scholar
Hummers WS, Offeman RE. Preparation of graphitic oxide. J Am Chem Soc. 1958;80(6):1339.
Chen D, Feng H, Li J. Graphene oxide: preparation, functionalization, and electrochemical applications. Chem Rev. 2012;112(11):6027–53.
Khan ZU, Kausar A, Ullah H, Badshah A, Khan WU. A review of graphene oxide, graphene buckypaper, and polymer/graphene composites: Properties and fabrication techniques. J Plast Film Sheeting. 2016;32(4):336–79.
Siriviriyanun A, Popova M, Imae T, Kiew LV, Looi CY, Wong WF, et al. Preparation of graphene oxide/dendrimer hybrid carriers for delivery of doxorubicin. Chem Eng J. 2015;281:771–81.
Hung AH, Holbrook RJ, Rotz MW, Glasscock CJ, Mansukhani ND, MacRenaris KW, et al. Graphene oxide enhances cellular delivery of hydrophilic small molecules by co-incubation. ACS Nano. 2014;8(10):10168–77.
Lee J, Yim Y, Kim S, Choi M-H, Choi B-S, Lee Y, et al. In-depth investigation of the interaction between DNA and nano-sized graphene oxide. Carbon. 2016;97:92–8.
Khan ZU, Kausar A, Ullah H. a review on composite papers of graphene oxide, carbon nanotube, Polymer/GO, and Polymer/CNT: processing strategies, properties, and relevance. Polym Plast Technol Eng. 2016;55(6):559–81.
Kundu A, Nandi S, Das P, Nandi AK. Fluorescent graphene oxide via polymer grafting: an efficient nanocarrier for both hydrophilic and hydrophobic drugs. ACS Appl Mater Interfaces. 2015;7(6):3512–23.
Goenka S, Sant V, Sant S. Graphene-based nanomaterials for drug delivery and tissue engineering. J Control Release. 2014;173:75–88.
Cheng S-J, Chiu H-Y, Kumar PV, Hsieh KY, Yang J-W, Lin Y-R, et al. Simultaneous drug delivery and cellular imaging using graphene oxide. Biomater Sci. 2018;6(4):813–9.
Liu J, Cui L, Losic D. Graphene and graphene oxide as new nanocarriers for drug delivery applications. Acta Biomater. 2013;9(12):9243–57.
Park J, Kim B, Han J, Oh J, Park S, Ryu S, et al. Graphene oxide flakes as a cellular adhesive: prevention of reactive oxygen species mediated death of implanted cells for cardiac repair. ACS Nano. 2015;9(5):4987–99.
Guex LG, Sacchi B, Peuvot KF, Andersson RL, Pourrahimi AM, Strom V, et al. Experimental review: chemical reduction of graphene oxide (GO) to reduced graphene oxide (rGO) by aqueous chemistry. Nanoscale. 2017;9(27):9562–71.
Tung VC, Allen MJ, Yang Y, Kaner RB. High-throughput solution processing of large-scale graphene. Nat Nanotechnol. 2009;4(1):25–9.
Zhang L, Wang Z, Lu Z, Shen H, Huang J, Zhao Q, et al. PEGylated reduced graphene oxide as a superior ssRNA delivery system. J Mater Chem B. 2013;1(6):749–55.
Feng H, Cheng R, Zhao X, Duan X, Li J. A low-temperature method to produce highly reduced graphene oxide. Nat Commun. 2013;4(1):1–8.
CAS Google Scholar
Wang L, Park Y, Cui P, Bak S, Lee H, Lee SM, et al. Facile preparation of an n-type reduced graphene oxide field effect transistor at room temperature. Chem Commun. 2014;50(10):1224–6.
Akhavan O, Ghaderi E, Aghayee S, Fereydooni Y, Talebi A. The use of a glucose-reduced graphene oxide suspension for photothermal cancer therapy. J Mater Chem. 2012;22(27):13773–81.
Zhou X, Zhang J, Wu H, Yang H, Zhang J, Guo S. Reducing graphene oxide via hydroxylamine: a simple and efficient route to graphene. J Phys Chem C. 2011;115(24):11957–61.
Amarnath CA, Hong CE, Kim NH, Ku B-C, Kuila T, Lee JH. Efficient synthesis of graphene sheets using pyrrole as a reducing agent. Carbon. 2011;49(11):3497–502.
Chen D, Li L, Guo L. An environment-friendly preparation of reduced graphene oxide nanosheets via amino acid. Nanotechnology. 2011;22(32):325601.
Fan X, Peng W, Li Y, Li X, Wang S, Zhang G, et al. Deoxygenation of exfoliated graphite oxide under alkaline conditions: a green route to graphene preparation. Adv Mater. 2008;20(23):4490–3.
Wakeland S, Martinez R, Grey JK, Luhrs CC. Production of graphene from graphite oxide using urea as expansion–reduction agent. Carbon. 2010;48(12):3463–70.
He J, Fang L. Controllable synthesis of reduced graphene oxide. Curr Appl Phys. 2016;16(9):1152–8.
Xu C, Shi X, Ji A, Shi L, Zhou C, Cui Y. Fabrication and characteristics of reduced graphene oxide produced with different green reductants. PLoS ONE. 2015;10(12):e0144842.
Singh RK, Kumar R, Singh DP. Graphene oxide: strategies for synthesis, reduction and frontier applications. RSC Adv. 2016;6(69):64993–5011.
Shim G, Kim J-Y, Han J, Chung SW, Lee S, Byun Y, et al. Reduced graphene oxide nanosheets coated with an anti-angiogenic anticancer low-molecular-weight heparin derivative for delivery of anticancer drugs. J Control Release. 2014;189:80–9.
Song J, Yang X, Jacobson O, Lin L, Huang P, Niu G, et al. Sequential drug release and enhanced photothermal and photoacoustic effect of hybrid reduced graphene oxide-loaded ultrasmall gold nanorod vesicles for cancer therapy. ACS Nano. 2015;9(9):9199–209.
Kim M-G, Park JY, Miao W, Lee J, Oh Y-K. Polyaptamer DNA nanothread-anchored, reduced graphene oxide nanosheets for targeted delivery. Biomaterials. 2015;48:129–36.
Xu S, Zhang Z, Chu M. Long-term toxicity of reduced graphene oxide nanosheets: effects on female mouse reproductive ability and offspring development. Biomaterials. 2015;54:188–200.
Nahain A-A, Lee J-E, Jeong JH, Park SY. Photoresponsive fluorescent reduced graphene oxide by spiropyran conjugated hyaluronic acid for in vivo imaging and target delivery. Biomacromol. 2013;14(11):4082–90.
Senapati S, Patel DK, Ray B, Maiti P. Fluorescent-functionalized graphene oxide for selective labeling of tumor cells. J Biomed Mater Res A. 2019;107(9):1917–24.
Thirumalraj B, Dhenadhayalan N, Chen S-M, Liu Y-J, Chen T-W, Liang P-H, et al. Highly sensitive fluorogenic sensing of L-Cysteine in live cells using gelatin-stabilized gold nanoparticles decorated graphene nanosheets. Sensors Actuators B Chem. 2018;259:339–46.
Xia J, Xu T, Qing J, Wang L, Tang J. Detection of single nucleotide Polymorphisms by fluorescence embedded Dye SYBR Green I based on graphene oxide. Front Chem. 2021;9:631959.
Yuan Y, Liu G, Yuan R, Chai Y, Gan X, Bai L. Dendrimer functionalized reduced graphene oxide as nanocarrier for sensitive pseudobienzyme electrochemicalaptasensor. Biosens Bioelectron. 2013;42:474–80.
Zhao M, Chai XDY-Q, Han J, Gui G-F, Yuan R, Zhuo Y. A reagentless electrochemiluminescent immunosensor for apurinic/apyrimidinic endonuclease 1 detection based on the new Ru (bpy) 32+/bi-arginine system. Anal Chim Acta. 2014;846:36–43.
Magaz A, Li X, Gough JE, Blaker JJ. Graphene oxide and electroactive reduced graphene oxide-based composite fibrous scaffolds for engineering excitable nerve tissue. Mater Sci Eng C. 2021;119:111632.
Zhang C, Wang X, Fan S, Lan P, Cao C, Zhang Y. Silk fibroin/reduced graphene oxide composite mats with enhanced mechanical properties and conductivity for tissue engineering. Colloids Surf B Biointerfaces. 2021;197:111444.
Fang X, Guo H, Zhang W, Fang H, Li Q, Bai S, et al. Reduced graphene oxide–GelMA–PCL hybrid nanofibers for peripheral nerve regeneration. J Mater Chem B. 2020;8(46):10593–601.
Menazea A, Ahmed M. Synthesis and antibacterial activity of graphene oxide decorated by silver and copper oxide nanoparticles. J Mol Struct. 2020;1218:128536.
Halouane F, Jijie R, Meziane D, Li C, Singh SK, Bouckaert J, et al. Selective isolation and eradication of E. coli associated with urinary tract infections using anti-fimbrial modified magnetic reduced graphene oxide nanoheaters. J Mater Chem B. 2017;5(40):8133–42.
Singh DP, Herrera CE, Singh B, Singh S, Singh RK, Kumar R. Graphene oxide: an efficient material and recent approach for biotechnological and biomedical applications. Mater Sci Eng C. 2018;86:173–97.
Santhosh K, Modak MD, Paik P. Graphene oxide for biomedical applications. J Nanomed Res. 2017;5(6):1–6.
Google Scholar
Plachá D, Jampilek J. Graphenic materials for biomedical applications. Nanomaterials. 2019;9(12):1758.
Tadyszak K, Wychowaniec JK, Litowczenko J. Biomedical applications of graphene-based structures. Nanomaterials. 2018;8(11):944.
Zare P, Aleemardani M, Seifalian A, Bagher Z, Seifalian AM. Graphene oxide: opportunities and challenges in biomedicine. Nanomaterials. 2021;11(5):1083.
Dreyer DR, Park S, Bielawski CW, Ruoff RS. The chemistry of graphene oxide. Chem Soc Rev. 2010;39(1):228–40.
Poh HL, Sanek F, Ambrosi A, Zhao G, Sofer Z, Pumera M. Graphenes prepared by Staudenmaier, Hofmann and Hummers methods with consequent thermal exfoliation exhibit very different electrochemical properties. Nanoscale. 2012;4(11):3515–22.
Chen J, Li Y, Huang L, Li C, Shi G. High-yield preparation of graphene oxide from small graphite flakes via an improved Hummers method with a simple purification process. Carbon. 2015;81:826–34.
Adetayo A, Runsewe D. Synthesis and fabrication of graphene and graphene oxide: a review. Open J Compos Mater. 2019;9(02):207.
Tang L, Li X, Ji R, Teng KS, Tai G, Ye J, et al. Bottom-up synthesis of large-scale graphene oxide nanosheets. J Mater Chem. 2012;22(12):5676–83.
Pei S, Wei Q, Huang K, Cheng H-M, Ren W. Green synthesis of graphene oxide by seconds timescale water electrolytic oxidation. Nat Commun. 2018;9(1):1–9.
Shen Y, Boffa V, Corazzari I, Qiao A, Tao H, Yue Y. Revealing hidden endotherm of Hummers’ graphene oxide during low-temperature thermal reduction. Carbon. 2018;138:337–47.
Aslam M, Kalyar M, Raza Z. Synthesis and structural characterization of separate graphene oxide and reduced graphene oxide nanosheets. Mater Res Express. 2016;3(10):105036.
Bagri A, Mattevi C, Acik M, Chabal YJ, Chhowalla M, Shenoy VB. Structural evolution during the reduction of chemically derived graphene oxide. Nat Chem. 2010;2(7):581–7.
Akhavan O. The effect of heat treatment on formation of graphene thin films from graphene oxide nanosheets. Carbon. 2010;48(2):509–19.
Shao Y, Wang J, Engelhard M, Wang C, Lin Y. Facile and controllable electrochemical reduction of graphene oxide and its applications. J Mater Chem. 2010;20(4):743–8.
Agarwal V, Zetterlund PB. Strategies for reduction of graphene oxide-a comprehensive review. Chem Eng J. 2020;405:127018.
Pei S, Cheng H-M. The reduction of graphene oxide. Carbon. 2012;50(9):3210–28.
Feng J, Ye Y, Xiao M, Wu G, Ke Y. Synthetic routes of the reduced graphene oxide. Chem Pap. 2020;74:3767–83.
Guo H, Peng M, Zhu Z, Sun L. Preparation of reduced graphene oxide by infrared irradiation induced photothermal reduction. Nanoscale. 2013;5(19):9040–8.
Sengupta I, Chakraborty S, Talukdar M, Pal SK, Chakraborty S. Thermal reduction of graphene oxide: how temperature influences purity. J Mater Res. 2018;33(23):4113–22.
Khiem TN, Chinh HD, Van Tuan P, Tan VT. Adsorption capacities of reduced graphene oxide: effect of reductants. Mater Res Express. 2019;6(7):075615.
Phukan P, Narzary R, Sahu PP. A green approach to fast synthesis of reduced graphene oxide using alcohol for tuning semiconductor property. Mater Sci Semicond Process. 2019;104:104670.
Liu W, Speranza G. Tuning the oxygen content of reduced graphene oxide and effects on its properties. ACS Omega. 2021;6(9):6195–205.
Loh KP, Bao Q, Eda G, Chhowalla M. Graphene oxide as a chemically tunable platform for optical applications. Nat Chem. 2010;2(12):1015.
Shen J, Hu Y, Shi M, Lu X, Qin C, Li C, et al. Fast and facile preparation of graphene oxide and reduced graphene oxide nanoplatelets. Chem Mater. 2009;21(15):3514–20.
Amieva EJC, López-Barroso J, Martínez-Hernández AL, Velasco-Santos C. Graphene-based materials functionalization with natural polymeric biomolecules. Recent Adv Gaphene Res. 2016;1:257–98.
Lesiak B, Trykowski G, Tóth J, Biniak S, Kövér L, Rangam N, et al. Chemical and structural properties of reduced graphene oxide—dependence on the reducing agent. J Mater Sci. 2021;56(5):3738–54.
Shams M, Guiney LM, Huang L, Ramesh M, Yang X, Hersam MC, et al. Influence of functional groups on the degradation of graphene oxide nanomaterials. Environ Sci Nano. 2019;6(7):2203–14.
Hu Y, Song S, Lopez-Valdivieso A. Effects of oxidation on the defect of reduced graphene oxides in graphene preparation. J Colloid Interface Sci. 2015;450:68–73.
Akhavan O. Bacteriorhodopsin as a superior substitute for hydrazine in chemical reduction of single-layer graphene oxide sheets. Carbon. 2015;81:158–66.
Silva C, Simon F, Friedel P, Pötschke P, Zimmerer C. Elucidating the chemistry behind the reduction of graphene oxide using a green approach with polydopamine. Nanomaterials. 2019;9(6):902.
López-Díaz D, Delgado-Notario JA, Clericò V, Diez E, Merchán MD, Velázquez MM. Towards understanding the Raman spectrum of graphene oxide: the effect of the chemical composition. Coatings. 2020;10(6):524.
Hoseini-Ghahfarokhi M, Mirkiani S, Mozaffari N, Sadatlu MAA, Ghasemi A, Abbaspour S, et al. Applications of Graphene and Graphene oxide in smart drug/gene delivery: is the world still flat? Int J Nanomedicine. 2020;15:9469.
Azizighannad S, Mitra S. Stepwise reduction of graphene oxide (GO) and its effects on chemical and colloidal properties. Sci Rep. 2018;8(1):1–8.
Luo D, Zhang G, Liu J, Sun X. Evaluation criteria for reduced graphene oxide. J Phys Chem C. 2011;115(23):11327–35.
Qi Y, Xia T, Li Y, Duan L, Chen W. Colloidal stability of reduced graphene oxide materials prepared using different reducing agents. Environ Sci Nano. 2016;3(5):1062–71.
Suk JW, Piner RD, An J, Ruoff RS. Mechanical properties of monolayer graphene oxide. ACS Nano. 2010;4(11):6557–64.
Gómez-Navarro C, Burghard M, Kern K. Elastic properties of chemically derived single graphene sheets. Nano Lett. 2008;8(7):2045–9.
Konios D, Stylianakis MM, Stratakis E, Kymakis E. Dispersion behaviour of graphene oxide and reduced graphene oxide. J Colloid Interface Sci. 2014;430:108–12.
Sharma S, Cheng C-A, Santiago SRM, Feria DN, Yuan C-T, Chang S-H, et al. Aggregation-induced negative differential resistance in graphene oxide quantum dots. Phys Chem Chem Phys. 2021;23(31):16909–14.
Shang J, Ma L, Li J, Ai W, Yu T, Gurzadyan GG. The origin of fluorescence from graphene oxide. Sci Rep. 2012;2(1):1–8.
Aunkor M, Mahbubul I, Saidur R, Metselaar H. Deoxygenation of graphene oxide using household baking soda as a reducing agent: a green approach. RSC Adv. 2015;5(86):70461–72.
Shi H, Wang C, Sun Z, Zhou Y, Jin K, Redfern SA, et al. Tuning the nonlinear optical absorption of reduced graphene oxide by chemical reduction. Opt Express. 2014;22(16):19375–85.
Hashemi M, Omidi M, Muralidharan B, Smyth H, Mohagheghi MA, Mohammadi J, et al. Evaluation of the photothermal properties of a reduced graphene oxide/arginine nanostructure for near-infrared absorption. ACS Appl Mater Interfaces. 2017;9(38):32607–20.
Zheng P, Wu N. Fluorescence and sensing applications of graphene oxide and graphene quantum dots: a review. Chem Asian J. 2017;12(18):2343–53.
Chien CT, Li SS, Lai WJ, Yeh YC, Chen HA, Chen IS, et al. Tunable photoluminescence from graphene oxide. Angew Chem Int Ed. 2012;51(27):6662–6.
Yang K, Wan J, Zhang S, Tian B, Zhang Y, Liu Z. The influence of surface chemistry and size of nanoscale graphene oxide on photothermal therapy of cancer using ultra-low laser power. Biomaterials. 2012;33(7):2206–14.
Robinson JT, Tabakman SM, Liang Y, Wang H, Sanchez Casalongue H, Vinh D, et al. Ultrasmall reduced graphene oxide with high near-infrared absorbance for photothermal therapy. J Am Chem Soc. 2011;133(17):6825–31.
Lammel T, Boisseaux P, Fernández-Cruz M-L, Navas JM. Internalization and cytotoxicity of graphene oxide and carboxyl graphene nanoplatelets in the human hepatocellular carcinoma cell line Hep G2. Part Fibre Toxicol. 2013;10(1):1–21.
Gurunathan S, Han JW, Kim J-H. Green chemistry approach for the synthesis of biocompatible graphene. Int J Nanomedicine. 2013;8:2719.
Muthoosamy K, Bai RG, Abubakar IB, Sudheer SM, Lim HN, Loh H-S, et al. Exceedingly biocompatible and thin-layered reduced graphene oxide nanosheets using an eco-friendly mushroom extract strategy. Int J Nanomedicine. 2015;10:1505.
Liu S, Zeng TH, Hofmann M, Burcombe E, Wei J, Jiang R, et al. Antibacterial activity of graphite, graphite oxide, graphene oxide, and reduced graphene oxide: membrane and oxidative stress. ACS Nano. 2011;5(9):6971–80.
Liao K-H, Lin Y-S, Macosko CW, Haynes CL. Cytotoxicity of graphene oxide and graphene in human erythrocytes and skin fibroblasts. ACS Appl Mater Interfaces. 2011;3(7):2607–15.
Li R, Guiney LM, Chang CH, Mansukhani ND, Ji Z, Wang X, et al. Surface oxidation of graphene oxide determines membrane damage, lipid peroxidation, and cytotoxicity in macrophages in a pulmonary toxicity model. ACS Nano. 2018;12(2):1390–402.
Wu K, Zhou Q, Ouyang S. Direct and indirect genotoxicity of graphene family nanomaterials on DNA—a review. Nanomaterials. 2021;11(11):2889.
Qu G, Wang X, Liu Q, Liu R, Yin N, Ma J, et al. The ex vivo and in vivo biological performances of graphene oxide and the impact of surfactant on graphene oxide’s biocompatibility. J Environ Sci. 2013;25(5):873–81.
Yang K, Gong H, Shi X, Wan J, Zhang Y, Liu Z. In vivo biodistribution and toxicology of functionalized nano-graphene oxide in mice after oral and intraperitoneal administration. Biomaterials. 2013;34(11):2787–95.
Singh SK, Singh MK, Kulkarni PP, Sonkar VK, Grácio JJ, Dash D. Amine-modified graphene: thrombo-protective safer alternative to graphene oxide for biomedical applications. ACS Nano. 2012;6(3):2731–40.
Ahmadian H, Hashemi E, Akhavan O, Shamsara M, Hashemi M, Farmany A, et al. Apoptotic and anti-apoptotic genes transcripts patterns of graphene in mice. Mater Sci Eng C. 2017;71:460–4.
Souza JP, Baretta JF, Santos F, Paino IM, Zucolotto V. Toxicological effects of graphene oxide on adult zebrafish (Danio rerio). Aquat Toxicol. 2017;186:11–8.
Yunus MA, Ramli MM, Osman NH, Mohamed R. Stimulation of innate and adaptive immune cells with graphene oxide and reduced graphene oxide affect cancer progression. Arch Immunol Ther Exp (Warsz). 2021;69(1):1–16.
Liu S, Hu M, Zeng TH, Wu R, Jiang R, Wei J, et al. Lateral dimension-dependent antibacterial activity of graphene oxide sheets. Langmuir. 2012;28(33):12364–72.
Zhao L, Duan G, Yang Z, Weber JK, Liu X, Lu S, et al. Particle size-dependent antibacterial activity and murine cell cytotoxicity induced by graphene oxide nanomaterials. J Nanomater. 2016;2016:1–9.
Hatamie S, Shih P-J, Zomorod MS, Heravi P, Ahadian MM, Hatami N. Hyperthermia response of PEGylated magnetic graphene nanocomposites for heating applications and accelerate antibacterial activity using magnetic fluid hyperthermia. Appl Phys A. 2020;126(4):1–10.
Tikum AF, Ko JW, Kim S, Kim J. Reduced graphene oxide-oligonucleotide interfaces: understanding based on electrochemical oxidation of guanines. ACS Omega. 2018;3(11):15464–70.
Achari A, Datta K, De M, Dravid V, Eswaramoorthy M. Amphiphilic aminoclay–RGO hybrids: a simple strategy to disperse a high concentration of RGO in water. Nanoscale. 2013;5(12):5316–20.
He Y, Jiao B, Tang H. Interaction of single-stranded DNA with graphene oxide: fluorescence study and its application for S1 nuclease detection. RSC Adv. 2014;4(35):18294–300.
Kim H, Kim WJ. Photothermally controlled gene delivery by reduced graphene oxide–polyethylenimine nanocomposite. Small. 2014;10(1):117–26.
Sarkar K, Madras G, Chatterjee K. Dendron conjugation to graphene oxide using click chemistry for efficient gene delivery. RSC Adv. 2015;5(62):50196–211.
Roy S, Jaiswal A. DNA binding and NIR triggered DNA release from quaternary ammonium modified poly (allylamine hydrochloride) functionalized and folic acid conjugated reduced graphene oxide nanocomposites. Int J Biol Macromol. 2020;153:931–41.
Holt BD, Arnold AM, Sydlik SA. In it for the long haul: the cytocompatibility of aged graphene oxide and its degradation products. Adv Healthc Mater. 2016;5(23):3056–66.
Dimiev AM, Alemany LB, Tour JM. Graphene oxide. Origin of acidity, its instability in water, and a new dynamic structural model. ACS Nano. 2013;7(1):576–88.
Kurapati R, Martìn C, Palermo V, Nishina Y, Bianco A. Biodegradation of graphene materials catalyzed by human eosinophil peroxidase. Faraday Discuss. 2020;227:189–203.
Arnold AM, Holt BD, Tang C, Sydlik SA. Phosphate modified graphene oxide: Long–term biodegradation and cytocompatibility. Carbon. 2019;154:342–9.
Mukherjee SP, Gliga AR, Lazzaretto B, Brandner B, Fielden M, Vogt C, et al. Graphene oxide is degraded by neutrophils and the degradation products are non-genotoxic. Nanoscale. 2018;10(3):1180–8.
Lalwani G, Xing W, Sitharaman B. Enzymatic degradation of oxidized and reduced graphene nanoribbons by lignin peroxidase. J Mater Chem B. 2014;2(37):6354–62.
Sima LE, Chiritoiu G, Negut I, Grumezescu V, Orobeti S, Munteanu CVA, et al. Functionalized graphene oxide thin films for anti-tumor drug delivery to melanoma cells. Front Chem. 2020;8:184.
de Sousa M, Visani de Luna LA, Fonseca LC, Giorgio S, Alves OL. Folic-acid-functionalized graphene oxide nanocarrier: synthetic approaches, characterization, drug delivery study, and antitumor screening. ACS Appl Nano Mater. 2018;1(2):922–32.
Jana B, Mondal G, Biswas A, Chakraborty I, Saha A, Kurkute P, et al. Dual functionalized graphene oxide serves as a carrier for delivering oligohistidine- and biotin-tagged biomolecules into cells. Macromol Biosci. 2013;13(11):1478–84.
Kazempour M, Namazi H, Akbarzadeh A, Kabiri R. Synthesis and characterization of PEG-functionalized graphene oxide as an effective pH-sensitive drug carrier. Artif Cells Nanomed Biotechnol. 2019;47(1):90–4.
Tan Q, Qiu J, Luo X, Chen Y, Liu Y, Wei H, et al. Using functionalized graphene oxide as carrier for immobilization of Glutaryl-7-aminocephalosporanic acid acylase. J Nanosci Nanotechnol. 2019;19(5):2501–5.
Bao H, Pan Y, Ping Y, Sahoo NG, Wu T, Li L, et al. Chitosan-functionalized graphene oxide as a nanocarrier for drug and gene delivery. Small. 2011;7(11):1569–78.
Wang X, Sun Q, Cui C, Li J, Wang Y. Anti-HER2 functionalized graphene oxide as survivin-siRNA delivery carrier inhibits breast carcinoma growth in vitro and in vivo. Drug Des Devel Ther. 2018;12:2841–55.
Li J, Ge X, Cui C, Zhang Y, Wang Y, Wang X, et al. Preparation and characterization of functionalized graphene oxide carrier for siRNA delivery. Int J Mol Sci. 2018;19(10):3202.
Gao J, Bao F, Feng L, Shen K, Zhu Q, Wang D, et al. Functionalized graphene oxide modified polysebacic anhydride as drug carrier for levofloxacin controlled release. RSC Adv. 2011;1(9):1737–44.
Ma X, Tao H, Yang K, Feng L, Cheng L, Shi X, et al. A functionalized graphene oxide-iron oxide nanocomposite for magnetically targeted drug delivery, photothermal therapy, and magnetic resonance imaging. Nano Res. 2012;5(3):199–212.
Georgakilas V, Tiwari JN, Kemp KC, Perman JA, Bourlinos AB, Kim KS, et al. Noncovalent functionalization of graphene and graphene oxide for energy materials, biosensing, catalytic, and biomedical applications. Chem Rev. 2016;116(9):5464–519.
Karki N, Tiwari H, Tewari C, Rana A, Pandey N, Basak S, et al. Functionalized graphene oxide as a vehicle for targeted drug delivery and bioimaging applications. J Mater Chem B. 2020;8(36):8116–48.
Kavinkumar T, Varunkumar K, Ravikumar V, Manivannan S. Anticancer activity of graphene oxide-reduced graphene oxide-silver nanoparticle composites. J Colloid Interface Sci. 2017;505:1125–33.
Xie M, Lei H, Zhang Y, Xu Y, Shen S, Ge Y, et al. Non-covalent modification of graphene oxide nanocomposites with chitosan/dextran and its application in drug delivery. RSC Adv. 2016;6(11):9328–37.
Li Y, Lu Z, Li Z, Nie G, Fang Y. Cellular uptake and distribution of graphene oxide coated with layer-by-layer assembled polyelectrolytes. J Nanopart Res. 2014;16(5):2384.
Mu Q, Su G, Li L, Gilbertson BO, Yu LH, Zhang Q, et al. Size-dependent cell uptake of protein-coated graphene oxide nanosheets. ACS Appl Mater Interfaces. 2012;4(4):2259–66.
Hashemi M, Yadegari A, Yazdanpanah G, Jabbehdari S, Omidi M, Tayebi L. Functionalized R9–reduced graphene oxide as an efficient nano-carrier for hydrophobic drug delivery. RSC Adv. 2016;6(78):74072–84.
He D, Li X, He X, Wang K, Tang J, Yang X, et al. Noncovalent assembly of reduced graphene oxide and alkyl-grafted mesoporous silica: an effective drug carrier for near-infrared light-responsive controlled drug release. J Mater Chem B. 2015;3(27):5588–94.
Ma N, Zhang B, Liu J, Zhang P, Li Z, Luan Y. Green fabricated reduced graphene oxide: evaluation of its application as nano-carrier for pH-sensitive drug delivery. Int J Pharm. 2015;496(2):984–92.
Chen Y-W, Chen P-J, Hu S-H, Chen I-W, Chen S-Y. NIR-triggered synergic photo-chemothermal therapy delivered by reduced graphene oxide/carbon/mesoporous silica nanocookies. Adv Funct Mater. 2014;24(4):451–9.
Vinothini K, Rajendran NK, Rajan M, Ramu A, Marraiki N, Elgorban AM. A magnetic nanoparticle functionalized reduced graphene oxide-based drug carrier system for a chemo-photodynamic cancer therapy. New J Chem. 2020;44(14):5265–77.
Lin S, Ruan J, Wang S. Biosynthesized of reduced graphene oxide nanosheets and its loading with paclitaxel for their anti cancer effect for treatment of lung cancer. J Photochem Photobiol B: Biol. 2019;191:13–7.
Jafarizad A, Aghanejad A, Sevim M, Metin Ö, Barar J, Omidi Y, et al. Gold nanoparticles and reduced graphene oxide-gold nanoparticle composite materials as covalent drug delivery systems for breast cancer treatment. ChemistrySelect. 2017;2(23):6663–72.
Miao W, Shim G, Kang CM, Lee S, Choe YS, Choi H-G, et al. Cholesteryl hyaluronic acid-coated, reduced graphene oxide nanosheets for anti-cancer drug delivery. Biomaterials. 2013;34(37):9638–47.
Lerra L, Farfalla A, Sanz B, Cirillo G, Vittorio O, Le Grand M, et al. Graphene oxide functional nanohybrids with magnetic nanoparticles for improved vectorization of doxorubicin to neuroblastoma cells. Pharmaceutics. 2019;11(1):3.
Poudel K, Banstola A, Tran TH, Thapa RK, Gautam M, Ou W, et al. Hyaluronic acid wreathed, trio-stimuli receptive and on-demand triggerable nanoconstruct for anchored combinatorial cancer therapy. Carbohydr Polym. 2020;249:116815.
Li R, Wang Y, Du J, Wang X, Duan A, Gao R, et al. Graphene oxide loaded with tumor-targeted peptide and anti-cancer drugs for cancer target therapy. Sci Rep. 2021;11(1):1–10.
Işıklan N, Hussien NA, Türk M. Synthesis and drug delivery performance of gelatin-decorated magnetic graphene oxide nanoplatform. Colloids Surf Physicochem Eng Aspects. 2021;616:126256.
Kim H, Lee D, Kim J, Kim T-i, Kim WJ. Photothermally triggered cytosolic drug delivery via endosome disruption using a functionalized reduced graphene oxide. ACS Nano. 2013;7(8):6735–46.
Gupta N, Bhagat S, Singh M, Jangid AK, Bansal V, Singh S, et al. Site-specific delivery of a natural chemotherapeutic agent to human lung cancer cells using biotinylated 2D rGO nanocarriers. Mater Sci Eng C. 2020;112:110884.
Jiao D, Wang J, Yu W, Zhang N, Zhang K, Bai Y. Gelatin reduced graphene oxide nanosheets as kartogenin nanocarrier induces rat ADSCs chondrogenic differentiation combining with autophagy modification. Materials. 2021;14(5):1053.
Dhanavel S, Revathy T, Sivaranjani T, Sivakumar K, Palani P, Narayanan V, et al. 5-Fluorouracil and curcumin co-encapsulated chitosan/reduced graphene oxide nanocomposites against human colon cancer cell lines. Polym Bull. 2020;77(1):213–33.
Liu X, Wu X, Xing Y, Zhang Y, Zhang X, Pu Q, et al. Reduced graphene oxide/mesoporous silica nanocarriers for pH-triggered drug release and photothermal therapy. ACS Appl Bio Mater. 2020;3(5):2577–87.
Latief U, Umar MF, Ahmad R. Nrf2 protein as a therapeutic target during diethylnitrosamine-induced liver injury ameliorated by β-carotene-reduced graphene oxide (βC-rGO) nanocomposite. Int J Biol Macromol. 2019;137:346–57.
Hu Z, Zhang D, Yu L, Huang Y. Light-triggered C 60 release from a graphene/cyclodextrin nanoplatform for the protection of cytotoxicity induced by nitric oxide. J Mater Chem B. 2018;6(3):518–26.
Liu R, Zhang H, Zhang F, Wang X, Liu X, Zhang Y. Polydopamine doped reduced graphene oxide/mesoporous silica nanosheets for chemo-photothermal and enhanced photothermal therapy. Mater Sci Eng C. 2019;96:138–45.
Zhang X, Nan X, Shi W, Sun Y, Su H, He Y, et al. Polydopamine-functionalized nanographene oxide: a versatile nanocarrier for chemotherapy and photothermal therapy. Nanotechnology. 2017;28(29):295102.
Imani R, Shao W, Taherkhani S, Emami SH, Prakash S, Faghihi S. Dual-functionalized graphene oxide for enhanced siRNA delivery to breast cancer cells. Colloids Surf B Biointerfaces. 2016;147:315–25.
Alipour N, Namazi H. Chelating ZnO-dopamine on the surface of graphene oxide and its application as pH-responsive and antibacterial nanohybrid delivery agent for doxorubicin. Mater Sci Eng C. 2020;108:110459.
Chawda N, Basu M, Majumdar D, Poddar R, Mahapatra SK, Banerjee I. Engineering of gadolinium-decorated graphene oxide nanosheets for multimodal bioimaging and drug delivery. ACS Omega. 2019;4(7):12470–9.
Mosaiab T, In I, Park SY. Temperature and pH-tunable fluorescence nanoplatform with graphene oxide and BODIPY-conjugated polymer for cell imaging and therapy. Macromol Rapid Commun. 2013;34(17):1408–15.
Lu Y-J, Lin P-Y, Huang P-H, Kuo C-Y, Shalumon K, Chen M-Y, et al. Magnetic graphene oxide for dual targeted delivery of doxorubicin and photothermal therapy. Nanomaterials. 2018;8(4):193.
Xie M, Deng T, Li J, Shen H. The camouflage of graphene oxide by red blood cell membrane with high dispersibility for cancer chemotherapy. J Colloid Interface Sci. 2021;591:290–9.
Taheri-Kafrani A, Shirzadfar H, Kajani AA, Kudhair BK, Mohammed LJ, Mohammadi S, et al. Functionalized graphene oxide/Fe3O4 nanocomposite: a biocompatible and robust nanocarrier for targeted delivery and release of anticancer agents. J Biotechnol. 2021;331:26–36.
Assali A, Akhavan O, Adeli M, Razzazan S, Dinarvand R, Zanganeh S, et al. Multifunctional core-shell nanoplatforms (gold@ graphene oxide) with mediated NIR thermal therapy to promote miRNA delivery. Nanomed Nanotechnol Biol Med. 2018;14(6):1891–903.
Assali A, Akhavan O, Mottaghitalab F, Adeli M, Dinarvand R, Razzazan S, et al. Cationic graphene oxide nanoplatform mediates miR-101 delivery to promote apoptosis by regulating autophagy and stress. Int J Nanomedicine. 2018;13:5865.
Gulzar A, Xu J, Yang D, Xu L, He F, Gai S, et al. Nano-graphene oxide-UCNP-Ce6 covalently constructed nanocomposites for NIR-mediated bioimaging and PTT/PDT combinatorial therapy. Dalton Trans. 2018;47(11):3931–9.
Georgieva M, Gospodinova Z, Keremidarska-Markova M, Kamenska T, Gencheva G, Krasteva N. PEGylated nanographene oxide in combination with near-infrared laser irradiation as a smart nanocarrier in colon cancer targeted therapy. Pharmaceutics. 2021;13(3):424.
Sharker SM, Lee JE, Kim SH, Jeong JH, In I, Lee H, et al. pH triggered in vivo photothermal therapy and fluorescence nanoplatform of cancer based on responsive polymer-indocyanine green integrated reduced graphene oxide. Biomaterials. 2015;61:229–38.
Zhang D-Y, Zheng Y, Tan C-P, Sun J-H, Zhang W, Ji L-N, et al. Graphene oxide decorated with Ru (II)–polyethylene glycol complex for lysosome-targeted imaging and photodynamic/photothermal therapy. ACS Appl Mater Interfaces. 2017;9(8):6761–71.
Zeng W-N, Yu Q-P, Wang D, Liu J-L, Yang Q-J, Zhou Z-K, et al. Mitochondria-targeting graphene oxide nanocomposites for fluorescence imaging-guided synergistic phototherapy of drug-resistant osteosarcoma. J Nanobiotechnology. 2021;19(1):1–19.
Leitão MM, Alves CG, de Melo-Diogo D, Lima-Sousa R, Moreira AF, Correia IJ. Sulfobetaine methacrylate-functionalized graphene oxide-IR780 nanohybrids aimed at improving breast cancer phototherapy. RSC Adv. 2020;10(63):38621–30.
Jun SW, Manivasagan P, Kwon J, Mondal S, Ly CD, Lee J, et al. Folic acid–conjugated chitosan-functionalized graphene oxide for highly efficient photoacoustic imaging-guided tumor-targeted photothermal therapy. Int J Biol Macromol. 2020;155:961–71.
Baipaywad P, Ryu N, Im S-S, Lee U, Son HB, Kim WJ, et al. Facile preparation of poly (N-isopropylacrylamide)/graphene oxide nanocomposites for chemo-photothermal therapy. Des Monomers Polym. 2022;25(1):245–53.
Ferrer-Ugalde A, Sandoval S, Pulagam KR, Muñoz-Juan A, Laromaine A, Llop J, et al. Radiolabeled cobaltabis (dicarbollide) anion-graphene oxide nanocomposites for in vivo bioimaging and boron delivery. ACS Appl Nano Mater. 2021;4(2):1613–25.
Yu G, Yang J, Fu X, Wang Z, Shao L, Mao Z, et al. A supramolecular hybrid material constructed from graphene oxide and a pillar [6] arene-based host–guest complex as an ultrasound and photoacoustic signal nanoamplifier. Mater Horiz. 2018;5(3):429–35.
Qian R, Maiti D, Zhong J, Xiong S, Zhou H, Zhu R, et al. Multifunctional nano-graphene based nanocomposites for multimodal imaging guided combined radioisotope therapy and chemotherapy. Carbon. 2019;149:55–62.
Llenas M, Sandoval S, Costa PM, Oró-Solé J, Lope-Piedrafita S, Ballesteros B, et al. Microwave-assisted synthesis of SPION-reduced graphene oxide hybrids for magnetic resonance imaging (MRI). Nanomaterials. 2019;9(10):1364.
Bugárová N, Annušová A, Bodík M, Šiffalovič P, Labudová M, Kajanová I, et al. Molecular targeting of bioconjugated graphene oxide nanocarriers revealed at a cellular level using label-free Raman imaging. Nanomed Nanotechnol Biol Med. 2020;30:102280.
Lee SY, Kim SH, Kim SM, Lee H, Lee G, Park SY. Tunable and selective detection of cancer cells using a betainized zwitterionic polymer with BODIPY and graphene oxide. New J Chem. 2014;38(6):2225–8.
Yogesh GK, Shuaib E, Roopmani P, Gumpu MB, Krishnan UM, Sastikumar D. Synthesis, characterization and bioimaging application of laser-ablated graphene-oxide nanoparticles (nGOs). Diamond Relat Mater. 2020;104:107733.
Shin H, Park S-J, Kim J, Lee J-S, Min D-H. A graphene oxide-based fluorescent nanosensor to identify antiviral agents via a drug repurposing screen. Biosens Bioelectron. 2021;183:113208.
Thangamuthu M, Hsieh KY, Kumar PV, Chen G-Y. Graphene-and graphene oxide-based nanocomposite platforms for electrochemical biosensing applications. Int J Mol Sci. 2019;20(12):2975.
Yu H, Guo W, Lu X, Xu H, Yang Q, Tan J, et al. Reduced graphene oxide nanocomposite based electrochemical biosensors for monitoring foodborne pathogenic bacteria: a review. Food Control. 2021;127:108117.
Wang X, Xu R, Sun X, Wang Y, Ren X, Du B, et al. Using reduced graphene oxide-Ca: CdSe nanocomposite to enhance photoelectrochemical activity of gold nanoparticles functionalized tungsten oxide for highly sensitive prostate specific antigen detection. Biosens Bioelectron. 2017;96:239–45.
Yang H, Wang H, Xiong C, Chai Y, Yuan R. Highly sensitive electrochemiluminescence immunosensor based on ABEI/H2O2 system with PFO dots as enhancer for detection of kidney injury molecule-1. Biosens Bioelectron. 2018;116:16–22.
Balcioglu M, Buyukbekar BZ, Yavuz MS, Yigit MV. Smart-polymer-functionalized graphene nanodevices for thermo-switch-controlled biodetection. ACS Biomater Sci Eng. 2015;1(1):27–36.
Ghorbanzadeh Sheish S, Emadi R, Ahmadian M, Sadeghzade S, Tavangarian F. Fabrication and characterization of polyvinylpyrrolidone-eggshell membrane-reduced graphene oxide nanofibers for tissue engineering applications. Polymers. 2021;13(6):913.
Gohari PHM, Nazarpak MH, Solati-Hashjin M. The effect of adding reduced graphene oxide to electrospun polycaprolactone scaffolds on MG-63 cells activity. Mater Today Commun. 2021;27:102287.
Narayanan KB, Park GT, Han SS. Electrospun poly (vinyl alcohol)/reduced graphene oxide nanofibrous scaffolds for skin tissue engineering. Colloids Surf B Biointerfaces. 2020;191:110994.
Trucco D, Vannozzi L, Teblum E, Telkhozhayeva M, Nessim GD, Affatato S, et al. Graphene oxide-doped gellan gum–PEGDA bilayered hydrogel mimicking the mechanical and lubrication properties of articular cartilage. Adv Healthc Mater. 2021;10(7):2001434.
Li Y, Liao C, Tjong SC. Synthetic biodegradable aliphatic polyester nanocomposites reinforced with nanohydroxyapatite and/or graphene oxide for bone tissue engineering applications. Nanomaterials. 2019;9(4):590.
Jiao D, Zheng A, Liu Y, Zhang X, Wang X, Wu J, et al. Bidirectional differentiation of BMSCs induced by a biomimetic procallus based on a gelatin-reduced graphene oxide reinforced hydrogel for rapid bone regeneration. Bioact Mater. 2021;6(7):2011–28.
de Lacerda Dantas PC, Martins-Júnior PA, Coutinho DCO, Andrade VB, Valverde TM, de Souza ÁE, et al. Nanohybrid composed of graphene oxide functionalized with sodium hyaluronate accelerates bone healing in the tibia of rats. Mater Sci Eng C. 2021;123:111961.
Jiang L-B, Ding S-L, Ding W, Su D-H, Zhang F-X, Zhang T-W, et al. Injectable sericin based nanocomposite hydrogel for multi-modal imaging-guided immunomodulatory bone regeneration. Chem Eng J. 2021;418:129323.
Jo SB, Erdenebileg U, Dashnyam K, Jin G-Z, Cha J-R, El-Fiqi A, et al. Nano-graphene oxide/polyurethane nanofibers: mechanically flexible and myogenic stimulating matrix for skeletal tissue engineering. J Tissue Eng. 2020;11:2041731419900424.
Aparicio-Collado J, García-San-Martín N, Molina-Mateo J, Cabanilles CT, Quiles VD, Serrano-Aroca A, et al. Electroactive calcium-alginate/polycaprolactone/reduced graphene oxide nanohybrid hydrogels for skeletal muscle tissue engineering. Colloids Surf B Biointerfaces. 2022;214:112455.
Santhosh M, Choi J-H, Choi J-W. Magnetic-assisted cell alignment within a magnetic nanoparticle-decorated reduced graphene oxide/collagen 3D nanocomposite hydrogel. Nanomaterials. 2019;9(9):1293.
Yan Z, Li K, Shao D, Shen Q, Ding Y, Huang S, et al. Visible-light-responsive reduced graphene oxide/gC 3 N 4/TiO 2 composite nanocoating for photoelectric stimulation of neuronal and osteoblastic differentiation. RSC Adv. 2022;12(15):8878–88.
Jaswal R, Shrestha S, Shrestha BK, Kumar D, Park CH, Kim CS. Nanographene enfolded AuNPs sophisticatedly synchronized polycaprolactone based electrospun nanofibre scaffold for peripheral nerve regeneration. Mater Sci Eng C. 2020;116:111213.
Mousavi A, Mashayekhan S, Baheiraei N, Pourjavadi A. Biohybrid oxidized alginate/myocardial extracellular matrix injectable hydrogels with improved electromechanical properties for cardiac tissue engineering. Int J Biol Macromol. 2021;180:692–708.
Tsui JH, Leonard A, Camp ND, Long JT, Nawas ZY, Chavanachat R, et al. Tunable electroconductive decellularized extracellular matrix hydrogels for engineering human cardiac microphysiological systems. Biomaterials. 2021;272:120764.
Joz Majidi H, Babaei A, Kazemi-Pasarvi S, Arab-Bafrani Z, Amiri M. Tuning polylactic acid scaffolds for tissue engineering purposes by incorporating graphene oxide-chitosan nano-hybrids. Polym Adv Technol. 2021;32(4):1654–66.
Wang Z, Shen H, Song S, Zhang L, Chen W, Dai J, et al. Graphene oxide incorporated PLGA nanofibrous scaffold for solid phase gene delivery into mesenchymal stem cells. J Nanosci Nanotechnol. 2018;18(4):2286–93.
Pan N, Wei Y, Zuo M, Li R, Ren X, Huang T-S. Antibacterial poly (ε-caprolactone) fibrous membranes filled with reduced graphene oxide-silver. Colloids Surf Physicochem Eng Aspects. 2020;603:125186.
Sahu G, Das M, Sethy C, Wazalwar R, Kundu CN, Raichur AM, et al. Ionic liquid-assisted fabrication of poly (vinyl alcohol)/nanosilver/graphene oxide composites and their cytotoxicity/antimicrobial activity. Mater Chem Phys. 2021;266:124524.
Ghorbanzadeh R, Assadian H, Chiniforush N, Parker S, Pourakbari B, Ehsani B, et al. Modulation of virulence in Enterococcus faecalis cells surviving antimicrobial photodynamic inactivation with reduced graphene oxide-curcumin: An ex vivo biofilm model. Photodiagnosis Photodyn Ther. 2020;29:101643.
Ahmad N, Nordin NAHM, Jaafar J, Malek NANN, Ismail AF, Yahya MNF, et al. Eco-friendly method for synthesis of zeolitic imidazolate framework 8 decorated graphene oxide for antibacterial activity enhancement. Particuology. 2020;49:24–32.
Dewangan R, Asthana A, Singh AK, Carabineiro SA. Synthesis, characterization and antibacterial activity of a graphene oxide based NiO and starch composite material. J Dispersion Sci Technol. 2020;43(4):559–571.
Matharu RK, Tabish TA, Trakoolwilaiwan T, Mansfield J, Moger J, Wu T, et al. Microstructure and antibacterial efficacy of graphene oxide nanocomposite fibres. J Colloid Interface Sci. 2020;571:239–52.
Download references
Acknowledgements
This research was supported by the National Research Foundation of Korea (NRF) funded by Ministry of Science and ICT (NRF-2021R1A4A3025206) and by Chung-Ang University Research Scholarship Grants in 2020.
Author information
Naline Bellier and Phornsawat Baipaywad are equally contributed to this work.
Authors and Affiliations
School of Integrative Engineering, Chung-Ang University, Seoul, 06974, Republic of Korea
Naline Bellier, Phornsawat Baipaywad, Naeun Ryu & Hansoo Park
Biomedical Engineering Institute, Chiang Mai University, Chiang Mai, 50200, Thailand
Phornsawat Baipaywad
School of Materials Science and Engineering, Gwangju Institute of Science and Technology, Gwangju, 61005, Republic of Korea
Jae Young Lee
You can also search for this author in PubMed Google Scholar
Contributions
NB and PB collected the information, organized the review, and wrote the manuscript. NR collected the information and organized the review. JYL and HP carefully revised the manuscript. All authors have read and approved the final manuscript.
Corresponding authors
Correspondence to Jae Young Lee or Hansoo Park .
Ethics declarations
Ethics approval and consent to participate, consent for publication, competing interests.
The authors declare that they have no competing interests.
Additional information
Publisher’s note.
Springer Nature remains neutral with regard to jurisdictional claims in published maps and institutional affiliations.
Rights and permissions
Open Access This article is licensed under a Creative Commons Attribution 4.0 International License, which permits use, sharing, adaptation, distribution and reproduction in any medium or format, as long as you give appropriate credit to the original author(s) and the source, provide a link to the Creative Commons licence, and indicate if changes were made. The images or other third party material in this article are included in the article's Creative Commons licence, unless indicated otherwise in a credit line to the material. If material is not included in the article's Creative Commons licence and your intended use is not permitted by statutory regulation or exceeds the permitted use, you will need to obtain permission directly from the copyright holder. To view a copy of this licence, visit http://creativecommons.org/licenses/by/4.0/ . The Creative Commons Public Domain Dedication waiver ( http://creativecommons.org/publicdomain/zero/1.0/ ) applies to the data made available in this article, unless otherwise stated in a credit line to the data.
Reprints and permissions
About this article
Cite this article.
Bellier, N., Baipaywad, P., Ryu, N. et al. Recent biomedical advancements in graphene oxide- and reduced graphene oxide-based nanocomposite nanocarriers. Biomater Res 26 , 65 (2022). https://doi.org/10.1186/s40824-022-00313-2
Download citation
Received : 09 June 2022
Accepted : 30 October 2022
Published : 26 November 2022
DOI : https://doi.org/10.1186/s40824-022-00313-2
Share this article
Anyone you share the following link with will be able to read this content:
Sorry, a shareable link is not currently available for this article.
Provided by the Springer Nature SharedIt content-sharing initiative
- Functionalization
- Nanomedicine
- Drug loading
- Therapeutic biomolecules
Biomaterials Research
ISSN: 2055-7124
- Submission enquiries: Access here and click Contact Us
- General enquiries: [email protected]
share this!
April 8, 2024
This article has been reviewed according to Science X's editorial process and policies . Editors have highlighted the following attributes while ensuring the content's credibility:
fact-checked
peer-reviewed publication
trusted source
Improving sodium ion batteries with mechanically robust nanocellular graphene
by Tohoku University
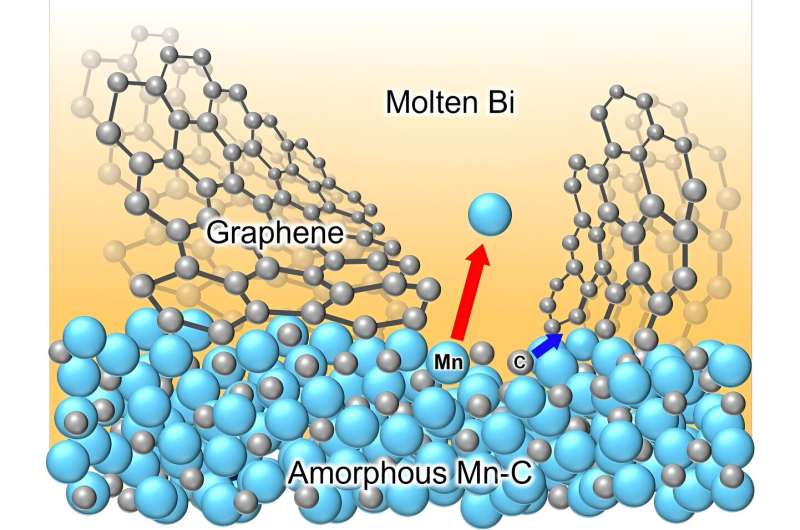
Ever since its discovery in 2004, graphene has been revolutionizing the field of materials science and beyond. Graphene comprises two-dimensional sheets of carbon atoms, bonded into a thin hexagonal shape with a thickness of one atom layer. This gives it remarkable physical and chemical properties.
Despite its thinness, graphene is incredibly strong, lightweight, flexible, and transparent. It also exhibits extraordinary electrical and thermal conductivity , high surface area , and impermeability to gases. From high-speed transistors to biosensors, it boasts an unrivaled versatility in applications.
Nanocellular graphene (NCG) is a specialized form of graphene that achieves a large specific surface area by stacking multiple layers of graphene and controlling its internal structure with a nanoscale cellular morphology.
NCG is coveted for its potential to improve the performance of electronic devices, energy devices and sensors. But its development has been stymied by defects that occur during the manufacturing process . Cracks often appear when forming NCG, and scientists are looking for new processing technologies that can fabricate homogeneous, crack-free and seamless NCGs at appropriate scales.
"We discovered that carbon atoms rapidly self-assemble into crack-free NCG during liquid metal dealloying of an amorphous Mn-C precursor in a molten bismuth," says Won-Young Park, a graduate student at Tohoku University.
The findings are published in the journal Advanced Materials .
Dealloying is a processing technique that exploits the varying miscibility of alloy components in a molten metal bath. This process selectively corrodes certain components of the alloy while preserving others.
Park and his colleagues demonstrated that NCGs developed by this method exhibited high tensile strength and high conductivity after graphitization. Moreover, they put the material to the test in a sodium-ion battery (SIB).
"We used the developed NCG as an active material and current collector in a SIB, where it demonstrated a high rate, long life and excellent deformation resistance. Ultimately, our method of making crack-free NCG will make it possible to raise the performance and flexibility of SIBs—an alternative technology to lithium-ion batteries for certain applications, particularly in large-scale energy storage and stationary power systems where cost, safety, and sustainability considerations are paramount."
Journal information: Advanced Materials
Provided by Tohoku University
Explore further
Feedback to editors

A microbial plastic factory for high-quality green plastic
2 hours ago

Can the bias in algorithms help us see our own?
5 hours ago

Humans have converted at least 250,000 acres of estuaries to cities and farms in last 35 years, study finds

Mysterious bones may have belonged to gigantic ichthyosaurs

Hurricane risk perception drops after storms hit, study shows
6 hours ago

Peter Higgs, who proposed the existence of the 'God particle,' has died at 94

Scientists help link climate change to Madagascar's megadrought
7 hours ago

Heat from El Niño can warm oceans off West Antarctica—and melt floating ice shelves from below
9 hours ago

Peregrine falcons expose lasting harms of flame retardant use

The hidden role of the Milky Way in ancient Egyptian mythology
Relevant physicsforums posts, system curve and centrifugal pump suitability.
Apr 3, 2024
Maximum mass flow in a shell & tube heat exchanger
Air-to-fuel ratio of ethanol.
Mar 27, 2024
Isotopic exchange and SIMS diffusion profile measurement
Mar 20, 2024
Stainless Steel corrosion issue
Mar 5, 2024
H2O2 35% on Al 5083 at 150° gives 'limescale'
Mar 3, 2024
More from Materials and Chemical Engineering
Related Stories

Researchers discover tightest arrangement of bilayer alkali metals between graphene layers
Mar 18, 2024

Laser-scribed graphene for sensors
Oct 5, 2023

Research team introduces new non-toxic method for producing high-quality graphene oxide
Feb 20, 2024

Improving lithium-sulfur batteries with metal-organic framework-based materials
Feb 27, 2024

High-performance magnesium-air primary battery with nitrogen-doped nanoporous graphene as air electrodes
Oct 17, 2023

Researchers synthesize graphene using intense light
Mar 31, 2023
Recommended for you

A targeted polymer to treat colorectal cancer liver metastases
Apr 8, 2024

Utilizing palladium for addressing contact issues of buried oxide thin film transistors
Apr 5, 2024

Propelling atomically layered magnets toward green computers

Unlocking exotic physics: Exploring graphene's topological bands in super-moiré structures
Apr 4, 2024

Biodegradable aerogel: Airy cellulose from a 3D printer

'Neutronic molecules': Study shows neutrons can bind to quantum dots
Let us know if there is a problem with our content.
Use this form if you have come across a typo, inaccuracy or would like to send an edit request for the content on this page. For general inquiries, please use our contact form . For general feedback, use the public comments section below (please adhere to guidelines ).
Please select the most appropriate category to facilitate processing of your request
Thank you for taking time to provide your feedback to the editors.
Your feedback is important to us. However, we do not guarantee individual replies due to the high volume of messages.
E-mail the story
Your email address is used only to let the recipient know who sent the email. Neither your address nor the recipient's address will be used for any other purpose. The information you enter will appear in your e-mail message and is not retained by Phys.org in any form.
Newsletter sign up
Get weekly and/or daily updates delivered to your inbox. You can unsubscribe at any time and we'll never share your details to third parties.
More information Privacy policy
Donate and enjoy an ad-free experience
We keep our content available to everyone. Consider supporting Science X's mission by getting a premium account.
E-mail newsletter
- Open access
- Published: 31 October 2016
Toxicity of graphene-family nanoparticles: a general review of the origins and mechanisms
- Lingling Ou 2 ,
- Bin Song 1 ,
- Huimin Liang 1 ,
- Jia Liu 1 ,
- Xiaoli Feng 1 ,
- Bin Deng 3 ,
- Ting Sun 2 &
- Longquan Shao 1
Particle and Fibre Toxicology volume 13 , Article number: 57 ( 2016 ) Cite this article
536k Accesses
503 Citations
2189 Altmetric
Metrics details
Due to their unique physicochemical properties, graphene-family nanomaterials (GFNs) are widely used in many fields, especially in biomedical applications. Currently, many studies have investigated the biocompatibility and toxicity of GFNs in vivo and in intro. Generally, GFNs may exert different degrees of toxicity in animals or cell models by following with different administration routes and penetrating through physiological barriers, subsequently being distributed in tissues or located in cells, eventually being excreted out of the bodies. This review collects studies on the toxic effects of GFNs in several organs and cell models. We also point out that various factors determine the toxicity of GFNs including the lateral size, surface structure, functionalization, charge, impurities, aggregations, and corona effect ect. In addition, several typical mechanisms underlying GFN toxicity have been revealed, for instance, physical destruction, oxidative stress, DNA damage, inflammatory response, apoptosis, autophagy, and necrosis. In these mechanisms, (toll-like receptors-) TLR-, transforming growth factor β- (TGF-β-) and tumor necrosis factor-alpha (TNF-α) dependent-pathways are involved in the signalling pathway network, and oxidative stress plays a crucial role in these pathways. In this review, we summarize the available information on regulating factors and the mechanisms of GFNs toxicity, and propose some challenges and suggestions for further investigations of GFNs, with the aim of completing the toxicology mechanisms, and providing suggestions to improve the biological safety of GFNs and facilitate their wide application.
Graphene, which is isolated from crystalline graphite, is a flat monolayer composed of single-atom-thick, two-dimensional sheets of a hexagonally arranged honeycomb lattice [ 1 ]. Because of its unique structural, specific surface area and mechanical characteristics, the functions and applications of graphene have gained considerable attention since the discovery of the material in 2004 [ 2 , 3 ]. Graphene and its derivatives include monolayer graphene, few-layer graphene (FLG), graphene oxide (GO), reduced graphene oxide (rGO), graphene nanosheets (GNS), and graphene nanoribbons, etc. [ 4 – 7 ]. GO is one of the most vital chemical graphene derivatives of the graphene-family nanomaterials (GFNs), which attracts increasing attention for its potential biomedical applications. Graphene-based materials usually have sizes ranging from several to hundreds of nanometer and are 1-10 nm thick [ 8 , 9 ], which is also the definition of ‘nanoparticles’ or ‘nanomaterials’. Due to their exceptional physical and chemical properties, graphene materials have been widely used in various fields, including energy storage; nanoelectronic devices; batteries [ 10 – 12 ]; and biomedical applications, such as antibacterials [ 13 , 14 ], biosensors [ 15 – 18 ], cell imaging [ 19 , 20 ], drug delivery [ 8 , 21 , 22 ], and tissue engineering [ 23 – 25 ].
Along with the application and production of GFNs increasing, the risk of unintentional occupational or environmental exposure to GFNs is increasing [ 26 ]. And recently, there are some investigation on GFNs exposure in occupational settings and published data showed that the occupational exposure of GFNs had potential toxicity to the workers and researchers [ 27 – 29 ]. GFNs can be delivered into bodies by intratracheal instillation [ 30 ], oral administration [ 31 ], intravenous injection [ 32 ], intraperitoneal injection [ 33 ] and subcutaneous injection [ 34 ]. GFNs can induce acute and chronic injuries in tissues by penetrating through the blood-air barrier, blood-testis barrier, blood-brain barrier, and blood-placenta barrier etc. and accumulating in the lung, liver, and spleen etc. For example, some graphene nanomaterials aerosols can be inhaled and substantial deposition in the respiratory tract, and they can easily penetrate through the tracheobronchial airways and then transit down to the lower lung airways, resulting in the subsequent formation of granulomas, lung fibrosis and adverse health effects to exposed persons [ 2 , 29 ]. Several reviews have outlined the unique properties [ 35 , 36 ] and summarized the latest potential biological applications of GFNs for drug delivery, gene delivery, biosensors, tissue engineering, and neurosurgery [ 37 – 39 ]; assessed the biocompatibility of GFNs in cells (bacterial, mammalian and plant) [ 7 , 40 , 41 ] and animals (mice and zebrafish) [ 42 ]; collected information on the influence of GFNs in the soil and water environments [ 43 ]. Although these reviews discussed the related safety profiles and nanotoxicology of GFNs, the specific conclusions and detailed mechanisms of toxicity were insufficient, and the mechanisms of toxicity were not summarized completely. The toxicological mechanisms of GFNs demonstrated in recent studies mainly contain inflammatory response, DNA damage, apoptosis, autophagy and necrosis etc., and those mechanisms can be collected to further explore the complex signalling pathways network regulating the toxicity of GFNs. It needs to point out that there are several factors which largely influence the toxicity of GFNs, such as the concentration, lateral dimension, surface structure and functionalization etc. Herein, this review presents a comprehensive summary of the available information on the mechanisms and regulating factors of GFNs toxicity in vitro and in vivo via different experimental methods, with the goals of providing suggestions for further studies of GFNs and completing the toxicology mechanisms to improve the biological safety of GFNs and facilitate their wide application.
Toxicity of GFNs (in vivo and in vitro)
GFNs penetrate through the physiological barriers or cellular structures by different exposure ways or administration routes and entry the body or cells, eventually resulting in toxicity in vivo and in vitro. The varying administration routes and entry paths, different tissue distribution and excretion, even the various cell uptake patterns and locations, may determine the degree of the toxicity of GFNs [ 44 – 46 ]. So to make them clear may be helpful to better understand the laws of the occurrence and development of GFNs toxicity.
Administration route
The common administration routes in animal models include airway exposure (intranasal insufflation, intratracheal instillation, and inhalation), oral administration, intravenous injection, intraperitoneal injection and subcutaneous injection. The major exposure route for GFNs in the working environment is airway exposure, thus inhalation and intratracheal instillation are used mostly in mice to simulate human exposure to GFNs. Though the inhalation method provides the most realistic simulation to real life exposure, instillation is more effective and time-saving method, and GFNs was found that causing longer inflammation period using instillation (intratracheal instillation, intrapleural installation and pharyngeal aspiration) than inhalation [ 24 , 30 , 47 , 48 ]. GFNs were investigated to deposit in the lungs and accumulate to a high level, which retained for more than 3 months in the lungs with slow clearing after intratracheal instillation [ 49 ]. Intravenous injection is also widely used to assess the toxicity of graphene nanomaterials, and graphene circulates through the body of mice in 30 min, accumulating at a working concentration in the liver and bladder [ 32 , 50 – 52 ]. However, GO derivatives had rather finite intestinal adsorption and were rapidly excreted in adult mice via oral administration [ 31 , 53 ]. Nano-sized GO (350 nm) caused less mononuclear cells to infiltrate subcutaneous adipose tissue after subcutaneous injection in the neck region compared to micron-sized GO (2 μm) [ 34 ]. GO agglomerated near the injection site after intraperitoneal injection, and numerous smaller aggregates settled in the proximity of the liver and spleen serosa [ 31 , 33 ]. Experiments on skin contact with or skin permeation of GFNs were not found in the papers reviewed here, and there is insufficient evidence available to conclude that graphene can penetrate intact skin or skin lesions. The route of nasal drops, which has been widely used to test the neurotoxicity or brain injury potential of other nanomaterials, was not mentioned in the papers reviewed here.
GFNs entry paths
GFNs reach various locations through blood circulation or biological barriers after entering the body, which results in varying degrees of retention in different organs. Due to their nanosize, GFNs can reach deeper organs by passing through the normal physiological barriers, such as the blood-air barrier, blood-testis barrier, blood-brain barrier and blood-placental barrier.
Blood-air barrier
The lungs are a potential entrance for graphene nanoparticles into the human body through airway. The inhaled GO nanosheets can destroy the ultrastructure and biophysical properties of pulmonary surfactant (PS) film, which is the first line of host defense, and emerge their potential toxicity [ 54 ]. The agglomerated or dispersed particles deposit on the inner alveolar surface within the alveoli and then be engulfed by alveolar macrophages (AMs) [ 55 ]. Clearance in the lungs is facilitated by the mucociliary escalator, AMs, or epithelial layer [ 56 – 58 ]. However, some small, inhaled nanoparticles infiltrate the intact lung epithelial barrier and can then transiently enter the alveolar epithelium or the interstitium [ 59 , 60 ]. Intratracheally instilled graphene can redistribute to the liver and spleen by passing through the air-blood barrier [ 61 ]. The study of blood-air barrier may draw an intensive attention, since the researchers and workers occupational exposure of GFNs usually through inhalation. To make clear how the blood-air barrier plays a role in the toxicity of GFNs may become a research hot topic.
Blood-brain barrier
The intricate arrangement of the blood-brain barrier, consisting of numbers of membrane receptors and highly selective carriers, only exerts subtle influence on blood circulation and the brain microenvironment compared to the peripheral vascular endothelium [ 62 ]. The research on the mechanism of blood-brain barrier had made some progress involved in diseases and nanotoxicity. Matrix-assisted laser desorption/ionization (MALDI) mass spectrometry imaging (MSI) revealed that rGO, with an average diameter of 342 ± 23.5 nm, permeated through the paracellular pathway into the inter-endothelial cleft in a time-dependent manner by decreasing the blood-brain barrier paracellular tightness [ 63 ]. In addition, graphene quantum dots (GQDs), with a small size of less than 100 nm, can cross through the blood-brain barrier [ 64 ]. Studies on how graphene materials pass through the blood-brain barrier and cause neurotoxicity are very rare, and more data are needed to draw a conclusion.
Blood-testis barrier
The blood-testis and blood-epididymis barriers are well known for being some of the tightest blood-tissue barriers in the mammalian body [ 65 ]. GO particles with diameters of 54.9 ± 23.1 nm had difficulty penetrating the blood-testis and blood-epididymis barriers after intra-abdominal injection, and the sperm quality of the mice was not obviously affected even at 300 mg/kg dosage [ 66 ].
Blood-placenta barrier
The placental barrier is indispensable in maintaining pregnancy, as it mediates the exchange of nutrients and metabolic waste products, exerts vital metabolic functions and secretes hormones [ 67 ]. A recent review suggested that the placenta does not provide a tight barrier against the transfer of nanoparticles to foetuses, specifically against the distribution of carbonaceous nanoparticles to and in the foetus [ 42 ]. It was suggested that rGO and gold particles (diameter of 13 nm) are barely present or are absent in the placenta and foetus in late gestation after intravenous injection [ 44 , 68 ]. However, other reports showed that transplacental transfer does occur in late gestational stages [ 69 , 70 ]. Much attention had been paid to the developmental toxicity of nanomaterials, and reports showed that many nanoparticles did cross the placental barrier and strongly influenced the development of embryos [ 71 – 75 ]. But studies of the exposure to graphene materials through the placenta barrier are deficient, and how these particles transfer to embryos should be evaluated in detail in the future.
These four barriers were the most frequently mentioned barriers in the literature, and other barriers have not been evaluated in recent studies, such as skin barriers, which have not been mentioned in any of the hundreds of GFNs toxicity studies searched. Moreover, the mechanism by which GFNs pass through these barriers is not well understood, and more systematic investigations are urgently needed.
Distribution and excretion of GFNs in tissue
The absorption, distribution, and excretion of graphene nanoparticles may be affected by various factors including the administration routes, physicochemical properties, particle agglomeration and surface coating of GFNs.
The different administration routes influence the distribution of GFNs, for example, intratracheally instilled FLG passing through the air-blood barrier mainly accumulated and was retained in the lungs, with 47 % remaining after 4 weeks [ 61 ]. Intravenously administered GO entered the body through blood circulation and was highly retained in the lung, liver, spleen and bone marrow, and inflammatory cell infiltration, granuloma formation and pulmonary edema were observed in the lungs of mice after intravenous injection of 10 mg kg/body weight GO [ 49 ]. Similarly, high accumulation of PEGylated GO derivatives was observed in the reticuloendothelial (RES) system including liver and spleen after intraperitoneal injection. In contrast, GO-PEG and FLG did not show detectable gastrointestinal tract absorption or tissue uptake via oral administration [ 31 ].
The different properties of GFNs, such as their size, dose and functional groups, always lead to inconsistent results in the distribution profiles of graphene. For instance, Zhang et al. found that GO was mainly entrapped in mouse lungs [ 49 ]; however, Li et al. observed that GO accumulated in mouse liver [ 76 ]. Notably, small GO sheets, with diameters of 10–30 nm, were mainly distributed in the liver and spleen, whereas larger GO sheets (10–800 nm) mainly accumulated in the lungs [ 49 , 52 , 77 ]. If the size of GO is larger than the size of the vessels, GO usually becomes stuck in the arteries and capillaries in the proximity of the injection site. The accumulation of GO in the lungs was shown to increase with an increase in the injected dose and size, but that in the liver significantly decreased [ 78 ]. Coating biocompatible polymers onto GO also affects the biodistribution, for instance, the intravenous injection of GO-PEG and GO-dextran (GO-DEX) accumulate in the reticuloendothelial system (RES), including the liver and spleen, without short-term toxicity [ 31 , 79 ]. Moreover, the charge of plasma proteins and adsorption of GO by plasma proteins also affects the biodistribution [ 34 ].
The excretion and clearance of GFNs vary in different organs. In the lungs, observations indicated that NGO is drawn into and cleared by AMs, which might be eliminated from the sputum through mucociliary clearance or other ways [ 57 ], and 46.2 % of the intratracheally instilled FLG was excreted through the faeces 28 d after exposure [ 61 ]. In the liver, nanoparticles can be eliminated thorough the hepato-biliary pathway following the biliary duct into the duodenum [ 80 ]. In addition, PEGylated GNS that mainly accumulates in the liver and spleen can be gradually cleared, likely by both renal and faecal excretion. As recently reviewed, GO sheets larger than 200 nm are trapped by splenic physical filtration, but small sizes (approximately 8 nm) can penetrate the renal tubules into the urine and be rapidly removed without obvious toxicity [ 81 ]. The excretion paths of GFNs have not yet been clearly explained, but renal and faecal routes appear to be the main elimination routes for graphene.
Recently, the distribution and excretion/toxicity strategy has become an important part of nano-toxicological studies. To date, several controversial results regarding the distribution and excretion of graphene in vivo have been reported in several papers, and a systematic evaluation of the toxicokinetics of GFNs is still needed. The metabolism and excretion of nanomaterials are long-period processes, however, the recent studies of GFNs had been limited to short-term toxicological assessments, and the long-term accumulation and toxicity of GFNs on different tissues remain unknown. Therefore, long-term studies on the deposition and excretion of GFNs need to be performed using different cells and animals to ensure the materials’ biosafety before utilization in human biomedical applications.
Uptake and location of GFNs in cells
The uptake and location of GFNs have also been observed to exert different effects in different cell lines. Graphene is taken up into cells via various routes [ 82 , 83 ]. Basically, the physicochemical parameters such as the size, shape, coating, charge, hydrodynamic diameter, isoelectric point, and pH gradient are important to allow GO to pass through the cell membrane [ 84 ]. As stated previously, nanoparticles with diameters <100 nm can enter cells, and those with diameters <40 nm can enter the nucleus [ 85 ]. For example, GQDs possibly penetrate cell membranes directly, rather than through energy-dependent pathways [ 86 , 87 ]. Larger protein-coated graphene oxide nanoparticles (PCGO) (~1 μm) enter cells mainly through phagocytosis, and smaller PCGO nanoparticles (~500 nm) enter cells primarily through clathrin-mediated endocytosis [ 88 ]. GO sheets could adhere and wrap around the cell membrane, insert in the lipid bilayer or be internalized into the cell as a consequence of interactions with cells [ 89 ]. Similarly, PEGylated reduced graphene oxide (PrGO) and rGO were shown to adhere onto the lipid bilayer cell membrane prominently due to the interaction of hydrophobic, unmodified graphitic domains with the cell membrane [ 90 , 91 ]. Consequently, it was suggested that prolonged exposure to or a high concentration of graphene induces physical or biological damage to the cell membrane, along with destabilization of actin filaments and the cytoskeleton [ 92 ].
Current data demonstrates that GO sheets interact with the plasma membrane and are phagocytosed by macrophages. Three major receptors on macrophages take part in the phagocytosis of GNS: the Fcg receptor (FcgR), mannose receptor (MR), and complement receptor (CR). Furthermore, FcgR is a key receptor in the mediated phagocytic pathway [ 90 , 93 , 94 ]. The protein corona of GO promotes the recognition by macrophage receptors, especially the IgG contained within the protein corona. Macrophages were observed to undergo prodigious morphological changes upon contact with GO [ 34 ]. After internalization, graphene accumulated in the cell cytoplasm, perinuclear space, and nucleus, which induced cytotoxicity in murine macrophages by increasing intracellular ROS through depletion of the mitochondrial membrane potential and by triggering apoptosis through activation of the mitochondrial pathway [ 83 ]. The possible interactions and accumulation sites of GFNs are summarized in Fig. 1 .
Graphene materials and their biological interactions. ( A ) A parameter space for the most widely used graphene materials can be described by the dimensions and surface functionalization of the material, the latter defined as the percentage of the carbon atoms in sp3 hybridization. Green squares represent epitaxially grown graphene; yellow , mechanically exfoliated graphene; red , chemically exfoliated graphene; blue , graphene oxide. Note that a number of other graphene-related materials (such as graphene quantum dots and graphene nanoribbons) are also being used in experiments. ( B ) Possible interactions between graphene-related materials with cells (the graphene flakes are not to scale). ( a ) Adhesion onto the outer surface of the cell membrane. ( b ) Incorporation in between the monolayers of the plasma membrane lipid bilayer. ( c ) Translocation of membrane. ( d ) Cytoplasmic internalization. ( e ) Clathrin-mediated endocytosis. ( f ) Endosomal or phagosomal internalization. ( g ) Lysosomal or other perinuclear compartment localization. ( h ) Exosomal localization. The biological outcomes from such interactions can be considered to be either adverse or beneficial, depending on the context of the particular biomedical application. Different graphene-related materials will have different preferential mechanisms of interaction with cells and tissues that largely await discovery. [ 90 ] Copyright (2014), with permission from American Association for Advancement of Science
Toxicity of GFNs in organs
The toxicity and biocompatibility of GFNs has been observed and assessed through theoretical and animal model studies. At present, there are a mass of data demonstrating the toxicity of GFNs in different organs or systems in animals, so that it is hard to list all the data in this review. Thus we summarized a certain number literature and chose some in vivo toxicological studies of GFNs listed in Table 1 .
Toxicity in internal organs
GO can result in acute inflammation response and chronic injury by interfering with the normal physiological functions of important organs [ 32 , 81 ]. Oral gavage experiments did not show detectable absorption of GO through the gastrointestinal tract [ 95 ]. Interesting, a low dose of GO caused serious damage to the gastrointestinal tract after maternal mice drank a GO suspension rather than a high-dose of GO because a low dose of GO without agglomeration can easily attach to the gastrointestinal surface and cause destruction through its abundant sharp edges [ 53 ]. GFNs caused inflammation and remained in the lung on day 90 after a single intratracheal instillation, and even translocated to lung lymph nodes by a nose-only inhalation [ 96 , 97 ]. A high dose of GO that forms aggregations can block pulmonary blood vessels and result in dyspnea [ 50 , 98 ], and platelet thrombi were observed at high concentrations of 1 and 2 mg/kg body weight via intravenous injection [ 89 ]. GO reportedly disrupted the alveolar-capillary barrier, allowing inflammatory cells to infiltrate into the lungs and stimulate the release of pro-inflammatory cytokines [ 99 ]. Fibrosis and inflammation could be verified by the increased levels of the protein markers collagen1, Gr1, CD68 and CD11b in the lungs. The use of Tween 80 to disperse FLG or a pluronic surfactant to disperse graphene was suggested to reduce the likelihood of lung fibrosis formation in cells or mice, whereas lung fibrosis was observed when graphene was suspended with bovine serum albumin (BSA) [ 100 ]. In addition, radioactive isotopes can be delivered into the lungs, accompanied by a depth distribution of 125 I-NGO in the lungs, and the isotopes might deposit there and result in mutations and cancers [ 30 ]. However, recent publications claimed no obvious pathological changes in mice exposed to low dosages of GO and functionalized graphene by intravenous injection, including aminated GO (GO-NH2), poly(acrylamide)-functionalized GO (GO-PAM), poly(acrylic acid)-functionalized GO (GO-PAA) and GO-PEG; only GO-PEG and GO-PAA induced less toxicity than pristine GO in vivo [ 31 , 79 , 89 ]. So the functional groups of GFNs and the working concentration or aggregate state largely influence the toxicity of GFNs. Recently, the ways to modify the functional group of GFNs, decrease the working concentration or change the aggregate condition are usually used to decrease the toxicity of GFNs.
Toxicity in the central nervous system
Graphene has largely benefited neurosurgery with the application of drug/gene delivery for brain tumour treatment, intracranial and spinal biocompatible devices, biosensing and bioimaging techniques. Studies regarding the potentialities or risks of graphene in the brain have emerged. In the chicken embryo model, pristine graphene flakes decreased the ribonucleic acid level and the rate of deoxyribonucleic acid synthesis, leading to harmful effects on brain tissue development and the atypical ultrastructure was observed in the brain [ 101 ]. The recent researches of GFNs in the central nervous system are mostly involved in the application rather than the toxicity. The data of the toxic study on GFNs is underway.
Toxicity in reproduction and development system
Pristine graphene reduced the vascularization of the heart and the density of branched vessels after injection into fertilized chicken eggs followed by incubation for 19 d [ 101 ]. GO and rGO damage zebrafish embryos by influencing the embryo hatching rate and body length in a concentration-dependent manner. Although no obvious malformation or mortality was observed in exposed zebrafish embryos [ 102 ], GO adhered to and was wrapped in the chorion of the zebrafish embryos, causing remarkable hypoxia and hatching delay. GO aggregates were retained in many organelles, such as the eyes, heart, yolk sac, and tail of the embryos, and apoptosis and reactive oxygen species (ROS) generation were observed in these regions [ 103 ].
The GFNs exert different toxicological effects on male or female reproductive system. Data showed that GO exerted very low or nearly no toxic effects on male reproduction even at a high dose via intra-abdominal injection [ 66 ]. Additionally, rGO did not change the serum estrogen levels of non-pregnant female mice. The condition is different in the female mouse: mouse dams could give birth to healthy offspring after rGO injection before mating or during early gestation, and only a few abnormal foetuses were present among the rGO-injected dam litters. However, the pregnant mice had abortions at all dose, and most pregnant mice died when the high dose of rGO was injected during late gestation [ 44 ]. Notably, the development of offspring in the high dosage group was delayed during the lactation period. The high dose of GO decreased the maternal mice’s water consumption by oral exposure, which reduced milk production and thus postponed the growth of offspring [ 53 ]. Though the findings indicate that GFNs are potentially harmful to development, but data on reproductive and developmental toxicity are still deficient. Studies of the influence of GFNs on male and female reproduction and development are still required to elucidate the underlying toxicity mechanism.
Influence of haemocompatibility
GO release into the blood is ineluctable. The haemocompatibility of GO was found to be dependent on the functional coating and the exposure conditions. GO with submicron size resulted in the greatest haemolytic activity, while aggregated graphene induced the lowest haemolytic reaction. Pristine graphene and GO demonstrated haemolytic effect up to 75 μg/mL [ 104 ]. GO-polyethylenimine (GO-PEI) exhibited notable toxicity by binding to HSA, even at 1.6 μg/mL [ 105 ]. Carboxylated graphene oxide (GO-COOH) showed significant cytotoxicity toward T lymphocytes at concentrations above 50 μg/mL and had good biocompatibility below 25 μg/mL, whereas GO-chitosan nearly inhibited haemolytic activity [ 106 ]. Until now, the corresponding risk of haemocompatibility has remained largely unknown.
In conclusion, the lung injury induced by GFNs has been studied in several studies, the results of which have demonstrated inflammatory cell infiltration, pulmonary edema and granuloma formation in the lungs. However, only a few specific studies have evaluated in other organs, such as the liver, spleen, and kidney, and the injury symptoms, damage index and level of damage to these internal organs were not fully investigated. Moreover, studies on the neurotoxicity of GFNs are quite rare; no data has revealed which nerves or brain areas experience damage, nor have the related behavioural manifestations been studied. The developmental toxicity of GFNs may induce structural abnormalities, growth retardation, behavioural and functional abnormalities, and even death. A study on the reproductive and developmental toxicity of GFNs will be extremely significant and gain extensive attention in the future. Almost all the GFNs toxicity studies were short-period experiments, and no studies have investigated long-term chronic toxic injury. However, based on studies of other nanomaterials toxicity, long-term GFNs exposure may be an important factor harming health [ 107 – 109 ]. Therefore, the long-term study of GFNs is necessary.
Toxicity of GFNs in cell models
The cytotoxicity of GFNs in vitro has been verified in various cells to change the cell viability and morphology, destroy the membrane integrity, and induce DNA damage [ 110 – 112 ]. GO or rGO decrease cell adhesion; induce cell apoptosis; and enter lysosomes, mitochondria, cell nuclei, and endoplasm [ 113 ]. GQDs entered cells and induced DNA damage by the increased expression of p53, Rad 51, and OGG1 proteins in NIH-3 T3 cells [ 87 ]. However, GQDs did not pose significant toxicity to human breast cancer cell lines (at a dose of 50 μg/mL) or human neural stem cells (at a dose of 250 μg/mL) [ 114 , 115 ]. GO derivatives dramatically decreased the expression of differential genes that are responsible for the structure and function of the cell membrane, such as regulation of the actin cytoskeleton, focal adhesion and endocytosis [ 89 ]. In rat pheochromocytoma cells (PC12 cells), graphene and rGO caused cytotoxic effects and mitochondrial injury, such as the release of lactate dehydrogenase (LDH), an increase in the activation of caspase-3, and the generation of ROS [ 82 , 116 ].
Graphene can increase cell viability [ 117 ] or cause cell death [ 118 ] depending on the cell line, type of graphene material and the doseage. GO cytotoxicity was observed in human fibroblasts and lung epithelial cells at concentrations above 20 μg/mL after 24 h, but minimal toxicity was found in A549 cells at concentrations higher than 50 μg/mL [ 119 ]. The biological responses induced by GO such as ROS, malondialdehyde (MDA), and LDH increased, whereas superoxide dismutase (SOD) decreased dose-dependently in HeLa cells [ 120 ]. However, GO-molecular beacon (GO-MB) showed low cytotoxicity even at 20 μg/mL in HeLa cells [ 121 ]. GO decreased the viability of A549 cells, while the same concentration and time of exposure increased the cell viability of CaCo2 colorectal carcinoma cells [ 122 ]. Another study reported that GO dramatically enhanced the differentiation of SH-SY5Y, accompanied by increasing neurite length and the expression of neuronal marker MAP2 at low concentrations but that GO suppressed the viability of SH-SY5Y cells at high doses (≥80 mg/mL) [ 123 ]. Functionalized coatings on GO, such as GO-PEG [ 124 ] and GO-chitosan [ 125 ], can profoundly attenuate the particles’ cytotoxicity by inhibiting the interactions between cells.
The toxicity of GFNs in vitro is summarized in Table 2 . Data on the cytotoxicity of graphene nanomaterials are contrasting, and varying characteristics influence the results. The mechanisms and influencing factors of toxicity need to be elucidated in detail.
Origins of GFNs toxicity
Reportedly, the characteristics of graphene, including its concentration, lateral dimension, surface structure, functional groups, purity and protein corona, strongly influence its toxicity in biological systems [ 2 , 7 , 104 , 126 – 129 ].
Concentration
Numerous results have shown that graphene materials cause dose-dependent toxicity in animals and cells, such as liver and kidney injury, lung granuloma formation, decreased cell viability and cell apoptosis [ 130 – 134 ]. In vivo studies, GO did not exhibit obvious toxicity in mice exposed to a low dose (0.1 mg) and middle dose (0.25 mg) but induced chronic toxicity at a high dose (0.4 mg). The high content of GO mainly deposited in the lungs, liver, spleen, and kidneys and was difficult to be cleaned by the kidneys via a single tail vein injection [ 135 ]. Intriguingly, increasing the dose resulted in a dramatic decrease in the hepatic uptake but an increase in the pulmonary uptake of s-GO by intravenous injection [ 31 ], because the high dose of GO potentially surpassed the uptake saturation or depleted the mass of plasma opsonins, which consequently suppressed the hepatic uptake. Moreover, an in vitro study reported that 20 μg/mL GO nanosheets exhibited no cytotoxicity in A549 within 2 h of incubation, but a higher concentration (85 μg/mL) decreased the cell viability to 50 % within 24 h [ 136 , 137 ]. Lü et al. also demonstrated that GO had no obvious cytotoxicity at low concentrations for 96 h in a human neuroblastoma SH-SY5Y cell line, but the viability of cells sharply decreased to 20 % after treatment with 100 mg/mL GO for 96 h of incubation [ 123 ]. The results in HeLa cells, NIH-3 T3 cells, and breast cancer cells (SKBR3, MCF7) treated with graphene nanoribbons also showed a dose- (10–400 mg/ml) and time-dependent (12–48 h) decrease in cell viability [ 138 ]. Increasing concentrations of GO entered the lysosomes, mitochondria, endoplasm, and cell nucleus [ 119 ]. Several data indicated that rGO caused apoptosis-mediated cell death at a lower dose and early time point but that necrosis was prevalent with the increase in time/dose [ 110 , 135 ].
Lateral dimension
Nanoparticles with sizes <100 nm can enter the cell, <40 nm can enter nucleus, and smaller than <35 nm can cross the blood brain barrier [ 85 ]. One study showed that GO (588, 556, 148 nm) did not enter A549 cells and had no obvious cytotoxicity [ 112 ]. When the diameter of graphene is between 100 ~ 500 nm, the smallest size may cause the most severe toxicity, and when the diameter is below 40 nm, the smallest sizes may be the safest. For instance, rGO with a diameter of 11 ± 4 nm could enter into the nucleus of the hMSCs and cause chromosomal aberrations and DNA fragmentation at very low concentrations of 0.1 and 1.0 mg/mL in 1 h. However, rGO sheets with diameters of 3.8 ± 0.4 nm exhibited no notable genotoxicity in hMSCs even at a high dose of 100 mg/mL after 24 h [ 118 ].
In an in vivo study, s-GO (100–500 nm) preferentially accumulated in the liver, whereas l-GO (1–5 μm) was mainly located in the lungs because l-GO formed larger GO-protein complexes that were filtered out by the pulmonary capillary vessels after intravenously injection [ 31 ]. Given the relative lateral sizes (205.8 nm, 146.8 nm and 33.78 nm) of the three GO nanosheets at the same concentration, smaller GO experiences much greater uptake than larger GO in Hela cells [ 139 ]. The high uptake of s-GO changed in the microenvironment of cells and consequently induced the greatest viability loss and most serious oxidative stress among three sizes of GO samples [ 119 ]. As a result, one study delineated that GO size-dependently induced the M1 polarization of macrophages and pro-inflammatory responses in vitro and in vivo. Larger GO showed stronger adsorption onto the plasma membrane with less phagocytosis, eliciting robust interactions with TLRs and activating NF-κB pathways, compared to smaller GO sheets, which were more likely taken up by cells [ 94 ]. To further uncover the detailed mechanism underlying these effects, more studies are needed to illustrate the vital mechanism of the lateral size of graphene materials.
Surface structure
GFNs possess widely varying surface chemistries. For example, the pristine graphene surface is hydrophobic, GO surface is partially hydrophobic with carboxylate groups [ 140 – 142 ], and rGO has intermediate hydrophilicity [ 143 ]. GFNs were observed to disrupt the function and structure of cell membranes and proteins probably by exceptionally strong molecular interactions with cells [ 2 , 91 ]. For instance, rGO bonded to cell membranes, stimulated receptors and activated mitochondrial pathways, inducing apoptosis [ 110 , 111 , 144 ]. Limited evidence showed that GO is smaller and less toxic than rGO because of the high oxygen content, smoother edges, and hydrophilic properties of the former species [ 104 , 145 , 146 ]. Because of the different surface oxidation states of GO and rGO, GO possessing distinct hydrophilicity might be internalized and taken up by HepG2 cells easily. On the contrary, rGO with evident hydrophobicity, could be adsorbed and aggregated at cell surfaces without (or with lower) uptake [ 110 ]. Due to strong π-π stacking interactions, graphene is highly capability of breaking many residues of the protein, particularly the aromatic ones, such as the villin headpiece (HP), F10, W23, and F35. The protein’s secondary and tertiary structures are largely lying on the graphene surface, disrupting the structure and function of the protein [ 41 ] (Fig. 2 ). In addition, GO can insert between the base pairs of double-stranded DNA and disturb the flow of genetic information at the molecular level, which might be one of the main causes of the mutagenic effect of GO [ 7 , 112 , 146 , 147 ].
A representative trajectory of HP35 adsorbing onto the graphene. ( a ) Representative snapshots at various time points. The proteins are shown in cartoons with red helix and green loop, and the graphene is shown in wheat. The aromatic residues that form the π-π stacking interactions are shown in blue, others are shown in green. ( b ) The contacting surface area of HP35 with the graphene. ( c ) The RMSD of HP35 from its native structure and the number of residues in the α-helix structure. Here, the secondary structures are determined by the DSSP program. ( d ) The distance between the graphene and the aromatic residues, including F35, W23, F10, F17, and F06. To show the adsorbing process clearer, the χ-axis had been truncated and rescaled. [ 41 ] Copyright (2011), with permission from Journal of Physical of Chemistry
A number of studies have highlighted the importance of the GO surface charge because of its ability to affect the internalization and uptake mechanism of cells [ 148 – 150 ]. GO internalization was negligible in non-phagocytes, which was likely due to the strong electrostatic repulsion between the negatively charged GO and the cell surface [ 34 ]. However, others have suggested that negatively charged nanoparticles can be internalized into non-phagocytic cells by binding to available cationic sites on the cell surface and be taken up by scavenger receptors [ 110 , 146 , 150 ]. GO/GS particles reportedly cause morphological changes and significant lysis, leading to high haemolysis in red blood cells (RBCs). RBC membrane disruption is probably attributed to the strong electrostatic interactions between the negatively charged oxygen groups on the GO/GS surface and positively charged phosphatidylcholine lipids on the RBC outer membrane [ 106 ].
Functionalization
Studies confirmed that functionalization with PEG [ 52 ], PEGylated poly-L-lysine (PLL) [ 151 ], poly(ε-caprolactone) [ 152 ], polyvinyl alcohol [ 3 ], Pluronic [ 153 ], amine [ 98 ], carboxyl, and dextran [ 79 ] groups largely decreases the toxicity and improves the biocompatibility of graphene. In vivo results revealed that only mild chronic inflammation emerged after the subcutaneous injection of GO-Pluronic hydrogel and no noticeable short-term toxicity was tested after the intravenous injection of GO-DEX [ 79 , 154 ]. PEGylated GS did not induce appreciable toxicity in mice exposed to 20 mg/kg for 3 months, as evaluated by blood biochemistry and histological examinations, and showed relatively low retention in the RES [ 52 , 155 ]. Coating GO with chitosan almost eliminated the haemolytic activity in blood [ 39 ]. Moreover, the PEG coating effectively alleviated GO-induced acute tissue injuries; decreased GO aggregation and retention in the liver, lungs, and spleen; and promoted the clearance of GO [ 81 ], GO-DEX [ 79 ], and fluorinated graphene oxide (FGO) [ 156 ].
In vitro, several cell function assays showed clear evidence that the surface functionalization of pristine graphene or GO was critical for reducing the strong toxicity effects [ 91 ]. PEG-GO, PEI-GO and LA-PEG-GO damaged human lung fibroblast cells less than GO [ 148 ]. PEG-GO exhibited no cytotoxicity toward several cell cultures, such as glioblastoma cells (U87MG), breast cancer cells (MCF-7), human ovarian carcinoma cells (OVCAR-3), colon cancer cells (HCT-116), and lymphoblastoid cells (RAJI), at concentrations up to 100 μg/mL [ 119 , 157 , 158 ]. GQDs-PEG exhibited very low or no toxicity against lung and cervical cancer cells even at very high concentrations (200 μg/mL) [ 159 ]. However, as a non-biodegradable material with great potential for cellular internalization, further investigation is needed to assess the possible long-term adverse effects of functionalized graphene.
Aggregations and sedimentation
Reportedly, nanomaterials have a propensity to form aggregates rather than individual units, particularly under physiological conditions. GS surfaces allowed fewer RBCs attach comparing to GO, and GS had the lower haemolytic activity for more aqueous aggregations formation. In contrast, the fast sedimentation and aggregate formation of GS greatly inhibited the nutrient availability of human skin fibroblast cells that were grown on the bottom of wells [ 106 ]. Therefore, the aggregations and sedimentation of graphene particles exert varying effects on different cells.
Nanomaterial purity is an important consideration because residual, contaminating metals may be responsible for the observed toxicity, rather than the nanomaterial itself, which has resulted in conflicting data on GFNs cytotoxicity [ 35 , 160 ]. Traditionally prepared GO often contains high levels of Mn 2+ and Fe 2+ , which are highly mutagenic to cells. The nonspecific release of these ions from traditionally prepared GO might lead to unusually high levels of cytotoxicity and DNA fracturing [ 39 ]. In particular, Peng et al. [ 161 ] produced high-purity GO containing only 0.025 ppm Mn 2+ and 0.13 ppm Fe 2+ , and Hanene et al. [ 162 ] invented a new method to prepare high-purity, single-layer GO sheets with good aqueous dispersibility and colloidal stability. GO produced by these new methods did not induce significant cytotoxic responses (at exposure doses up to 100 μg/mL) in vitro, and no obvious inflammatory response or granuloma formation (exposure doses up to 50 μg/animal) were observed in vivo. Therefore, the purity of GFNs deserves attention and is a vital step towards the determination of GFNs involved in bioapplications.
Protein corona effect
Because of the high free surface charge, nanomaterials can easily form “coronas” with proteins in biological systems [ 163 , 164 ]. The protein corona is suggested to affect the circulation, distribution, clearance and toxicity of nanoparticles. Several papers reported that GO forms GO-protein coronas with adsorbed plasma proteins in serum and these GO-protein coronas play an important role in deciding the fate of the GO biokinetic behaviour in vivo. Such GO-protein coronas can regulate the adhesion of GO to endothelial and immune cells through both specific and nonspecific interactions [ 165 ]. Basically, immunoglobulin G and complement proteins in the protein corona help to reorganize nanoparticles in immune cells, causing the particles to be engulfed by the RES, and IgG-coated GO was taken up by either specific or nonspecific interactions with cell membrane receptors [ 31 , 165 ]. However, another study found that GO could not adhere to mucosal epithelial cells directly in the intestinal tract after the filial mice drank an aqueous GO solution because abundant proteins in the milk had adsorbed on the surface of the GO and thus inhibited their direct interaction with the mucosal epithelial cells [ 53 ]. Protein corona mitigated the cytotoxicity of GO by limiting its physical interaction with the cell membrane and reducing the cellular morphological damage in HeLa, THP-1 and A549 cells [ 166 – 168 ]. The cytotoxic effect was largely reduced when GO was pre-coated with FBS and incubated with cells; nearly ∼ 90 % survival was observed with 100 μg/mL FBS-coated GO and 100 % survival with 20 μg/mL FBS-coated GO. Similar trends were observed for GO covered by BSA [ 166 , 169 ]. Consistently, additional serum could neutralize the toxicity of pristine GO in J774.A1 cells at a dose of 4 μg/mL, which lead to a decrease in cell number of 52.5 % compared to untreated cells [ 89 ].
After reviewing many studies, it can be concluded that the toxicity of graphene is influenced by multiple factors. Those factors combined to largely change the toxicity of GFNs in many cases. Scientific studies often need the clear identification of cause and effect, which should keep only one factor different at a time, so that the effect of that single factor can be determined. But in some papers, several factors influencing GFNs toxicity were studied at the same time, which led to confused results.
Possible toxicity mechanisms of GFNs
Although some physicochemical properties and the toxicity of GFNs have been well studied by many scholars, the exact mechanisms underlying the toxicity of GFNs remain obscure. A schematic of the main mechanisms of GFNs cytotoxicity is illustrated in Fig. 3 .
Schematic diagram showed the possible mechanisms of GFNs cytotoxicity. GFNs get into cells through different ways, which induce in ROS generation, LDH and MDA increase, and Ca 2+ release. Subsequently, GFNs cause kinds of cell injury, for instance, cell membrane damage, inflammation, DNA damage, mitochondrial disorders, apoptosis or necrosis
Physical destruction
Graphene is a unique nanomaterial compared with other spherical or one-dimensional nanoparticles due to its two-dimensional structure with sp2-carbons. The physical interaction of graphene nanoparticles with cell membranes is one of the major causes of graphene cytotoxicity [ 7 , 170 , 171 ]. Graphene has high capability to bind with the α-helical structures of peptides because of its favourable surface curvature [ 172 ]. At concentration above 75 μg/mL, pristine graphene largely adhered to the surfaces of RAW 264.7 cells and resulted in abnormal stretching of the cell membrane [ 104 ]. The strong hydrophobic interactions of GFNs with the cell membrane lead to the morphological extension of F-actin filopodial and cytoskeletal dysfunction. Furthermore, the sharpened edges of GNS may act as ‘blades’, inserting and cutting through bacterial cell membranes [ 173 ]. Moreover, GO also damaged the outer membrane of E. coli bacteria directly, resulting in the release of intracellular components [ 173 ]. However, TEM imaging revealed that pre-coating GO with FBS eliminated the destruction of cell membranes [ 166 ].
ROS production leading to oxidative stress
Oxidative stress arises when increasing levels of ROS overwhelm the activity of antioxidant enzymes, including catalase, SOD, or glutathione peroxidase (GSH-PX) [ 174 ]. ROS act as second messengers in many intracellular signalling cascades and lead to cellular macromolecular damage, such as membrane lipid breakdown, DNA fragmentation, protein denaturation and mitochondrial dysfunction, which greatly influence cell metabolism and signalling [ 175 – 177 ]. The interactions of GO with cells can lead to excessive ROS generation, which is the first step in the mechanisms of carcinogenesis, ageing, and mutagenesis [ 83 , 122 ]. Oxidative stress had a significant role in GO-induced acute lung injury [ 30 ], and the inflammatory responses caused by oxidative stress often emerged upon exposure to GFNs [ 133 , 177 , 178 ]. The activity of SOD and GSH-PX decreased after exposed to GO in a time- and dosage-dependent manner [ 82 , 106 , 119 ]. Similarly, oxidative stress was the key cause of apoptosis and DNA damage after HLF cells were exposed to GO [ 148 ]. Both the mitogen-activated protein kinase (MAPK) (JNK, ERK and p38) and TGF-beta-related signalling pathways were triggered by ROS generation in pristine graphene-treated cells, accompanied by the activation of Bim and Bax, which are two pro-apoptotic members of the Bcl-2 protein family. As a result, caspase-3 and its downstream effector proteins such as PARP were activated, and apoptosis was initiated [ 83 , 179 ]. Detailed information regarding the MAPK-, TGF-β- and TNF-α-related signalling pathways, which induce inflammation, apoptosis and necrosis, are summarized in Fig. 4 .
Schematic diagram of MAPKs, TGF-beta and TNF-α dependent pathways involved in GFNs toxicity. ROS was the main factors activating the MAPKs and TGF-beta signaling pathways to lead to the activation of Bim and Bax, triggering the cascade of caspases and JNK pathway. The activation of caspase 3 and RIP1 resulted in apoptosis and necrosis finally
Mitochondrial damage
Mitochondria are energy production centres involved in various signalling pathways in cells and are also a key point of apoptotic regulation [ 83 ]. After exposure to GO and carboxyl graphene (GXYG), the mitochondrial membrane was depolarized, and the amount of mitochondria decreased in HepG2 cells [ 180 ]. Exposure to GFNs resulted in significantly increased coupled and uncoupled mitochondrial oxygen consumption, dissipation of the mitochondrial membrane potential, and eventual triggering of apoptosis by activating the mitochondrial pathway [ 181 ]. For instance, GO increased the activity of mitochondrial electron transport complexes I/III and the supply of electrons to site I/II of the electron transport chain, accelerating the generation of ROS during mitochondrial respiration in MHS cells [ 99 ]. The formation of •OH mediated by GO and the cytochrome-c/H 2 O 2 electron-transfer system could enhance oxidative and thermal stress to impair the mitochondrial respiration system and eventually result in dramatic toxicity [ 151 ]. Additionally, the oxygen moieties on GO might accept electrons from cellular redox proteins, supporting the redox cycling of cytochrome c and electron transport proteins, and cytochromes MtrA, MtrB, and MtrC/OmcA might be involved in transferring electrons to GO [ 182 ]. Therefore, except for the plasma membrane damage and oxidative stress induction, GFNs can cause apoptosis and/or cell necrosis by direct influencing cell mitochondrial activity [ 183 , 184 ].
Due to its small size, high surface area and surface charge, GO may possess significant genotoxic properties and cause severe DNA damage, for example, chromosomal fragmentation, DNA strand breakages, point mutations, and oxidative DNA adducts and alterations [ 87 , 122 , 185 , 186 ]. Mutagenesis was observed in mice after intravenous injection of GO at a dose of 20 mg/kg compared with cyclophosphamide (50 mg/kg), a classic mutagen [ 112 ]. Even if GO cannot enter into the nucleus of a cell, it may still interact with DNA during mitosis when the nuclear membrane breaks down, which increases the opportunity for DNA aberrations [ 87 , 147 , 187 , 188 ]. The π stacking interaction between the graphene carbon rings and the hydrophobic DNA base pairs can make a DNA segment ‘stand up’ or ‘lay on’ the surface of graphene with its helical axis perpendicular or parallel, respectively. The intermolecular forces severely deform the end base pairs of DNA, which potentially increases the genotoxicity [ 189 ]. GO may also induce chromosomal fragmentation, DNA adducts and point mutations by promoting oxidative stress or triggering inflammation through the activation of intracellular signalling pathways such as MAPK, TGF-β and NF-κB [ 110 , 112 , 146 ]. Graphene and rGO can also elevate the expression of p53, Rad51, and MOGG1-1, which reflect chromosomal damage, and decrease the expression of CDK2 and CDK4 by arresting the cell cycle transition from the G1 to the S phase in various cell lines [ 112 ]. DNA damage can not only initiate cancer development but also possibly threaten the health of the next generation if the mutagenic potential of GO arises in reproductive cells, which impacts fertility and the health of offspring [ 112 , 190 ].
Inflammatory response
GFNs can cause a significant inflammatory response including inflammatory cell infiltration, pulmonary edema and granuloma formation at high doses via intratracheally instillation or intravenous administration [ 30 , 49 ]. Platelets are the important components in clot formation to attack pathogens and particulate matter during the inflammatory response, and GO could directly activate platelet-rich thrombi formation to occlude lung vessels after intravenous injection [ 98 , 191 ]. A strong inflammatory response was induced by subcutaneously injection with GO for 21 days, along with the secretion of key cytokines, including IL-6, IL-12, TNF-α, MCP-1, and IFN-g [ 34 , 192 ]. GFNs can trigger an inflammatory response and tissue injury by releasing cytokines and chemokines that lead to the recruitment of circulating monocytes and stimulating the secretion of Th1/Th2 cytokines and chemokines [ 124 , 193 ]. Additionally, pristine graphene [ 193 ] and rGO [ 110 ] evoke an inflammatory response by binding to toll-like receptors (TLRs) and activating the NF-κB signalling pathway in cells. The NF-κB signalling cascade is triggered by TLRs and pro-inflammatory cytokines such as IL-1 and TNF-α. Upon activation, NF-κB shifts from the cytoplasm to the nucleus, facilitating the binding of degrading IκB and acting as a transcription factor to synthesize numerous pro-inflammatory cytokines [ 194 ]. A schematic of the signalling pathway of TLR4 and TLR9 activated by GFNs is shown in Fig. 5 .
A schematic diagram elucidating signalling pathway of TLR4 and TLR9 responsible for GFNs-induced cytotoxicity. GFNs can be recognized by TLRs, thus activate IKK and IκB by a MyD88-dependent mechanism, resulting in the release of NF-κB subunits and initiating the translocation into the nucleus. Thus, pro-inflammatory factors were transcribed and secreted out of nucleus, modulating the immune responses initiating programmed autophagy, apoptosis and necrosis
Apoptosis is defined as the self-destruction of a cell regulated by genes through complicated programmes [ 83 , 195 ]. GO and rGO caused apoptosis and inflammation in mice lungs after inhalation [ 99 ], and GFNs also had pro-apoptotic effects in cells [ 111 , 113 , 124 , 196 ]. Additionally, graphene and GO physically damaged cell membranes [ 166 ], increased the permeabilization of the outer mitochondrial membrane and changed the mitochondrial membrane potential; the increased ROS triggered the MAPK and TGF-β signalling pathways and activated caspase-3 via mitochondrial-dependent apoptotic cascades, prompting the execution of apoptosis [ 83 , 99 ]. Similarly, rGO caused apoptosis at a low dose and an early time point, triggered by the death-receptor and canonical mitochondrial pathway [ 110 ]. Another study showed three different apoptosis pathways by GFNs: GO led to ROS-dependent apoptosis through direct interaction with protein receptors and subsequent activation of the B-cell lymphoma-2 (Bcl-2) pathway; GO-COOH transmitted a passive apoptosis signal to nuclear DNA by binding to protein receptors and activating a ROS-independent pathway; However, GO-PEI severely damaged the membranes of T lymphocytes to trigger apoptosis [ 105 , 197 ].
Autophagy is the process of self-degradation of cellular components and recently recognized as non-apoptotic cell death [ 198 – 200 ]. Autophagy activation requires autophagosome formation containing Beclin 1, multiple autophagy-related proteins (ATG), microtubule-associated protein light chain 3 (LC3) and p62 [ 201 ]. Autophagosome accumulation is associated with exposure to various nanoparticles [ 202 – 205 ], and autophagy can remove extracellular organisms and destruct the organisms in the cytosol [ 206 ]. GO and GQDs was shown to induce autophagosome accumulation and the conversion of LC3-I to LC3-II; inhibit the degradation of the autophagic substrate p62 protein [ 207 , 208 ]. Furthermore, GO can simultaneously trigger TLR4 and TLR9 responses in macrophages [ 34 , 192 ] and colon cancer cells CT26 [ 206 ]. The autophagy pathway is linked to phagocytosis by TLR signalling in macrophages [ 206 , 209 ].
Necrosis is an alternate form of cell death induced by inflammatory responses or cellular injury. The exposure of cells to pristine graphene causes apoptosis and necrosis at high doses (50 mg/mL) [ 83 ]. Reportedly, LDH leakage and the opening of the mitochondrial permeability transition pore, induced by elevated level of cytoplasmic Ca 2+ , lead to apoptosis/necrosis [ 210 ]. GO treatment was revealed to induce macrophagic necrosis by activating TLR4 signalling and subsequently partly triggering autocrine TNF-α production [ 93 ]. GO combined with CDDP (GO/CDDP) triggered necrosis by decreasing RIP1 and increasing RIP3 proteins, accompanied with the release of high mobility group B1 (HMGB1) into the cytosol from the nucleus and out of CT26 cells [ 205 , 211 , 212 ].
Epigenetic changes
Epigenetics involve DNA methylation, genomic imprinting, maternal effects, gene silencing, and RNA editing [ 213 – 215 ]. DNA methylation, which is one of the best-studied epigenetic modifications, includes phosphorylation, ubiquitination, and ATP-ribosylation and can lead to chromatin remodelling [ 197 , 216 , 217 ]. A recently paper reported that SL-GO/FL-GO exposure resulted in global DNA hypermethylation through upregulating DNMT3B and MBD1 genes; GNP treatment caused hypomethylation by decreasing the expression of DNMT3B and MBD1 genes [ 216 ]. GO could activate the miRNA-360 regulation pathway to suppress the DNA damage-apoptosis signalling cascade by affecting the component of CEP-1 [ 218 ]. Taken together, these data suggest that GFNs could cause subtle changes in gene expression programming by modulating epigenetic changes. However, studies of GFNs-induced epigenetic changes are few, and the epigenetic mechanism caused by GFNs exposure is not fully understood.
To conclude, many studies have discussed representative mechanisms of GFNs toxicity involving four signalling pathways: TLRs, TGF-β, TNF-α and MAPKs. These four signalling pathways are correlative and cross-modulatory, making the inflammatory response, autophagy, apoptosis and other mechanisms independent and yet connected to each other. Additionally, oxidative stress appears to play the most important role in activating these signalling pathways. It has been reported that there are intersections of apoptosis, autophagy and necrosis in the studies of other nanomaterials toxicity, they inhibit or promote mutually in some conditions. However, the signalling pathways of GFNs toxicity investigated in papers to date are only a small part of an intricate web, and the network of signalling pathways needs to be explored in detail in the future.
Data gaps and future studies
Currently, the literature is insufficient to draw conclusions about the potential hazards of GFNs. Two opposite opinions have begun to emerge: some researchers suggested that graphene materials are biocompatible in a number of studies focused on biomedical applications [ 119 , 154 , 162 , 219 ], and other studies reported adverse biological responses and cytotoxicity [ 32 , 118 , 135 , 138 , 192 ]. These inconsistent results might have been caused by several factors, including the different research groups, various cellular or animal models, and varying physicochemical characterizations of GFNs. When GFNs are explored for in vivo applications in the human body or some other biomedical applications, biocompatibility must be considered, and more detailed and accurate studies of GFNs toxicity are needed.
First, detailed physicochemical characterization is imperative in all future studies of GFNs toxicity. In the experiments, feature descriptions of GFNs should include their size, morphology, surface area, charge, surface modifications, purity, and agglomeration [ 88 , 141 , 148 , 162 ]. Because these physicochemical factors largely influence the toxicity and biocompatibility of GFNs, single-factor experimental designs and the exclusion of other interfering factors should be considered. Details of the fabrication process should also be provided because the formed oxidative debris could largely alter the surface structure of graphene and GO during functionalization [ 151 ]. Importantly, a single, universal method needs to be established in graphene technology, which will allow for better comparison of data from different studies or different laboratories.
Second, different observational criteria, parameters and selection of experimental methods might induce large inter-laboratory variations [ 220 , 221 ]. For example, the MTT assay always fails to accurately predict graphene toxicity because the spontaneous reduction results in a false positive signal. Therefore, appropriate alternative assessments should be utilized, such as the water-soluble tetrazolium salt reagent (WST-8), ROS assay, and trypan blue exclusion test [ 106 , 222 ]. Additionally, the comet assay often shows higher levels of DNA damage than the micronucleus assay because the former measures the repairable injury and the latter measures the gene damage that remains after cell division [ 159 , 223 ]. Therefore, caution is required in choosing the most appropriate assay to evaluate the toxicity of graphene materials to avoid false-positive results.
Third, the selection of cell lines is of vital importance because cancer cell lines tend to be sensitive or resistant depending upon their genetic background. The same graphene nanoparticles can cause different reactions depending on their various cells origins. Suitable cell lines with good stability must be used to avoid false positive or negative results. Primary cells derived from humans or animals can better simulate the health conditions of humans. A large amount of primary cells have been utilized to test the toxicity of other nanomaterials [ 224 – 228 ], but the culturing of primary cells is extremely rare in the experiments with GFNs to date [ 210 , 229 ]. Various cell experiments combined with primary cells should be performed to comprehensively evaluate the physicochemical properties and toxicity of GFNs.
Fourth, the administration route of GFNs plays a very important role in toxicity studies, and different delivery methods will result in different toxicological reactions [ 32 , 53 ]. Thus, the route and period of exposure should be carefully chosen according to the aim of the study. Nasal drug delivery is often used to study the neurotoxicity of nanomaterials [ 230 , 231 ], but this administration method has rarely been applied in the testing of GFNs toxicity. Toxicological studies of GFNs in the nervous system are rare, and the mechanism is unclear and needs to be studied further in the future. Recent toxicokinetic studies involving the absorption, distribution, metabolism, accumulation, and excretion of GFNs through different exposure routes have yielded some results but are far from sufficient to clarify the internal complex mechanisms. For instance, further studies are needed to understand the specific molecular mechanisms of GFNs passing through the physiological barriers and the amount of accumulation or the excretion period of GFNs in tissues. In addition, given the increased exposure of humans to GFNs, the assessment of systemic toxicity in the human body is indispensable in future studies.
Fifth, another important issue requiring attention is the long-term fate of GFNs after entering the body or being taken up by cells. Most recent studies have consisted of short-term toxicity assessments [ 89 , 232 ], and long-term toxic injury has not received much attention since the widespread application of GFNs in 2008. Moreover, a functionalized graphene surface can improve its biocompatibility, but the long-term stability of the surface coatings should be considered [ 233 ]. If the surface coatings eventually break down, their toxicity may be significantly different from the short-term exposure results. Extended studies are needed to determine if longer treatment times influence the nanotoxic potential of GFNs.
Sixth, more specific signalling pathways in the mechanism of GFNs toxicity need to be discovered and elucidated. Currently, several typical toxicity mechanisms of GFNs have been illustrated and widely accepted, such as oxidative stress, apoptosis, and autophagy. However, these mechanisms have only been described in general terms, and the specific signalling pathways within these mechanisms need to be investigated in detail. The signalling pathways involved in the toxicity of other nanomaterials may also be relevant to the study of GFNs. Therefore, more signalling pathways should be detected in future research. For instance, nano-epigenetics has been considered in numerous studies of nanomaterials, which is also helpful in assessing the limited toxicity and side effects of GFNs. Recent studies have shown that GFNs could cause epigenetic and genomic changes that might stimulate physical toxicity and carcinogenicity [ 234 ]. GFNs have high surface areas, smooth continuous surfaces and bio-persistence, similar to the properties of tumorigenic solid-state implants. It is unknown whether GFNs have the potential to induce foreign body sarcomas, and definitive studies of tumour potentialities or risks of graphene should therefore be conducted as soon as possible.
Conclusions
In the past few years, GFNs have been widely utilized in a wide range of technological and biomedical fields. Currently, most experiments have focused on the toxicity of GFNs in the lungs and livers. Therefore, studies of brain injury or neurotoxicity deserve more attention in the future. Many experiments have shown that GFNs have toxic side effects in many biological applications, but the in-depth study of toxicity mechanisms is urgently needed. In addition, contrasting results regarding the toxicity of GFNs need to be addressed by effective experimental methods and systematic studies. This review provides an overview of the toxicity of GFNs by summarizing the toxicokinetics, toxicity mechanisms and influencing factors and aimed to provide information to facilitate thorough research on the in vitro and in vivo haemo- and biocompatibility of GFNs in the future. This review will help address safety concerns before the clinical and therapeutic applications of GFNs, which will be important for further development of GFNs in biological applications.
Abbreviations
Alveolar macrophages
Blood-epididymis barriers
Complement receptor
Fcg receptor
Few-layer graphene
Graphene family nanomaterials
Graphene nanosheets
Graphene oxide
Carboxylated graphene oxide
GO-molecular beacon
Aminated GO
Poly(acrylic acid)-functionalized GO
Poly(acrylamide)-functionalized GO
PEGylated GO derivatives
GO-polyethylenimine
Graphene quantum dots
Glutathione peroxidase
Carboxyl graphene
Lactate and dehydrogenase
Matrix-assisted laser desorption/ionization
Mitogen-activated protein kinase
Malondialdehyde
Mannose receptor
Mass spectrometry imaging
Rat pheochromocytoma cells
Protein-coated graphene oxide nanoparticles
PEGylated reduced graphene oxide
Reticuloendothelial system
Reduced graphene oxide
Reactive oxygen species
Superoxide dismutase
Toll-like receptor
Novoselov KS, Geim AK, Morozov SV, Jiang D, Zhang Y, Dubonos SV, et al. Electric field effect in atomically thin carbon films. Science. 2004;306(5696):666–9.
Article CAS PubMed Google Scholar
Sanchez VC, Jachak A, Hurt RH, Kane AB. Biological interactions of graphene-family nanomaterials: an interdisciplinary review. Chem Res Toxicol. 2012;25(1):15–34.
Yang XY, Wang YS, Huang X, Ma YF, Huang Y, Yang RC, et al. Multi-functionalized graphene oxide based anticancer drug-carrier with dual-targeting function and pH-sensitivity. J Mat Chem. 2011;21(10):3448–54.
Article CAS Google Scholar
Park S, An J, Jung I, Piner RD, An SJ, Li X, et al. Colloidal suspensions of highly reduced graphene oxide in a wide variety of organic solvents. Nano Lett. 2009;9(4):1593–7.
Geim AK. Graphene: status and prospects. Science. 2009;324(5934):1530–4.
Guo X, Mei N. Assessment of the toxic potential of graphene family nanomaterials. J Food Drug Anal. 2014;22(1):105–15.
Seabra AB, Paula AJ, de Lima R, Alves OL, Duran N. Nanotoxicity of graphene and graphene oxide. Chem Res Toxicol. 2014;27(2):159–68.
Shen H, Zhang L, Liu M, Zhang Z. Biomedical applications of graphene. Theranostics. 2012;2(3):283–94.
Article CAS PubMed PubMed Central Google Scholar
Han U, Seo Y, Hong J. Effect of pH on the structure and drug release profiles of layer-by-layer assembled films containing polyelectrolyte, micelles, and graphene oxide. Sci Rep. 2016;6(2045–2322 (Electronic)):24158.
Wang H, Liang Y, Mirfakhrai T, Chen Z, Casalongue HS, Dai H. Advanced asymmetrical supercapacitors based on graphene hybrid materials. Nano Res. 2011;4(8):729–36.
Loh KP, Bao Q, Eda G, Chhowalla M. Graphene oxide as a chemically tunable platform for optical applications. Nat Chem. 2010;2(12):1015–24.
Wang D, Zhu L, Chen JF, Dai L. Mn3O4-graphene hybrid as a high-capacity anode material for lithium ion batteries. J Am Chem Soc. 2015;132(1520–5126 (Electronic)):13978–80.
Google Scholar
Gurunathan S, Han JW, Dayem AA, Eppakayala V, Kim JH. Oxidative stress-mediated antibacterial activity of graphene oxide and reduced graphene oxide in Pseudomonas aeruginosa. Int J Nanomed. 2012;7(1178–2013 (Electronic)):e14.
Zhan S, Zhu D, Ma S, Yu W, Jia Y, Li Y, et al. Highly efficient removal of pathogenic bacteria with magnetic graphene composite. ACS Appl Mater Interf. 2015;7(1944–8252 (Electronic)):4290–8.
Yang HW, Hua MY, Chen SL, Tsai RY. Reusable sensor based on high magnetization carboxyl-modified graphene oxide with intrinsic hydrogen peroxide catalytic activity for hydrogen peroxide and glucose detection. Biosens Bioelectron. 2013;41:172–9.
Wang Y, Yuan R, Chai Y, Yuan Y, Bai L. In situ enzymatic silver enhancement based on functionalized graphene oxide and layer-by-layer assembled gold nanoparticles for ultrasensitive detection of thrombin. Biosens Bioelectron. 2012;38(1):50–4.
Article PubMed CAS Google Scholar
Huang J, Zhang L, Liang RP, Qiu JD. “On-off” switchable electrochemical affinity nanobiosensor based on graphene oxide for ultrasensitive glucose sensing. Biosens Bioelectron. 2013;41:430–5.
Gao L, Lian C, Zhou Y, Yan L, Li Q, Zhang C, et al. Graphene oxide-DNA based sensors. Biosens Bioelectron. 2014;60(1873–4235 (Electronic)):22–9.
Chen ML, Liu JW, Hu B, Chen ML, Wang JH. Conjugation of quantum dots with graphene for fluorescence imaging of live cells. Analyst. 2011;136(20):4277–83.
Wang Y, Wang H, Liu D, Song S, Wang X, Zhang H. Graphene oxide covalently grafted upconversion nanoparticles for combined NIR mediated imaging and photothermal/photodynamic cancer therapy. Biomaterials. 2013;34(1878–5905 (Electronic)):7715–24.
Pan Y, Sahoo NG, Li L. The application of graphene oxide in drug delivery. Expert Opin Drug Deliv. 2012;9(11):1365–76.
Huiyun W, Chunyan D, Haiqing D, Aijun S, Wenjuan X, Xiaojun C, et al. Engineered redox-responsive PEG detachment mechanism in PEGylated nano-graphene oxide for intracellular drug delivery. Small. 2012;8(5):760–9.
Yang X, Qiu L, Cheng C, Wu Y, Ma ZF, Li D. Ordered gelation of chemically converted graphene for next-generation electroconductive hydrogel films. Angewandte Chem Int Ed Engl. 2011;50(32):7325–8.
Schinwald A, Murphy FA, Jones A, Macnee W, Donaldson K. Graphene-based nanoplatelets: a new risk to the respiratory system as a consequence of their unusual aerodynamic properties. ACS Nano. 2012;6(1):736–46.
Chaenyung C, Ryon SS, Xiguang G, Nasim A, Dokmeci MR, Xiaowu Shirley T, et al. Controlling mechanical properties of cell-laden hydrogels by covalent incorporation of graphene oxide. Small. 2014;10(3):514–23.
Arvidsson R, Molander S, Sandén BA. Review of potential environmental and health risks of the nanomaterial graphene. Hum Ecol Risk Assess. 2013;19(4):873–87.
CAS Google Scholar
Lee JH, Han JH, Kim JH, Kim B, Bello D, Kim JK, et al. Exposure monitoring of graphene nanoplatelets manufacturing workplaces. Inhal Toxicol. 2016;28(6):281–91.
Maynard RL. Nano-technology and nano-toxicology. Emerg Health Threats J. 2012;5.
Su WC, Ku BK, Kulkarni P, Cheng YS. Deposition of graphene nanomaterial aerosols in human upper airways. J Occup Environ Hyg. 2015;13(1):1–34.
Li B, Yang J, Huang Q, Zhang Y, Peng C, Zhang Y, et al. Biodistribution and pulmonary toxicity of intratracheally instilled graphene oxide in mice. NPG Asia Mater. 2013;5:E44.
Yang K, Gong H, Shi X, Wan J, Zhang Y, Liu Z. In vivo biodistribution and toxicology of functionalized nano-graphene oxide in mice after oral and intraperitoneal administration. Biomaterials. 2013;34(11):2787–95.
Wen KP, Chen YC, Chuang CH, Chang HY, Lee CY, Tai NH. Accumulation and toxicity of intravenously-injected functionalized graphene oxide in mice. J Appl Toxicol. 2015;35(10):1211–8.
Kurantowicz N, Strojny B, Sawosz E, Jaworski S, Kutwin M, Grodzik M, et al. Biodistribution of a high dose of diamond, graphite, and graphene oxide nanoparticles after multiple intraperitoneal injections in rats. Nanoscale Res Lett. 2015;10(1):398.
Article PubMed PubMed Central CAS Google Scholar
Yue H, Wei W, Yue Z, Wang B, Luo N, Gao Y, et al. The role of the lateral dimension of graphene oxide in the regulation of cellular responses. Biomaterials. 2012;33(16):4013–21.
Nezakati T, Cousins BG, Seifalian AM. Toxicology of chemically modified graphene-based materials for medical application. Arch Toxicol. 2014;88(11):1987–2012.
Chng ELK, Pumera M. Toxicity of graphene related materials and transition metal dichalcogenides. Rsc Advances. 2015;5(4):3074–80.
Zheng XT, Ananthanarayanan A, Luo KQ, Chen P. Glowing graphene quantum dots and carbon dots: properties, syntheses, and biological applications. Small. 2015;11(1613–6829 (Electronic)):1620–36.
Caffo M, Merlo L, Marino D, Caruso G. Graphene in neurosurgery: the beginning of a new era. Nanomed. 2015;10:615–25.
Wu SY, An SS, Hulme J. Current applications of graphene oxide in nanomedicine. Int J Nanomed. 2015;10(Spec Iss):9–24.
Tonelli FMP, Goulart VAM, Gomes KN, Ladeira MS, Santos AK, Lorencon E, et al. Graphene-based nanomaterials: biological and medical applications and toxicity. Nanomedicine. 2015;10(15):2423–50.
Zhou R, Gao H. Cytotoxicity of graphene: recent advances and future perspective. Wiley Interdiscip Rev Nanomed Nanobiotechnol. 2014;6(5):452–74.
Ema M, Hougaard KS, Kishimoto A, Honda K. Reproductive and developmental toxicity of carbon-based nanomaterials: A literature review. Nanotoxicology. 2015;10:391–412.
Jastrzebska AM, Olszyna AR. The ecotoxicity of graphene family materials: current status, knowledge gaps and future needs. J Nanopart Res. 2015;17(1):1–21.
Xu S, Zhang Z, Chu M. Long-term toxicity of reduced graphene oxide nanosheets: Effects on female mouse reproductive ability and offspring development. Biomaterials. 2015;54:188–200.
Jennifer M, Maciej W. Nanoparticle technology as a double-edged sword: cytotoxic, genotoxic and epigenetic effects on living cells. J Biomater Nanobiotechnol. 2013;4:53–63.
Wu W, Yan L, Wu Q, Li Y, Li Q, Chen S, et al. Evaluation of the toxicity of graphene oxide exposure to the eye. Nanotoxicology. 2016;10(9):1329–40.
Lee K, Jeong Y, Bae J, Seok H, Yang Y, Roh S, et al. The role of surface functionalization on the pulmonary inflammogenicity and translocation into mediastinal lymph nodes of graphene nanoplatelets in rats. Arch Toxicol.2016:1–10.
Schinwald A, Murphy F, Askounis A, Koutsos V, Sefiane K, Donaldson K, et al. Minimal oxidation and inflammogenicity of pristine graphene with residence in the lung. Nanotoxicology. 2013;8(8):824–32.
Zhang X, Yin J, Peng C, Hu W, Zhu Z, Li W, et al. Distribution and biocompatibility studies of graphene oxide in mice after intravenous administration. Carbon. 2011;49(3):986–95.
Singh SK, Singh MK, Nayak MK, Kumari S, Shrivastava S, Gracio JJ, et al. Thrombus inducing property of atomically thin graphene oxide sheets. ACS Nano. 2011;5(6):4987–96.
Gurunathan S, Han JW, Eppakayala V, Kim JH. Biocompatibility of microbially reduced graphene oxide in primary mouse embryonic fibroblast cells. Colloids Surf B Biointerf. 2013;105:58–66.
Yang K, Wan J, Zhang S, Zhang Y, Lee ST, Liu Z. In vivo pharmacokinetics, long-term biodistribution, and toxicology of PEGylated graphene in mice. ACS Nano. 2011;5(1):516–22.
Fu C, Liu T, Li L, Liu H, Liang Q, Meng X. Effects of graphene oxide on the development of offspring mice in lactation period. Biomaterials. 2015;40:23–31.
Hu Q, Jiao B, Shi X, Valle RP, Zuo YY, Hu G. Effects of graphene oxide nanosheets on the ultrastructure and biophysical properties of the pulmonary surfactant film. Nanoscale. 2015;7(43):18025–9.
Gosens I, Post JA, de la Fonteyne LJ, Jansen EH, Geus JW, Cassee FR, et al. Impact of agglomeration state of nano- and submicron sized gold particles on pulmonary inflammation. Part Fibre Toxicol. 2010;7(1743–8977 (Electronic)):1.
Geiser M, Kreyling WG. Deposition and biokinetics of inhaled nanoparticles. Part Fibre Toxicol. 2010;7:2.
Ruge CA, Schaefer UF, Herrmann J, Kirch J, Canadas O, Echaide M, et al. The interplay of lung surfactant proteins and lipids assimilates the macrophage clearance of nanoparticles. PLoS One. 2012;7(7):e40775.
Morfeld P, Treumann S, Ma-Hock L, Bruch J, Landsiedel R. Deposition behavior of inhaled nanostructured TiO2 in rats: fractions of particle diameter below 100 nm (nanoscale) and the slicing bias of transmission electron microscopy. Inhal Toxicol. 2012;24(1091–7691 (Electronic)):939–51.
Wiemann M, Vennemann A, Sauer UG, Wiench K, Ma-Hock L, Landsiedel R. An in vitro alveolar macrophage assay for predicting the short-term inhalation toxicity of nanomaterials. J Nanobiotechnol. 2016;14(1477–3155 (Electronic)):1.
Kreyling WG, Semmler-Behnke M, Takenaka S, Möller W. Differences in the biokinetics of inhaled nano- versus micrometer-sized particles. Accounts Chem Res. 2012;46(1520–4898 (Electronic)):714–22.
Liang M, Hu M, Pan B, Xie Y, Petersen EJ. Biodistribution and toxicity of radio-labeled few layer graphene in mice after intratracheal instillation. Part Fibre Toxicol. 2016;13(1):1–12.
Abbott NJ, Patabendige AA, Dolman DE, Yusof SR, Begley DJ. Structure and function of the blood–brain barrier. Neurobiol Dis. 2010;37(1):13–25.
Mendonca MC, Soares ES, de Jesus MB, Ceragioli HJ, Ferreira MS, Catharino RR, et al. Reduced graphene oxide induces transient blood–brain barrier opening: an in vivo study. J Nanobiotechnol. 2015;13:78.
Article Google Scholar
Liu Y, Xu LP, Dai W, Dong H, Wen Y, Zhang X. Graphene quantum dots for the inhibition of beta amyloid aggregation. Nanoscale. 2015;7(45):19060–5.
Mital P, Hinton BT, Dufour JM. The blood-testis and blood-epididymis barriers are more than just their tight junctions. Biol Reprod. 2011;84(5):851–8.
Liang S, Xu S, Zhang D, He J, Chu M. Reproductive toxicity of nanoscale graphene oxide in male mice. Nanotoxicology. 2015;9(1):92–105.
Buerkithurnherr T, Von MU, Wick P. Knocking at the door of the unborn child: engineered nanoparticles at the human placental barrier. Swiss Med Wkly. 2012;142:w13559.
Yang H, Sun C, Fan Z, Tian X, Yan L, Du L, et al. Effects of gestational age and surface modification on materno-fetal transfer of nanoparticles in murine pregnancy. Sci Rep. 2012;2(46):847.
PubMed PubMed Central Google Scholar
Huang X, Zhang F, Sun X, Choi KY, Niu G, Zhang G, et al. The genotype-dependent influence of functionalized multiwalled carbon nanotubes on fetal development. Biomaterials. 2014;35(2):856–65.
Qi W, Bi J, Zhang X, Wang J, Wang J, Liu P, et al. Damaging effects of multi-walled carbon nanotubes on pregnant mice with different pregnancy times. Sci Rep. 2014;4(3):doi: 10.1038/srep04352.
Du J, Wang S, You H, Jiang R, Zhuang C, Zhang X. Developmental toxicity and DNA damage to zebrafish induced by perfluorooctane sulfonate in the presence of ZnO nanoparticles. Environ Toxicol. 2014;31(1522–7278 (Electronic)):360–71.
PubMed Google Scholar
Zhou Z, Son J, Harper B, Zhou Z, Harper S. Influence of surface chemical properties on the toxicity of engineered zinc oxide nanoparticles to embryonic zebrafish. Beilstein J Nanotechnol. 2015;6(2190–4286 (Electronic)):1568–79.
Rollerova E, Tulinska J, Liskova A, Kuricova M, Kovriznych J, Mlynarcikova A, et al. Titanium dioxide nanoparticles: some aspects of toxicity/focus on the development. Endocr Reg. 2014;49(1210–0668 (Print)):97–112.
Warheit DB, Boatman R, Brown SC. Developmental toxicity studies with 6 forms of titanium dioxide test materials (3 pigment-different grade & 3 nanoscale) demonstrate an absence of effects in orally-exposed rats. Reg Toxicol Pharmacol. 2015;73(1096–0295 (Electronic)):887–96.
Ema M, Gamo M, Honda K. Developmental toxicity of engineered nanomaterials in rodents. Toxicol Appl Pharmacol. 2015;299(1096–0333 (Electronic)):47–52.
Li Z, Geng Y, Zhang X, Qi W, Fan Q, Li Y, et al. Biodistribution of co-exposure to multi-walled carbon nanotubes and graphene oxide nanoplatelets radiotracers. J Nanopart Res. 2011;13(7):2939–47.
Wang Y, Li Z, Hu D, Lin CT, Li J, Lin Y. Aptamer/graphene oxide nanocomplex for in situ molecular probing in living cells. J Am Chem Soc. 2010;132(27):9274–6.
Liu JH, Yang ST, Wang H, Chang Y, Cao A, Liu Y. Effect of size and dose on the biodistribution of graphene oxide in mice. Nanomedicine. 2012;7(12):1801–12.
Zhang S, Yang K, Feng L, Liu Z. In vitro and in vivo behaviors of dextran functionalized graphene. Carbon. 2011;49(12):4040–9.
Hirn S, Semmler-Behnke M, Schleh C, Wenk A, Lipka J, Schaffler M, et al. Particle size-dependent and surface charge-dependent biodistribution of gold nanoparticles after intravenous administration. Eur J Pharm Biopharm. 2011;77(3):407–16.
Li B, Zhang XY, Yang JZ, Zhang YJ, Li WX, Fan CH, et al. Influence of polyethylene glycol coating on biodistribution and toxicity of nanoscale graphene oxide in mice after intravenous injection. Int J Nanomedicine. 2014;9:4697–707.
Zhang Y, Ali SF, Dervishi E, Xu Y, Li Z, Casciano D, et al. Cytotoxicity effects of graphene and single-wall carbon nanotubes in neural phaeochromocytoma-derived PC12 cells. ACS Nano. 2010;4(6):3181–6.
Li Y, Liu Y, Fu Y, Wei T, Le Guyader L, Gao G, et al. The triggering of apoptosis in macrophages by pristine graphene through the MAPK and TGF-beta signaling pathways. Biomaterials. 2012;33(2):402–11.
Sydlik SA, Jhunjhunwala S, Webber MJ, Anderson DG, Langer R. In vivo compatibility of graphene oxide with differing oxidation states. ACS Nano. 2015;9(4):3866–74.
Mytych J, Wnuk M. Nanoparticle technology as a double-edged sword: cytotoxic, genotoxic and epigenetic effects on living cells. J Biomater Nanobiotechnol. 2013;4:53–63.
Peng C, Hu W, Zhou Y, Fan C, Huang Q. Intracellular imaging with a graphene-based fluorescent probe. Small. 2010;6(15):1686–92.
Wang D, Zhu L, Chen JF, Dai L. Can graphene quantum dots cause DNA damage in cells? Nanoscale. 2015;7(21):9894–901.
Mu Q, Su G, Li L, Gilbertson BO, Yu LH, Zhang Q, et al. Size-dependent cell uptake of protein-coated graphene oxide nanosheets. ACS Appl Mater Interf. 2012;4(4):2259–66.
Xu M, Zhu J, Wang F, Xiong Y, Wu Y, Wang Q, et al. Improved in vitro and in vivo biocompatibility of graphene oxide through surface modification: poly(acrylic acid)-functionalization is superior to PEGylation. ACS Nano. 2016;10:3267–81.
Kostarelos K, Novoselov KS. Materials science. Exploring the interface of graphene and biology. Science. 2014;344(6181):261–3.
Sasidharan A, Panchakarla LS, Chandran P, Menon D, Nair S, Rao CN, et al. Differential nano-bio interactions and toxicity effects of pristine versus functionalized graphene. Nanoscale. 2011;3(6):2461–4.
Li Y, Yuan H, von dem Bussche A, Creighton M, Hurt RH, Kane AB, et al. Graphene microsheets enter cells through spontaneous membrane penetration at edge asperities and corner site. Proc Natl Acad Sci U S A. 2013;110(1091–6490 (Electronic)):12295–300.
Qu G, Liu S, Zhang S, Wang L, Wang X, Sun B, et al. Graphene oxide induces toll-like receptor 4 (TLR4)-dependent necrosis in macrophages. ACS Nano. 2013;7(7):5732–45.
Ma J, Liu R, Wang X, Liu Q, Chen Y, Valle RP, et al. Crucial role of lateral size for graphene oxide in activating macrophages and stimulating Pro-inflammatory responses in cells and animals. ACS Nano. 2015;9(10):10498–515.
Mao L, Hu M, Pan B, Xie Y, Petersen EJ. Biodistribution and toxicity of radio-labeled few layer graphene in mice after intratracheal instillation. Part Fibre Toxicol. 2016;13(1743–8977 (Electronic)):1.
Park EJ, Lee SJ, Lee K, Choi YC, Lee BS, Lee GH, et al. Pulmonary persistence of graphene nanoplatelets may disturb physiological and immunological homeostasis. J Appl Toxicol. 2016.
Kim JK, Shin JH, Lee JS, Hwang JH, Lee JH, Baek JE, et al. 28-Day inhalation toxicity of graphene nanoplatelets in Sprague–Dawley rats. Nanotoxicology. 2016;10(7):891–901.
Singh SK, Singh MK, Kulkarni PP, Sonkar VK, Gracio JJ, Dash D. Amine-modified graphene: thrombo-protective safer alternative to graphene oxide for biomedical applications. ACS Nano. 2012;6(3):2731–40.
Duch MC, Budinger GR, Liang YT, Soberanes S, Urich D, Chiarella SE, et al. Minimizing oxidation and stable nanoscale dispersion improves the biocompatibility of graphene in the lung. Nano Lett. 2011;11(12):5201–7.
Wang X, Duch MC, Mansukhani N, Ji Z, Liao YP, Wang M, et al. Use of a pro-fibrogenic mechanism-based predictive toxicological approach for tiered testing and decision analysis of carbonaceous nanomaterials. ACS Nano. 2015;9(1936-086X (Electronic)):3032–43.
Sawosz E, Jaworski S, Kutwin M, Hotowy A, Wierzbicki M, Grodzik M, et al. Toxicity of pristine graphene in experiments in a chicken embryo model. Int J Nanomed. 2014;9:3913–22.
Liu XT, Mu XY, Wu XL, Meng LX, Guan WB, Ma YQ, et al. Toxicity of multi-walled carbon nanotubes, graphene oxide, and reduced graphene oxide to zebrafish embryos. Biomed Environ Sci. 2014;27(9):676–83.
Chen Y, Hu X, Sun J, Zhou Q. Specific nanotoxicity of graphene oxide during zebrafish embryogenesis. Nanotoxicology. 2016;10(1):42–52.
CAS PubMed Google Scholar
Sasidharan A, Panchakarla LS, Sadanandan AR, Ashokan A, Chandran P, Girish CM, et al. Hemocompatibility and macrophage response of pristine and functionalized graphene. Smal. 2012;8(8):1251–63.
Ding Z, Zhang Z, Ma H, Chen Y. In vitro hemocompatibility and toxic mechanism of graphene oxide on human peripheral blood T lymphocytes and serum albumin. ACS Appl Mater Interf. 2014;6(22):19797–807.
Liao KH, Lin YS, Macosko CW, Haynes CL. Cytotoxicity of graphene oxide and graphene in human erythrocytes and skin fibroblasts. ACS Appl Mater Interfaces. 2011;3(7):2607–15.
Kouhi SMM, Lahouti M, Ganjeali A, Entezari MH. Long-term exposure of rapeseed (Brassica napus L.) to ZnO nanoparticles: anatomical and ultrastructural responses. Environ Sci Pollut Res. 2015;22(1614–7499 (Electronic)):10733–43.
Vales G, Rubio L, Marcos R. Long-term exposures to low doses of titanium dioxide nanoparticles induce cell transformation, but not genotoxic damage in BEAS-2B cells. Nanotoxicology. 2015;9(1743–5404 (Electronic)):568–78.
Sancey L, Kotb S, Truillet C, Appaix F, Marais A, Thomas E, et al. Long-term in vivo clearance of gadolinium-based AGuIX nanoparticles and their biocompatibility after systemic injection. ACS Nano. 2015;9(1936-086X (Electronic)):2477–88.
Chatterjee N, Eom HJ, Choi J. A systems toxicology approach to the surface functionality control of graphene-cell interactions. Biomaterials. 2014;35:1109–27.
Jaworski S, Sawosz E, Grodzik M, Winnicka A, Prasek M, Wierzbicki M, et al. In vitro evaluation of the effects of graphene platelets on glioblastoma multiforme cells. Int J Nanomed. 2013;8:413–20.
Liu Y, Luo Y, Wu J, Wang Y, Yang X, Yang R, et al. Graphene oxide can induce in vitro and in vivo mutagenesis. Sci Rep. 2013;3:3469.
Vallabani NV, Mittal S, Shukla RK, Pandey AK, Dhakate SR, Pasricha R, et al. Toxicity of graphene in normal human lung cells (BEAS-2B). J Biomed Nanotechnol. 2011;7(1):106–7.
Peng J, Gao W, Gupta BK, Liu Z, Romero-Aburto R, Ge L, et al. Graphene quantum dots derived from carbon fibers. Nano Lett. 2012;12(1530–6992 (Electronic)):844–9.
Shang W, Zhang X, Zhang M, Fan Z, Sun Y, Han M, et al. The uptake mechanism and biocompatibility of graphene quantum dots with human neural stem cells. Nanoscale. 2014;6(2040–3372 (Electronic)):5799–806.
Zhang L, Xia J, Zhao Q, Liu L, Zhang Z. Functional graphene oxide as a nanocarrier for controlled loading and targeted delivery of mixed anticancer drugs. Small. 2010;6(4):537–44.
Ruiz ON, Fernando KA, Wang B, Brown NA, Luo PG, McNamara ND, et al. Graphene oxide: a nonspecific enhancer of cellular growth. ACS Nano. 2011;5(10):8100–7.
Akhavan O, Ghaderi E, Akhavan A. Size-dependent genotoxicity of graphene nanoplatelets in human stem cells. Biomaterials. 2012;33(32):8017–25.
Chang Y, Yang ST, Liu JH, Dong E, Wang Y, Cao A, et al. In vitro toxicity evaluation of graphene oxide on A549 cells. Toxicol Lett. 2011;200(3):201–10.
Zhang X, Hu W, Li J, Tao L, Wei Y. A comparative study of cellular uptake and cytotoxicity of multi-walled carbon nanotubes, graphene oxide, and nanodiamond. Toxicol Res. 2012;1(1):62–8.
Lu CH, Zhu CL, Li J, Liu JJ, Chen X, Yang HH. Using graphene to protect DNA from cleavage during cellular delivery. Chem Commun. 2010;46(1364-548X (Electronic)):3116–8.
De Marzi L, Ottaviano L, Perrozzi F, Nardone M, Santucci S, De Lapuente J, et al. Flake size-dependent cyto and genotoxic evaluation of graphene oxide on in vitro A549, CaCo2 and vero cell lines. J Biol Regul Homeost Agents. 2014;28(2):281–9.
Lv M, Zhang Y, Liang L, Wei M, Hu W, Li X, et al. Effect of graphene oxide on undifferentiated and retinoic acid-differentiated SH-SY5Y cells line. Nanoscale. 2012;4(13):3861–6.
Reshma SC, Syama S, Mohanan PV. Nano-biointeractions of PEGylated and bare reduced graphene oxide on lung alveolar epithelial cells: A comparative in vitro study. Colloids Surf B Biointerf. 2016;140(1873–4367 (Electronic)):104–16.
Rana VK, Choi MC, Kong JY, Kim GY, Mi JK, Kim SH, et al. Synthesis and drug‐delivery behavior of chitosan‐functionalized graphene oxide hybrid nanosheets. Macromol Mater Eng. 2011;296(2):131–40.
Yang K, Li Y, Tan X, Peng R, Liu Z. Behavior and toxicity of graphene and its functionalized derivatives in biological systems. Small. 2013;9(9–10):1492–503.
Yoon OJ, Kim I, Sohn IY, Kieu TT, Lee NE. Toxicity of graphene nanoflakes evaluated by cell-based electrochemical impedance biosensing. J Biomed Mater Res A. 2014;102(7):2288–94.
Jastrzebska AM, Kurtycz P, Olszyna AR. Recent advances in graphene family materials toxicity investigations. J Nanopart Res. 2012;14(12):1320.
Misra SK, Kondaiah P, Bhattacharya S, Rao CN. Graphene as a nanocarrier for tamoxifen induces apoptosis in transformed cancer cell lines of different origins. Small. 2012;8(1):131–43.
Singh Z. Applications and toxicity of graphene family nanomaterials and their composites. Nanotechnol Sci Appl. 2016;9(1177–8903 (Electronic)):15.
Article PubMed PubMed Central Google Scholar
Combarros RG, Collado S, Diaz M. Toxicity of graphene oxide on growth and metabolism of Pseudomonas putida. J Hazard Mater. 2016;310(1873–3336 (Electronic)):246–52.
Lee JK, Jeong AY, Bae J, Seok JH, Yang JY, Roh HS, et al. The role of surface functionalization on the pulmonary inflammogenicity and translocation into mediastinal lymph nodes of graphene nanoplatelets in rats. Arch Toxicol. 2016(1432–0738 (Electronic)):1–10. DOI: .1007/s00204-016-1706-y
Patlolla AK, Randolph J, Kumari SA, Tchounwou PB. Toxicity evaluation of graphene oxidein kidneys of Sprague–Dawley rats. Int J Environ Res Public Health. 2016;13(1660–4601 (Electronic)):380.
Wang ZG, Zhou R, Jiang D, Song JE, Xu Q, Si J, et al. Toxicity of graphene quantum dots in zebrafish embryo. Biomed Environ Sci. 2015;28(0895–3988 (Print)):341–51.
Wang K, Jing R, Song H, Zhang J, Yan W, Guo S, et al. Biocompatibility of graphene oxide. Nanoscale Res Lett. 2010;6(1):1–8.
Hu W, Peng C, Luo W, Lv M, Li X, Li D, et al. Graphene-based antibacterial paper. ACS Nano. 2010;4(7):4317–23.
Dreyer DR, Park S, Bielawski CW, Ruoff RS. The chemistry of graphene oxide. Chem Soc Rev. 2010;39(1):228–40.
Mullick Chowdhury S, Lalwani G, Zhang K, Yang JY, Neville K, Sitharaman B. Cell specific cytotoxicity and uptake of graphene nanoribbons. Biomaterials. 2013;34(1):283–93.
Zhang H, Peng C, Yang J, Lv M, Liu R, He D, et al. Uniform ultrasmall graphene oxide nanosheets with low cytotoxicity and high cellular uptake. ACS Appl Mater Interf. 2013;5(5):1761–7.
Hasan SA, Rigueur JL, Harl RR, Krejci AJ, Isabel GJ, Rogers BR, et al. Transferable graphene oxide films with tunable microstructures. ACS Nano. 2010;4(12):7367–72.
Hsieh CT, Chen WY. Water/oil repellency and work of adhesion of liquid droplets on graphene oxide and graphene surfaces. Surf Coat Technol. 2011;205(19):4554–61.
Yang ST, Chang Y, Wang H, Liu G, Sheng C, Wang Y, et al. Folding/aggregation of graphene oxide and its application in Cu 2+ removal. J Colloid Interf Sci. 2010;351(1):122–7.
Bagri A, Mattevi C, Acik M, Chabal YJ, Chhowalla M, Shenoy VB. Structural evolution during the reduction of chemically derived graphene oxide. Nat Chem. 2010;2(7):581–7.
Hinzmann M, Jaworski S, Kutwin M, Jagiello J, Kozinski R, Wierzbicki M, et al. Nanoparticles containing allotropes of carbon have genotoxic effects on glioblastoma multiforme cells. Int J Nanomed. 2014;9:2409–17.
Jin C, Wang F, Tang Y, Zhang X, Wang J, Yang Y. Distribution of graphene oxide and TiO2-graphene oxide composite in A549 cells. Biol Trace Elem Res. 2014;159(1–3):393–8.
Jarosz A, Skoda M, Dudek I, Szukiewicz D. Oxidative stress and mitochondrial activation as the main mechanisms underlying graphene toxicity against human cancer cells. Oxid Med Cell Longev. 2016;2016:5851035.
Article PubMed Google Scholar
Ren H, Wang C, Zhang J, Zhou X, Xu D, Zheng J, et al. DNA cleavage system of nanosized graphene oxide sheets and copper ions. ACS Nano. 2010;4(12):7169–74.
Wang A, Pu K, Dong B, Liu Y, Zhang L, Zhang Z, et al. Role of surface charge and oxidative stress in cytotoxicity and genotoxicity of graphene oxide towards human lung fibroblast cells. J Appl Toxicol. 2013;33(10):1156–64.
Jiang X, Dausend J, Hafner M, Musyanovych A, Rocker C, Landfester K, et al. Specific effects of surface amines on polystyrene nanoparticles in their interactions with mesenchymal stem cells. Biomacromolecules. 2010;11(3):748–53.
Yue ZG, Wei W, Lv PP, Yue H, Wang LY, Su ZG, et al. Surface charge affects cellular uptake and intracellular trafficking of chitosan-based nanoparticles. Biomacromolecules. 2011;12(7):2440–6.
Zhang W, Wang C, Li Z, Lu Z, Li Y, Yin JJ, et al. Unraveling stress-induced toxicity properties of graphene oxide and the underlying mechanism. Adv Mater. 2012;24(39):5391–7.
Wojtoniszak M, Chen X, Kalenczuk RJ, Wajda A, Łapczuk J, Kurzewski M, et al. Synthesis, dispersion, and cytocompatibility of graphene oxide and reduced graphene oxide. Colloids Surf B Biointerf. 2011;89(1):79–85.
Hu H, Yu J, Li Y, Zhao J, Dong H. Engineering of a novel pluronic F127/graphene nanohybrid for pH responsive drug delivery. J Biomed Mater Res A. 2012;100(1):141–8.
Sahu A, Choi WI, Tae G. A stimuli-sensitive injectable graphene oxide composite hydrogel. Chem Commun (Camb). 2012;48(47):5820–2.
Yang K, Zhang S, Zhang G, Sun X, Lee ST, Liu Z. Graphene in mice: ultrahigh in vivo tumor uptake and efficient photothermal therapy. Nano Lett. 2010;10(9):3318–23.
Romero-Aburto R, Narayanan TN, Nagaoka Y, Hasumura T, Mitcham TM, Fukuda T, et al. Fluorinated graphene oxide; a new multimodal material for biological applications. Adv Mater. 2013;25(39):5632–7.
Feng L, Liu Z. Graphene in biomedicine: opportunities and challenges. Nanomed (Lond). 2011;6(2):317–24.
Robinson JT, Tabakman SM, Liang Y, Wang H, Casalongue HS, Vinh D, et al. Ultrasmall reduced graphene oxide with high near-infrared absorbance for photothermal therapy. J Am Chem Soc. 2011;133(17):6825–31.
Singh N, Manshian B, Jenkins GJS, Griffiths SM, Williams PM, Maffeis TGG, et al. NanoGenotoxicology: The DNA damaging potential of engineered nanomaterials. Biomaterials. 2009;30(s 23–24):3891–914.
Yin PT, Shah S, Chhowalla M, Lee KB. Design, synthesis, and characterization of graphene-nanoparticle hybrid materials for bioapplications. Chem Rev. 2015;115(7):2483–531.
Peng L, Xu Z, Liu Z, Wei Y, Sun H, Li Z, et al. An iron-based green approach to 1-h production of single-layer graphene oxide. Nat Commun. 2015;6:5716.
Ali-Boucetta H, Bitounis D, Raveendran-Nair R, Servant A, Van den Bossche J, Kostarelos K. Purified graphene oxide dispersions lack in vitro cytotoxicity and in vivo pathogenicity. Adv Healthc Mater. 2013;2(3):433–41.
Dell’Orco D, Lundqvist M, Oslakovic C, Cedervall T, Linse S. Modeling the time evolution of the nanoparticle-protein corona in a body fluid. PLoS One. 2010;5(6):e10949-e.
Eudald C, Tobias P, Albert D, Gertie Janneke O, Victor P. Time evolution of the nanoparticle protein corona. ACS Nano. 2010;4(7):3623–32.
Aggarwal P, Hall JB, McLeland CB, Dobrovolskaia MA, McNeil SE. Nanoparticle interaction with plasma proteins as it relates to particle biodistribution, biocompatibility and therapeutic efficacy. Adv Drug Deliv Rev. 2009;61(6):428–37.
Hu W, Peng C, Lv M, Li X, Zhang Y, Chen N, et al. Protein corona-mediated mitigation of cytotoxicity of graphene oxide. ACS Nano. 2011;5(5):3693–700.
Duan G, Kang SG, Tian X, Garate JA, Zhao L, Ge C, et al. Protein corona mitigates the cytotoxicity of graphene oxide by reducing its physical interaction with cell membrane. Nanoscale. 2015;7:15214–24.
Cuicui G, Jiangfeng D, Lina Z, Liming W, Ying L, Denghua L, et al. Binding of blood proteins to carbon nanotubes reduces cytotoxicity. Proc Natl Acad Sci U S A. 2011;108(41):16968–73.
Li Y, Feng L, Shi X, Wang X, Yang Y, Yang K, et al. Surface coating-dependent cytotoxicity and degradation of graphene derivatives: towards the design of non-toxic, degradable nano-graphene. Small. 2014;10(8):1544–54.
Gurunathan S, Han J, Park JH, Kim JH. An in vitro evaluation of graphene oxide reduced by Ganoderma spp. in human breast cancer cells (MDA-MB-231). Int J Nanomed. 2014;9:1783–97.
Yuan J, Gao H, Ching CB. Comparative protein profile of human hepatoma HepG2 cells treated with graphene and single-walled carbon nanotubes: an iTRAQ-coupled 2D LC-MS/MS proteome analysis. Toxicol Lett. 2011;207(3):213–21.
Tomasio SM, Walsh TR. Modeling the binding affinity of peptides for graphitic surfaces. Influences of aromatic content and interfacial shape. J Phys Chem C. 2009;113(20):8778–85.
Akhavan O, Ghaderi E. Toxicity of graphene and graphene oxide nanowalls against bacteria. ACS Nano. 2010;4(10):5731–6.
Burton GJ, Jauniaux E. Oxidative stress. Best Pract Res Clin Obstet Gynaecol. 2011;25:287–99.
Waiwijit U, Kandhavivorn W, Oonkhanond B, Lomas T, Phokaratkul D, Wisitsoraat A, et al. Cytotoxicity assessment of MDA-MB-231 breast cancer cells on screen-printed graphene-carbon paste substrate. Colloids Surf B Biointerf. 2014;113:190–7.
Chong Y, Ma Y, Shen H, Tu X, Zhou X, Xu J, et al. The in vitro and in vivo toxicity of graphene quantum dots. Biomaterials. 2014;35(19):5041–8.
Chen M, Yin J, Liang Y, Yuan S, Wang F, Song M, et al. Oxidative stress and immunotoxicity induced by graphene oxide in zebrafish. Aqua Toxicol. 2016;174(1879–1514 (Electronic)):54–60.
Meng C, Zhi X, Li C, Li C, Chen Z, Qiu X, et al. Graphene oxides decorated with carnosine as an adjuvant to modulate innate immune and improve adaptive immunity in vivo. ACS Nano. 2016;10(1936-086X (Electronic)):2203–13.
Ravichandran P, Baluchamy S, Sadanandan B, Gopikrishnan R, Biradar S, Ramesh V, et al. Multiwalled carbon nanotubes activate NF-κB and AP-1 signaling pathways to induce apoptosis in rat lung epithelial cells. Apoptosis. 2010;15(12):1507–16.
Lammel T, Boisseaux P, Fernandez-Cruz ML, Navas JM. Internalization and cytotoxicity of graphene oxide and carboxyl graphene nanoplatelets in the human hepatocellular carcinoma cell line Hep G2. Part Fibre Toxicol. 2013;10:27.
Gurunathan S, Han JW, Eppakayala V, Kim JH. Green synthesis of graphene and its cytotoxic effects in human breast cancer cells. Int J Nanomedicine. 2013;8:1015–27.
Salas EC, Sun Z, Luttge A, Tour JM. Reduction of graphene oxide via bacterial respiration. ACS Nano. 2010;4(8):4852–6.
Shekaramiz E. Immobilization of mitochondria on graphene. Dissert Theses Gradworks. 2012;217(1):120–31.
Park EJ, Lee GH, Han BS, Lee BS, Lee S, Cho MH, et al. Toxic response of graphene nanoplatelets in vivo and in vitro. Arch Toxicol. 2015;89(9):1557–68.
Chatterjee N, Yang J, Choi J. Differential genotoxic and epigenotoxic effects of graphene family nanomaterials (GFNs) in human bronchial epithelial cells. Mutat Res Gen Tox En. 2016;798(1879–3592 (Electronic)):1–10.
Ivask A, Voelcker NH, Seabrook SA, Hor M, Kirby JK, Fenech M, et al. DNA melting and genotoxicity induced by silver nanoparticles and graphene. Chem Res Toxicol. 2015;28(1520–5010 (Electronic)):1023–35.
Magdolenova Z, Collins A, Kumar A, Dhawan A, Stone V, Dusinska M. Mechanisms of genotoxicity. A review of in vitro and in vivo studies with engineered nanoparticles. Nanotoxicology. 2014;8(3):233–78.
Golbamaki N, Rasulev B, Cassano A, Marchese Robinson RL, Benfenati E, Leszczynski J, et al. Genotoxicity of metal oxide nanomaterials: review of recent data and discussion of possible mechanisms. Nanoscale. 2015;7(6):2154–98.
Zhao X. Self-assembly of DNA segments on graphene and carbon nanotube arrays in aqueous solution: A molecular simulation study. J Phys Chem C. 2011;115(14):6181–9.
Ciccia A, Elledge SJ. The DNA damage response: making it safe to play with knives. Mol Cell. 2010;40(2):179–204.
Satoshi F, Macconmara MP, Maung AA, Yan Z, Mannick JA, Lederer JA, et al. Platelet depletion in mice increases mortality after thermal injury. Blood. 2006;107(11):4399–406.
Chen GY, Yang HJ, Lu CH, Chao YC, Hwang SM, Chen CL, et al. Simultaneous induction of autophagy and toll-like receptor signaling pathways by graphene oxide. Biomaterials. 2012;33(27):6559–69.
Zhou H, Zhao K, Li W, Yang N, Liu Y, Chen C, et al. The interactions between pristine graphene and macrophages and the production of cytokines/chemokines via TLR- and NF-kappaB-related signaling pathways. Biomaterials. 2012;33(29):6933–42.
Lawrence T. The nuclear factor NF-kappaB pathway in inflammation. Cold Spring Harb Perspect Biol. 2009;1(1943–0264 (Electronic)):a001651.
Hengartner MO. The biochemistry of apoptosis. Nature. 2000;407(6805):770–6.
Matesanz MC, Vila M, Feito MJ, Linares J, Goncalves G, Vallet-Regi M, et al. The effects of graphene oxide nanosheets localized on F-actin filaments on cell-cycle alterations. Biomaterials. 2013;34(5):1562–9.
Yao Y, Costa M. Genetic and epigenetic effects of nanoparticles. J Mol Genet Med. 2013;7:86.
Stern ST, Adiseshaiah PP, Crist RM. Autophagy and lysosomal dysfunction as emerging mechanisms of nanomaterial toxicity. Part Fibre Toxicol. 2012;9(1743–8977 (Electronic)):1.
Mizushima N, Yoshimori T, Levine B. Methods in mammalian autophagy research. Cel. 2010;140(1097–4172 (Electronic)):313–26.
Patel AS, Lin L, Geyer A, Haspel JA, An CH, Cao J, et al. Autophagy in idiopathic pulmonary fibrosis. PLoS One. 2012;7(1932–6203 (Electronic)):e41394.
Levine B, Mizushima N, Virgin HW. Autophagy in immunity and inflammation. Nature. 2011;469(7330):323–35.
Kenzaoui BH, Bernasconi CC, Guney-Ayra S, Juillerat-Jeanneret L. Induction of oxidative stress, lysosome activation and autophagy by nanoparticles in human brain-derived endothelial cells. Biochem J. 2012;441(1470–8728 (Electronic)):813–21.
Hussain S, Garantziotis S. Interplay between apoptotic and autophagy pathways after exposure to cerium dioxide nanoparticles in human monocytes. Autophagy. 2013;9(1554–8635 (Electronic)):101–3.
Sun T, Yan Y, Zhao Y, Guo F, Jiang C. Copper oxide nanoparticles induce autophagic cell death in A549 cells. PLoS One. 2012;7(1932–6203 (Electronic)):e43442.
Chen GY, Meng CL, Lin KC, Tuan HY, Yang HJ, Chen CL, et al. Graphene oxide as a chemosensitizer: Diverted autophagic flux, enhanced nuclear import, elevated necrosis and improved antitumor effects. Biomaterials. 2015;40:12–22.
Chen GY, Chen CL, Tuan HY, Yuan PX, Li KC, Yang HJ, et al. Graphene oxide triggers toll-like receptors/autophagy responses in vitro and inhibits tumor growth in vivo. Adv Healthc Mater. 2014;3(9):1486–95.
Wan B, Wang ZX, Lv QY, Dong PX, Zhao LX, Yang Y, et al. Single-walled carbon nanotubes and graphene oxides induce autophagosome accumulation and lysosome impairment in primarily cultured murine peritoneal macrophages. Toxicol Lett. 2013;221(1879–3169 (Electronic)):118–27.
Markovic ZM, Ristic BZ, Arsikin KM, Klisic DG, Harhaji-Trajkovic LM, Todorovic-Markovic BM, et al. Graphene quantum dots as autophagy-inducing photodynamic agents. Biomaterials. 2012;33(29):7084–92.
Sanjuan MA, Dillon CP, Tait SW, Moshiach S, Dorsey F, Connell S, et al. Toll-like receptor signalling in macrophages links the autophagy pathway to phagocytosis. Nature. 2007;450(7173):1253–7.
Sasidharan A, Swaroop S, Chandran P, Nair S, Koyakutty M. Cellular and molecular mechanistic insight into the DNA-damaging potential of few-layer graphene in human primary endothelial cells. Nanomed. 2016;12(1549–9642 (Electronic)):1347–55.
Yang H, Rivera Z, Jube S, Nasu M, Bertino P, Goparaju C, et al. Programmed necrosis induced by asbestos in human mesothelial cells causes high-mobility group box 1 protein release and resultant inflammation. Proc Natl Acad Sci U S A. 2010;107(1091–6490 (Electronic)):12611–6.
Raucci A, Palumbo R, Bianchi ME. HMGB1: a signal of necrosis. Autoimmunity. 2007;40(4):285–9.
Smith ZD, Meissner A. DNA methylation: roles in mammalian development. Nat Rev Genet. 2013;14(3):204–20.
Fabian MR, Sonenberg N. The mechanics of miRNA-mediated gene silencing: a look under the hood of miRISC. Nat Struct Mol Biol. 2012;19(6):586–93.
Nishikura K. Functions and regulation of RNA editing by ADAR deaminases. Annu Rev Biochem. 2010;79(79):321–49.
Dubey P, Matai I, Kumar SU, Sachdev A, Bhushan B, Gopinath P. Perturbation of cellular mechanistic system by silver nanoparticle toxicity: Cytotoxic, genotoxic and epigenetic potentials. Adv Colloid Interf Sci. 2015;221:4–21.
Collins AR, Ferguson LR. DNA repair as a biomarker. Mutat Res. 2012;736(1–2):2–4.
Zhao Y, Wu Q, Wang D. An epigenetic signal encoded protection mechanism is activated by graphene oxide to inhibit its induced reproductive toxicity in Caenorhabditis elegans. Biomaterials. 2016;79(1878–5905 (Electronic)):15–24.
Liu C, Yu W, Chen Z, Zhang J, Zhang N. Enhanced gene transfection efficiency in CD13-positive vascular endothelial cells with targeted poly(lactic acid)-poly(ethylene glycol) nanoparticles through caveolae-mediated endocytosis. J Contr Rel. 2011;151(1873–4995 (Electronic)):162–75.
Ema M, Aoyama H, Arima A, Asano Y, Chihara K, Endoh K, et al. Historical control data on prenatal developmental toxicity studies in rabbits. Congenit Anom. 2012;52(3):155–61.
Ema M, Endoh K, Fukushima R, Fujii S, Hara H, Hirata-Koizumi M, et al. Historical control data on developmental toxicity studies in rodents. Congenit Anom. 2014;54(3):150–61.
Bitounis D, Ali-Boucetta H, Hong BH, Min DH, Kostarelos K. Prospects and challenges of graphene in biomedical applications. Adv Mater. 2013;25(16):2258–68.
Van Goethem F, Lison D, Kirsch-Volders M. Comparative evaluation of the in vitro micronucleus test and the alkaline single cell gel electrophoresis assay for the detection of DNA damaging agents: genotoxic effects of cobalt powder, tungsten carbide and cobalt-tungsten carbide. Mutat Res. 1997;392(1–2):31–43.
Natarajan V, Wilson CL, Hayward SL, Kidambi S. Titanium dioxide nanoparticles trigger loss of function and perturbation of mitochondrial dynamics in primary hepatocytes. PLoS One. 2015;10(1932–6203 (Electronic)):e0134541.
Hong F, Zhao X, Chen M, Zhou Y, Ze Y, Wang L, et al. TiO2 nanoparticles-induced apoptosis of primary cultured Sertoli cells of mice. J Biochem Mater Res A. 2016;104(1552–4965 (Electronic)):124–35.
Yang WE, Lan MY, Lee SW, Chang JK, Huang HH. Primary human nasal epithelial cell response to titanium surface with a nanonetwork structure in nasal implant applications. Nanoscale Res Lett. 2015;10(1931–7573 (Print)):1–10.
Wang J, Deng X, Zhang F, Chen D, Ding W. ZnO nanoparticle-induced oxidative stress triggers apoptosis by activating JNK signaling pathway in cultured primary astrocytes. Nanoscale Res Lett. 2014;9(1931–7573 (Print)):1–12.
Osmond-McLeod MJ, Osmond RI, Oytam Y, McCall MJ, Feltis B, Mackay-Sim A, et al. Surface coatings of ZnO nanoparticles mitigate differentially a host of transcriptional, protein and signalling responses in primary human olfactory cells. Part Fibre Toxicol. 2013;10(1743–8977 (Electronic)):1.
Meng S, Peng R. Growth and follow-up of primary cortical neuron cells on nonfunctionalized graphene nanosheet film. J Appl Biomater Funct Mater. 2016;14(2280–8000 (Electronic)):e26–34.
Kwon JT, Seo GB, Jo, Lee M, Kim HM, Shim I, et al. Aluminum nanoparticles induce ERK and p38MAPK activation in rat brain. Toxicol Res. 2013;29(1976–8257 (Print)):181–5.
Radcliffe PM, Olabisi AO, Wagner DJ, Leavens T, Wong BA, Struve MF, et al. Acute sodium tungstate inhalation is associated with minimal olfactory transport of tungsten (188W) to the rat brain. Neurotoxicology. 2009;30(1872–9711 (Electronic)):445–50.
Zhang H, Li ZF, Snyder A, Xie J, Stanciu LA. Functionalized graphene oxide for the fabrication of paraoxon biosensors. Anal Chim Acta. 2014;827:86–94.
Schriver M, Regan W, Gannett WJ, Zaniewski AM, Crommie MF, Zettl A. Graphene as a long-term metal oxidation barrier: worse than nothing. ACS Nano. 2013;7(1936-086X (Electronic)):5763–8.
Soldano C, Mahmood A, Dujardin E. Production, properties and potential of graphene. Carbon. 2010;48(8):2127–50.
Han SG, Kim JK, Shin JH, Hwang JH, Lee JS, Kim TG, et al. Pulmonary Responses of Sprague–Dawley Rats in Single Inhalation Exposure to Graphene Oxide Nanomaterials. Biomed Res Int. 2015;2015:376756.
Pan WY, Huang CC, Lin TT, Hu HY, Lin WC, Li MJ, et al. Synergistic antibacterial effects of localized heat and oxidative stress caused by hydroxyl radicals mediated by graphene/iron oxide-based nanocomposites. Nanomedicine. 2016;12(2):431–8.
Yang K, Gong H, Shi X, Wan J, Zhang Y, Liu Z. Invivo biodistribution and toxicology of functionalized nano-graphene oxide in mice after oral and intraperitoneal administration. Biomaterials. 2013;34(11):2787–95.
Jaworski S, Sawosz E, Kutwin M, Wierzbicki M, Hinzmann M, Grodzik M, et al. In vitro and in vivo effects of graphene oxide and reduced graphene oxide on glioblastoma. Int J Nanomedicine. 2015;10:1585–96.
Akhavan O, Ghaderi E, Emamy H, Akhavan F. Genotoxicity of graphene nanoribbons in human mesenchymal stem cells. Carbon. 2013;54(2):419–31.
Chatterjee N, Yang J, Choi J. Differential genotoxic and epigenotoxic effects of graphene family nanomaterials (GFNs) in human bronchial epithelial cells. Mut Res Gen Tox Environ Mutagenesis. 2016;798–799:1–10.
Download references
Acknowledgements
Not applicable.
This review was supported by the National Natural Science Foundation of China (81550011, 51172283, 81400557), Natural Science Foundation of Guangdong Province (2015A030313299) and Guangdong Provincial Medical Research Foundation (A2016360).
Availability of data and materials
Databases/repositories and materials is not applicable in this review.
Authors’ contributions
All authors contributed to the design and concept of this article. LO drafted the manuscript. BS and JL critically revised the manuscript. All authors read and approved the final manuscript.
Competing interest
The authors declare that they have no competing interests.
Consent for publication
Ethics approval and consent to participate, author information, authors and affiliations.
Nanfang Hospital, Southern Medical University, Guangzhou, 510515, China
Bin Song, Huimin Liang, Jia Liu, Xiaoli Feng & Longquan Shao
The First Affiliated Hospital of Jinan University, Guangzhou, China
Lingling Ou & Ting Sun
The General Hospital of People’s Liberation Army, Beijing, China
You can also search for this author in PubMed Google Scholar
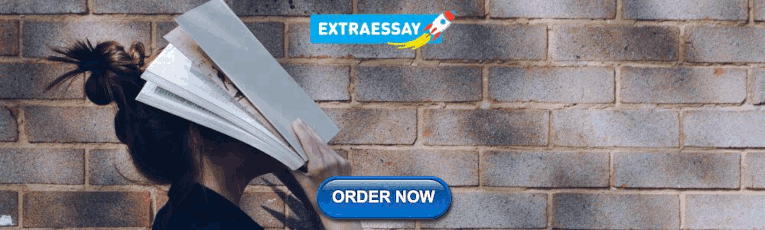
Corresponding author
Correspondence to Longquan Shao .
Rights and permissions
Open Access This article is distributed under the terms of the Creative Commons Attribution 4.0 International License ( http://creativecommons.org/licenses/by/4.0/ ), which permits unrestricted use, distribution, and reproduction in any medium, provided you give appropriate credit to the original author(s) and the source, provide a link to the Creative Commons license, and indicate if changes were made. The Creative Commons Public Domain Dedication waiver ( http://creativecommons.org/publicdomain/zero/1.0/ ) applies to the data made available in this article, unless otherwise stated.
Reprints and permissions
About this article
Cite this article.
Ou, L., Song, B., Liang, H. et al. Toxicity of graphene-family nanoparticles: a general review of the origins and mechanisms. Part Fibre Toxicol 13 , 57 (2016). https://doi.org/10.1186/s12989-016-0168-y
Download citation
Received : 13 June 2016
Accepted : 13 October 2016
Published : 31 October 2016
DOI : https://doi.org/10.1186/s12989-016-0168-y
Share this article
Anyone you share the following link with will be able to read this content:
Sorry, a shareable link is not currently available for this article.
Provided by the Springer Nature SharedIt content-sharing initiative
- Graphene-family nanomaterials
- Toxicokinetics
- Physicochemical properties
- Future prospects
Particle and Fibre Toxicology
ISSN: 1743-8977
- General enquiries: [email protected]

An official website of the United States government
The .gov means it’s official. Federal government websites often end in .gov or .mil. Before sharing sensitive information, make sure you’re on a federal government site.
The site is secure. The https:// ensures that you are connecting to the official website and that any information you provide is encrypted and transmitted securely.
- Publications
- Account settings
Preview improvements coming to the PMC website in October 2024. Learn More or Try it out now .
- Advanced Search
- Journal List
- Materials (Basel)

Synthesis and Applications of Graphene Oxide
Associated data.
The data presented in this study are available on request from the corresponding author. The data are not publicly available due to privacy.
Thanks to the unique properties of graphite oxides and graphene oxide (GO), this material has become one of the most promising materials that are widely studied. Graphene oxide is not only a precursor for the synthesis of thermally or chemically reduced graphene: researchers revealed a huge amount of unique optical, electronic, and chemical properties of graphene oxide for many different applications. In this review, we focus on the structure and characterization of GO, graphene derivatives prepared from GO and GO applications. We describe GO utilization in environmental applications, medical and biological applications, freestanding membranes, and various composite systems.
1. Introduction
Graphene is one of the most studied materials in the world; thanks to its unique properties, it was called a “material of the future” [ 1 ]. Graphene consists only of carbon atoms where every carbon atom is attached to three other carbon atoms with sp 2 hybridized orbitals making a honeycomb lattice [ 2 ]. Graphene’s rare properties make it a very promising material for a huge variety of applications, including field-effect transistors (FETs), gas and biomolecules sensors, transparent conductive films (TCFs), and graphene batteries [ 3 , 4 , 5 , 6 , 7 ].
Graphene oxide (GO) is a layered carbon structure with oxygen-containing functional groups (=O, -OH, -O-, -COOH) attached to both sides of the layer as well as the edges of the plane [ 8 ]. As with any 2D carbon material, GO can also have either single layer or multilayer structure. A structure with one layer is graphene oxide; two layers of graphene oxide are referred to as a two-layered GO. GO with more than two layers and less than five layers is called few-layered graphene oxide, GO with five to ten layers is called multilayered GO, and material with eleven or more layers is called graphite oxide [ 9 ]. GO can be synthesized by the oxidation of graphite into graphite oxide followed by the exfoliation of this graphite oxide into GO. The properties of the material are strongly dependent on the synthesizing method, which influences the resulting number and type of oxygen-containing groups in the formed GO. In contrary to graphene, GO is hydrophilic, and it is hence relatively simple to prepare a water- or organic solvent-based suspensions. Highly oxidized forms of GO are electric insulators with a bandgap of approximately 2.2 eV.
Due to the presence of various oxygen functionalities on the surface of GO, GO can be used as a starting material for the synthesis of graphene derivatives such as fluorographene, bromographene, graphane, and many others. On the other hand, by thermal or chemical reduction of GO, thermally or chemically reduced graphene can be prepared (see Figure 1 ). Interestingly, GO can also be used for advanced applications such as for drug delivery, in high-temperature materials, or in construction materials. There are still some remaining issues that can be improved and studied more intensively. It is very important to develop novel methods of environmentally friendly low-cost large-scale synthesis of GO. In this review, we tried to summarize available knowledge about GO structure, synthesis and characterization of GO, GO functionalization, and selected GO applications.

Scheme of preparation and utilization of GO (colors of atoms: grey—carbon, red—oxygen, yellow—fluorine, white—hydrogen).
2. Structure of GO
Over the years, the structure of GO was studied in detail using several instrumental techniques: annular dark-field imaging, 13 C and 1 H NMR, ultra-high-resolution transmission electron microscopy, X-ray diffraction, and many others [ 10 , 11 , 12 , 13 ]. Despite the number of attempts to reveal the structure of GO, a number of possible structural models exist with no unambiguous one. The main reason for this is the complexity of the material and the originality of every sample with variable stoichiometry [ 14 ]. Simplistically, GO is a monolayer sheet of graphite containing hydroxyl, carboxyl, and epoxy oxygen groups on its basal plane and edges, resulting in a mixture of sp 2 and sp 3 hybridized carbon atoms [ 15 ].
Many models of GO have been developed based on a number of analyses and theoretical simulations. The first model was suggested by Hofmann and Rudolf [ 16 ] in 1939, where a lot of epoxy groups were distributed randomly across the graphite monolayer. Then, in 1946, Ruess [ 17 ] updated the model by incorporating hydroxyl groups and alternating sp 2 hybridized carbons with those revealing sp 3 hybridization. In 1969, Scholz and Boem [ 18 ] suggested a less organized structure with double bonds C=C and periodically recurring C-C single bonds in the carbon layers that are corrugated with hydroxyls and carbonyls, without ether oxygen. Later, in 1994, Nakajima and Matsuo [ 19 ] proposed a model that resembled graphite intercalation compound. Then, in 1998, Lerf and Klinowski [ 11 ] created a model (LK model) that contains two different kinds of regions: regions with six-membered aliphatic rings and regions with nonoxidized benzene aromatic rings (see Figure 2 ). The size of the two regions is dependent on the level of material oxidation. The model is composed mainly of aromatic bodies, epoxide groups, and double bonds. Wrinkling in the monolayer is caused by the slightly distorted tetrahedral configuration of hydroxyl groups attached to carbon atoms. The oxygen functional groups are attached to the monolayer of carbon above and below, creating two layers of oxygen atoms with variable concentrations composed mainly of epoxide and hydroxyl groups that are very close to each other. All of the oxygen functionalities, aromatic bodies, and oxidized rings are distributed randomly across the carbon monolayer. The acidity of GO can be explained by the oxygen groups that are attached to the edges of the lattice, which are hydroxyl and carboxyl groups. This LK model has become one of the most acceptable and used models for moderately oxidized GO.

Lerf–Klinowski model of graphene oxide.
After the discovery of graphene, researchers worldwide started to focus on GO and other derivatives. In 2006, Szábó and Dékány examined previous models by a number of analyses and suggested a model without carboxylic acids composed of two main regions: corrugated hexane ribbons occupied with quinones and ketones, and translinked cyclohexane chairs with 1,3-epoxide and tertiary alcohols. In 2013, Dimiev, Alemany, and Tour [ 20 ] proposed a dynamical structural model (DSM) that describes the development of various carbon structures with attached water, contrary to the static LK model. Recently, Liu et al. [ 21 ] experimentally observed the evidence of the C=O bonds on the edge of the carbon monolayer, confirming parts of the earlier models, especially the LK model [ 22 ]. Real GO also includes some defects, such as topological defects (pentagons, heptagons, octagons, etc.), adatoms, vacancies, and adsorbed impurities.
By using concentrated acids for oxidation, low-molecular-weight fragments are produced, known as oxidation debris [ 23 , 24 ]. Oxidation debris is a mixture of highly oxidized polyaromatic fragments adsorbed on the poorly oxidized GO platelets by π–π stacking, hydrogen bonding, and van der Walls interactions [ 25 ]. The amount of the oxidation debris is strongly influenced by the reaction time of graphite and concentrated acids [ 26 , 27 ]. To wash away those fragments, a base washing is needed [ 27 , 28 , 29 ]. The pure GO without the oxidation debris presents an oxidation level that is similar to chemically reduced GO [ 30 ].
3. Conventional Routes of GO Synthesis
The first attempt to synthesize graphite oxide was performed in 1859 by British chemist B. C. Brodie who investigated the reactivity of flake graphite [ 31 , 32 ]. It is a chlorate route, where potassium chlorate is used as an oxidizing agent. Benjamin Brodie treated graphite with a number of strong oxidizing agents for the first time to decode its structure. In the experiment, he treated graphite in a mixture of potassium chlorate and fuming nitric acid at 60 °C for 4 days (Brodie’s graphene oxide (BR-GO)). He performed multiple oxidative treatments one after another (4–7) and the resulting composition of carbon, oxygen, and hydrogen was estimated as C 11 H 4 O 5 (corresponds to C/O ratio 2.2) [ 32 ]. The product was found to be soluble in pure water, while it tended to flocculate in a more acidic environment. Brodie named the product “graphic acid” because it had a slight reaction with litmus paper. Another chlorate route is the Staudenmaier method [ 33 ]. Later, L. Staudenmaier modified Brodie’s method by adjusting the way the chlorate was added and also adding sulfuric acid into the mixture (ST-GO—Staudenmaier’s graphene oxide). Potassium chlorate was added in small portions into the mixture in order to eliminate the danger of explosive by-products and heat evolution. The increased acidic environment caused a decrease in terms of reaction time. The obtained material has very similar properties to BR-GO. In 1937, Hofmann used potassium chlorate and nonfuming nitric acid to synthesize Hofmann’s graphene oxide (HO-GO) with lower oxygen content (C/O ratio 2.5). It was found that the concentration of nitric acid highly influences the level of oxidation of the resulting graphite oxide or graphene oxide [ 34 ]. The lower the concentration of nitric acid, the higher level of oxidation of graphene oxide.
The most used and effective method of all time is one of the permanganate methods, the Hummers method [ 35 ] created by Hummers and Offeman. It is a relatively fast conventional method used for the synthesis of GO. In this method, the reaction mixture is composed of an excess of potassium permanganate, sulfuric acid, and a small amount of sodium nitrate. The reaction time ranges between 8 and 12 h. This route is much safer because it avoids the creation of explosive ClO 2 . At the end of the reaction, the excess of the potassium permanganate is neutralized with a diluted solution of H 2 O 2 . The product of the Hummers’ method (Hummers’ graphene oxide (HU-GO)) has a very similar C/O ratio (2.25) to the C/O ratio of BR-GO (2.2). Unfortunately, this method is not environment-friendly, because of NO x that evolves during the reaction. There are several modified Hummers methods, including nitrate-free [ 36 , 37 , 38 ], two-step [ 36 , 37 ], co-oxidant [ 38 ], and low- and room-temperature [ 39 , 40 ] methods. Then, in 2010, Tour developed his own method, Tour’s method, which is described below.
4. Modern Ways of GO Synthesis
There are several ways to prepare graphite oxide/graphene oxide. The most common way is to use an oxidizing agent in an acidic environment. Other methods are electrochemical and microbial.
In 2010, a novel method was developed. Tour’s method [ 41 ] (Tour’s graphene oxide (TO-GO)) also falls under permanganate methods. In this procedure, phosphoric acid is mixed with sulfuric acid in the ratio 1:9 and potassium permanganate and graphite added in the ratio 6:1 in an ice bath ( Figure 3 A). The mixture is then heated at 50 °C and stirred for 12 h ( Figure 3 B). After cooling down, the mixture is poured onto ice ( Figure 3 C). Finally, 30% H 2 O 2 is added in order to remove the excess of potassium permanganate ( Figure 3 D). Phosphoric acid works as a dispersive and etching agent, as well as a stabilizer of the oxidation process, which makes the synthesis of GO safe. This route produces a higher yield of GO with a higher level of oxidation and a more regular structure.

Photographs describing preparation process of GO by Tour’s method: ( A ) before addition of potassium permanganate; ( B ) after oxidation; ( C ) after pouring on ice; ( D ) after addition of H 2 O 2.
Besides permanganate and chlorate methods, there are more modern ways to oxidize graphite in order to prepare GO, including the use of potassium chromate in combination with perchloric or nitric acid [ 42 ] or under the Jones conditions [ 43 , 44 ]. Alternatively, less toxic potassium ferrate in sulfuric acid [ 45 ] can be applied for GO preparation. Contrary to these results, another study evidences why it is not possible to prepare GO by using potassium ferrate [ 46 ]. Moreover, graphite can be oxidized in water with H 2 O 2 at 50 °C by Fe(VI) [ 47 ] or at 110 °C with benzoyl peroxide [ 48 ]. Let us note that GO prepared by chemical routes often displays a highly damaged structure due to the harsh acidic conditions of the synthesis as well as the presence of impurities. Such characteristics are indeed far from being optimal for electronics applications. Even though chemical, especially chlorate and permanganate, ways of preparation provide GO with poor electrical properties, the exploration is not at its end yet. For example, in 2017, Jankovský et al. modified Tour’s method and suggested that the shortened reaction time (from 12 h to 1 h) has no significant impact on the resulting material [ 49 ]. In 2018, Ranjan et al. [ 50 ] also modified Tour’s method and proposed the oxidation process of graphite flakes in permanganate (ratio 1:6) in a mixture of sulfuric and phosphoric acids (ratio 9:1) heated at 65 °C for 12 h. All of the chemicals were precooled at 5 °C.
Apart from the chemical routes, electrochemical synthesis represents another approach to GO synthesis that might be the key to large-scale production. Electrochemical production is more eco-friendly than chemical production due to reusing the electrolyte multiple times and minimal washing of the utensils [ 51 , 52 ]. The better quality of electrochemical GO (EGO), in contrast to standard procedures, can be explained by the use of aqueous electrolytes and no need for oxidizing agents, hence avoiding impurities [ 52 ]. Moreover, thanks to the variety of experimental setups, the level of oxidation and density of defects can be controlled.
Interestingly, the usage of biological systems to oxidize graphitic materials is very important to obtain eco-friendly graphene oxide. However, after the microbial cultivation, graphite is not homogeneously oxidized. Acidithiobacillus ferrooxidans or Pseudomonas have been tested [ 53 ] as oxidizing bacteria.
5. Derivatives of GO
Derivatives of graphene oxide are materials based on GO as a starting material. These involve graphene acid (GAF), a highly oxidized GO exhibiting a composition close to [C 1 (COOH) 1 ] n ; chemically reduced graphene oxide (CRG) and thermally reduced graphene oxide (TRG), which are reduced forms of GO with some remaining oxygen functionalities left in the structure; and fluorographene, a fluorinated form of graphene with composition [C 1 F 1 ] n (see Figure 1 ). The functionalization of graphene oxide is possible thanks to the presence of oxygen functionalities, unlike in other carbon nanomaterials.
For a closer inspection of the essential characteristics of those derivatives, we performed transmission electron microscopy ( Figure 4 left), scanning electron microscopy ( Figure 4 right), and energy-dispersive spectroscopy ( Figure 5 ) to study the surface and composition of the samples. The first micrographs in Figure 4 of GAF show a wrinkled structure, where the flakes of GAF are connected together forming a foil-like structure. Next, micrographs of [C 1 F 1 ] n show small flakes of fluorographene which consist of multiple wrinkled sheets. Whereas micrographs of CRG do not show such wrinkled structure of multiple sheets, the last micrographs of TRG show small flakes of multiple-sheet structure which are highly wrinkled. According to EDS (see Figure 5 ), all samples consist of carbon, oxygen, and sulfur except for fluorographene which consists of carbon, oxygen, and fluorine.

TEM ( left ) and SEM ( right ) micrographs of graphene acid (GAF), fluorographene (C 1 F 1 ), chemically reduced graphene (CRG), and thermally reduced graphene (TRG).

TEM and EDS micrographs of graphene acid (GAF), fluorographene (C 1 F 1 ), chemically reduced graphene (CRG), and thermally reduced graphene (TRG).
Graphene acid (GA) is a graphene derivative with a composition close to [C 1 (COOH) 1 ] n . The synthesis of such material consists of two consecutive oxidation steps of graphite. After the first oxidation by the Tour method, GO is obtained and further used as a starting material for the second oxidation. The second oxidation runs according to the Tour method as well [ 54 ]. Further oxidation leads to a total decomposition of GA (oxidation to CO 2 ). Another possible way to synthesize GA is by acidic hydrolysis of cyanographene (graphene–nitrile) by 20% HNO 3 [ 55 ].
GO is mostly used for the production of graphene (reduced graphene oxide (rGO)) by chemical (chemically reduced graphene (CRG)) or thermal (thermally reduced graphene (TRG)) reduction. Reduced graphene oxide can be used in electronic devices, energy storage devices, (bio)sensors, biomedical applications, supercapacitors, membranes, catalysts, and water purification. As an electronic device, rGO is used in field-effect transistors (FETs) as chemical sensors and biosensors [ 56 , 57 , 58 , 59 ]. rGO was also used in light-emitting diodes (LEDs) as a transparent electrode [ 60 , 61 ]. Thanks to the extreme surface area of rGO, the material is used as an electrode in double-layered capacitors, batteries, fuel cells, and solar cells [ 62 , 63 ]. Energy storage capacity and cycle stability of Li-ion battery devices can be enhanced using Fe 3 O 4 on rGO anode rather than pure Fe 3 O 4 or Fe 2 O 3 [ 64 ]. Stacked sheets of GO have nanocapillaries between individual sheets, which are closed by chemical reduction of GO, creating a material that is impermeable to liquids, gases, and even strong chemicals. Corrosive acids can be stored in glass or copper containers that are covered inside with such graphene paint [ 65 , 66 ]. In order to improve shelf life in medical infrastructure, graphene-coated plastic films may be used [ 67 ].
Graphene oxide can be reduced thermally simply by applying heat, a process called thermal annealing reduction. Firstly, the exfoliation of GO occurs during rapid heating, where gases such as CO 2 , CO, and H 2 O are released from the sample [ 68 , 69 , 70 ]. During the rapid increase in temperature, the oxygen-containing groups transform into gases mentioned above that generate huge pressure between the stacked layers of graphene oxide. At approximately 300 °C, the pressure reaches ~40 MPa, and it is increased to ~130 MPa if the temperature is raised to 1000 °C [ 71 ]. In fact, a pressure as low as 2.5 MPa is enough to separate two stacked layers of GO, as predicted by the evaluation of the Hamaker constant [ 71 ]. The originated platelets can be referred to as graphene or TRG because the elevated temperature causes decomposition of oxygen-containing functional groups which leads to the exfoliation of the material. This could be a good strategy for bulk rGO synthesis; however, this route yields only small-sized and wrinkled graphene sheets [ 68 , 72 ]. This effect is caused by the removal of carbon atoms during the transformation of oxygen-containing groups into the above-mentioned gases, which splits wide sheets of graphene oxide into small-sized graphene sheets [ 73 , 74 ]. High temperatures of the thermal reduction lead to the emissions of highly toxic volatile organic hydrocarbons [ 75 ]. Another way to fabricate reduced graphene oxide is by a liquid-phase exfoliation in an inert atmosphere. This route is highly affected by the temperature of GO reduction [ 6 , 68 , 76 , 77 ]. At a temperature lower than 500 °C, the C/O ratio is not higher than 7, while when the temperature reaches 750 °C, the C/O ratio is very likely to be higher than 13. In addition to this effect, there is also a great importance of the used annealing atmosphere. Annealing reduction of GO can be carried out in vacuum [ 6 ], inert [ 77 ], or reducing atmosphere [ 69 , 77 , 78 , 79 ].
The first option to reduce GO chemically is using the reducing agent at room temperature or at slightly elevated temperature. It is an easy and cheap way for the mass production of graphene in comparison to the thermal reduction route. Hydrazine was the first chemical compound used for the reduction of GO even before the discovery of graphene [ 80 ]. Stankovich et al. reported the preparation of chemically derived graphene using hydrazine [ 81 , 82 ]. Apart from hydrazine, its derivatives such as hydrazine hydrate and dimethylhydrazine can be also used to reduce GO [ 83 ]. The reduction is achieved by adding the liquid reagents to a GO aqueous suspension, where the graphene-based nanosheets are agglomerated due to the increased hydrophobicity. Another great chemical reducing reagent is ascorbic acid (vitamin C), which is considered to be an ideal hydrazine substitute [ 84 ]. The resulting material has a very similar C/O ratio as the one reduced by hydrazine, but vitamin C has a great advantage of nontoxicity. Moreover, the colloid state reduction of vitamin C does not bring about a product agglomeration, which is helpful for further applications. In addition, using Ar + ion irradiation of GO foils creates highly conductive graphene papers [ 85 ].
Fluorographene is fluorinated graphene with stoichiometry [C 1 F 1 ] n [ 86 , 87 ]. As with every graphene/graphene oxide derivative, it has extraordinary electronic, optical, physical, and chemical properties that make it one of the thinnest insulators with a wide electronic gap [ 88 ]. The preparation of such a material can be divided into two strategies. The first one is based on the exfoliation of bulk graphitic materials containing fluorine atoms, while the second strategy relies on the fluorination of graphene or graphene oxide with fluorinating agents. Exfoliation can be performed in a liquid phase or mechanically. In the liquid-phase exfoliation, a medium is used to weaken the van der Waals interactions between the layers, resulting in single- or few-layer fluorographene [ 89 ]. For the first time, sulfolane was used and the mixture of the solvent and bulk graphite fluoride was sonicated for 1 h at 50 °C [ 90 ]. Isopropanol [ 91 ], ethanol [ 92 ], acetonitrile [ 93 ], and chloroform [ 94 ] can be mentioned as other reported solvents for the exfoliation of bulk graphite fluoride. Another strategy to prepare fluorographene is to combine graphene with fluorination agents such as xenon difluoride in a reactor [ 95 ]. The process can be initiated by exposing the reactants to temperature, irradiation, or pressure. Fluorographite and fluorographene can be used as a precursor for the synthesis of highly hydrogenated graphene (graphane) [ 96 ].
6. Typically Used Analytical Methods for GO
In order to provide typical analytical results of GO, a sample of GO was prepared by modified Tour’s method [ 49 ] and analyzed by SEM, EDS, and TEM. Usually, Raman spectroscopy, XPS, XRD, XRF, EA, EDS, AFM, and STA-MS are also used for the characterization.
Transmission electron microscopy (TEM), energy-dispersive spectroscopy (EDS), and scanning electron microscopy (SEM) were used to study the surface of GO. The first micrographs in Figure 6 A of TO-GO show wrinkled sheets of graphene oxide by both TEM and SEM methods. According to EDS (see Figure 6 B), the sample consists of carbon, oxygen, and sulfur. Let us note that the hydrogen is not visible on EDS.

TEM ( left ) and SEM ( right ) micrographs ( A ) and EDS micrographs ( B ) of GO.
The composition of the sample can be determined via X-ray fluorescence (XRF). The composition of GO depends on the level of oxidation of the sample. Generally, graphene oxide is composed of carbon, oxygen, and hydrogen, but there are very often other impurities from the starting materials, such as sulfur, chlorine, nitrogen, manganese, and potassium [ 97 ]. Manganese and potassium are contaminants that remain from the starting reactants (potassium permanganate), and chlorine remains from hydrochloric acid that is usually used in order to wash away the contaminants.
The composition of TO-GO can be also investigated by elemental analysis (EA) [ 98 ]. The results can also prove the presence of impurities such as sulfur and nitrogen, depending on the used synthesis method. Sulfur and nitrogen remain from the sulfuric acid and nitric acid, respectively, that are used as starting chemicals to synthesize GO. EA is a suitable method to determine hydrogen content; however, the determination of oxygen is not precise due to the indirect calculation.
Raman spectroscopy is a powerful tool for the determination of defect rate. Using Raman spectroscopy, two local maxima around 1350 cm −1 and 1600 cm −1 , respectively, are usually registered. The first local maximum is called the D band and shows a quasitetrahedral coordination with sp 3 carbon hybridization (irregularities such as functional groups). The other local maximum is called the G band and detects a planar arrangement with sp 2 hybridization (regular graphene lattice). The D/G ratio, calculated from the intensity of peaks, shows the level of oxidation of the sample and should be around 1.00 [ 99 ].
X-ray diffraction is used to determine the interlayer distance between GO layers. Whereas pure graphite has (002) reflection at 26.3° that corresponds to the interlayer distance of 3.342 Å [ 100 ], the (002) reflection for GO can be found around 10.0–12.0° [ 101 , 102 , 103 , 104 , 105 ], indicating an interlayer distance of around 7.4–9.0 Å. This significant increase in the interlayer distance of pristine graphite and graphene oxide is caused due to oxygen functional groups attached to the carbon layer.
GO may be analyzed by X-ray photoelectron spectroscopy (XPS) to determine the composition of the sample surface and more interestingly to determine the ratio of individual functional groups. Two local maxima are usually detected around ~284.4 eV for the C1s peak and around ~532.4 eV for the O1s peak. Maxima for N1s, S2s, and S2p peaks might also be found, showing the contamination of the sample by sulfur and nitrogen. The composition is very variable and depends on the level of oxidation of the sample [ 106 ]. The deconvolution of the C1s peak can be used in order to quantify individual bonds that are present in the samples, for a sample of deconvoluted C1s peak. From the C1s peak, six kinds of bonds can be distinguished: C–C at ~284.5 eV; C=C at ~285.2 eV; C-O at ~286.2 eV; C=O at ~287.8 eV, O–C=O at ~289.0 eV; and π–π* interaction at ~291.0 eV. From the O1s peak, four kinds of bonds with the following binding energy may be identified: O-C=O at ~531.2 eV, C=O at ~ 532.5 eV, C-OH at ~533.3 eV, and C-O-C at ~534.0 eV [ 107 ].
With simultaneous thermal analysis in an inert atmosphere, the temperature of exfoliation is investigated. The literature claims that the temperature of the exfoliation of graphene oxide is around 200 °C [ 108 ]. The obtained value is dependent on the heating rate and other conditions. The process of exfoliation, where stacked layers of graphene oxide are divided into individual reduced graphene oxide sheets, is accompanied by the formation of gases; gases detected by mass spectrometer were H 2 O, CO, and CO 2 .
Atomic force microscopy (AFM) can be employed in order to study the thickness of the GO sample, therefore investigating the number of layers. It is known from the literature that a monolayered sample of GO has a thickness of 0.8–1.2 nm [ 109 , 110 ]. Shenghua Lv et al. reported AFM results of graphene oxide nanosheets with a sample thickness of less than 7.7 nm (see Figure 7 ).

Typical AFM image of GO ( a ); surface patterns from AFM in positions 1, 2, and 3 ( b ) [ 111 ] (with the approval of MDPI Materials ).
7. Applications of GO
7.1. environmental applications of go.
One of the biggest threats to the environment is air pollution caused by the industrial release of harmful gases such as CO 2 , CO, NO 2 , and NH 3 . Thanks to the oxygen groups decorating the basal planes and the edges, GO is capable of covalent or noncovalent interactions with various molecules. GO can be employed in catalysis for converting polluting gases during industrial processing. The elimination of such harmful gases can be performed by capturing and storing gases, catalyst reactions of gas conversion, or direct utilization [ 112 ]. Apart from gas pollution, water pollution also represents a very huge environmental problem. The approach of GO application in this area can be divided into two paths: pollutant adsorption and conversion. The main water pollutants are heavy metal ions and organic dyes; they strongly threaten humans, aquatic life, animals, and plants.
7.1.1. Removal of Toxic Gases
The functional groups of few-layered GO composites exhibit unique adsorption behavior towards CO 2 [ 113 , 114 , 115 , 116 ]. Laminar GO structures were assembled having fast and selective channels for gas separation with excellent preferential CO 2 permeation performance [ 117 ]. Multi-permselective mixed matrix membranes and other mixed matrix membranes for efficient separation of CO 2 were developed, enhancing the diffusivity selectivity, solubility selectivity, and reactive selectivity [ 118 , 119 , 120 , 121 , 122 ]. In addition, GO-based composites have a unique ammonia adsorption capability [ 123 , 124 , 125 , 126 , 127 ]. Moreover, other harmful gases such as acetone [ 128 , 129 ], formaldehyde [ 130 ], H 2 S [ 131 , 132 , 133 ], SO 2 [ 134 , 135 , 136 ], and NO x [ 137 , 138 ] can be adsorbed by GO-based composites.
7.1.2. Conversion of CO 2
Thanks to the unique electronic properties, GO-based composites exhibit superior photocatalyst abilities for CO 2 conversion [ 139 , 140 ]. Hsu et al. [ 141 ] reported the photocatalytic conversion of carbon dioxide to hydrocarbons or their derivatives such as methanol for possible simultaneous CO 2 reduction and solar energy harvesting.
7.1.3. Water Purification
GO exhibits high adsorption ability towards Cd(II), Co(II), Au(III), Pd(II), Ga(III), and Pt(IV) [ 142 , 143 , 144 ]. Researchers Klímová et al. explored the adsorption ability of GO towards the whole periodic table. Adsorption ability mainly depends on the synthesizing method [ 145 ]. Few-layered graphene oxide nanosheets show a very high affinity towards Pb(II) ions, with a sorption capacity of about 842 mg g −1 at 293 K [ 146 ]. On the other hand, the adsorption capacity of Cu 2+ ions is very low, even with oxygen groups on GO acting as active sites [ 147 ]. With the assistance of organic compounds, GO can provide more feasible anchoring sites for heavy metal ions [ 148 , 149 , 150 , 151 , 152 , 153 , 154 , 155 ]. Additionally, graphene oxide provides the ability to adsorb other harmful water pollutants—organic dyes [ 156 , 157 , 158 , 159 ]. Molla et al. [ 160 ] reported that the selectivity of positive dye methylene blue and rhodamine B was rapid (within 15 min) with the efficiencies of 97% and 88%, respectively, whereas the negative dye, methyl orange, was not absorbed.
7.2. Medical and Biological Applications of GO
The first possible application in this field is GO-based biosensors. GO-based biosensors rely on their preferred interaction with single-strand DNA (ssDNA) rather than double-strand DNA (dsDNA). This effect is caused by the effective hiding of nucleo-bases in dsDNA in a helical structure, which prevents GO from direct interaction with nucleo-bases [ 161 , 162 , 163 ].
Another interesting application is gene delivery, which is a promising way to treat genetic disorders, including cancer. The therapy uses gene vectors protecting DNA from nuclease degradation. GO sheets have been covered by polyethyleneimine (PEI) as a surface modifier for gene delivery into the cells. The delivery runs through complexation by electrostatic interaction and covalent conjugation for the loading of plasmid DNA (pDNA) [ 161 , 164 ].
Small-molecule drug delivery seems to be another promising medical application of GO. Small molecules of drugs can be attached to a GO surface using pH-sensitive linkers. A complex of doxorubicin and GO (DOX-GO) shows a release of DOX from GO dependent on pH due to higher solubility of DOX at low pH [ 165 ]. Moreover, cancer-targeting was successfully manifested as a codelivery of camptothecin (CPT) using folic acid conjugated nano-GO (FA-NGO) [ 166 ].
7.3. GO Membranes
GO membranes may be used as ionic and molecular sieves or for selective gas transport. GO membranes were first introduced to the world by Nair et al. [ 167 ]. It was reported that a membrane of pure graphene oxide can block everything except for water vapor (see Figure 8 A). Nair et al. claimed that a GO membrane allows only water vapor to pass through ( Figure 8 B), while ethanol and other alcohol molecules are blocked from passing through. The membrane can be prepared by vacuum filtration or by spraying a suspension of GO on a solid surface and then etching away the membrane from the surface. Others reported a study exploring the dependence of gases that pass through on the number of layers of GO. In other words, the selective diffusion of gases can be accomplished by the regulation of gas flow pores and channels by various stacking strategies [ 113 ].

Membrane system scheme ( A ); schematic of possible water transport mechanism ( B ).
As a reaction to Nair’s paper, Sun et al. [ 168 ] demonstrated a selective ion penetration through the GO membrane. They reported that salts of heavy metals and organic pollutants take a much longer time to permeate than sodium salts that pass through quite freely. The reason for this observation is that the salts of metals pass through capillaries in the membrane, where the heavy metal ions create coordination between the GO membrane and those ions that block the permeation. In 2014, Gao et al. [ 169 ] proposed the use of an ozonated GO membrane, having more oxygen functional groups, to improve proton conductivity in fuel cell applications at higher humidity. The water surface was used as a template for assembling the GO film on it by using the amphiphilicity of the GO [ 170 ].
7.4. High-Temperature Materials and GO
Graphene and graphene oxide are very promising materials for the reinforcement and general enhancement of mechanical properties of high-temperature materials. Some researchers studied the effects of graphene oxide on high-temperature materials such as metal alloys and ceramics. The mechanical resistance can be significantly improved by only 1 vol.% of GO. High energy ball milling was used to disperse graphene oxide powder in an aluminum (AlMg5) alloy matrix. Hot pressing was used to densify the obtained material [ 171 ]. More frequently, the reinforcement of high-temperature ceramics or graphene oxide/reduced graphene oxide coatings has been found to achieve better corrosion resistance. Spark plasma sintered Si 3 N 4 ceramic matrix was enriched by multilayered graphene or graphene oxide to study the influence of the addition on mechanical, tribological, and electrical properties. The addition of multilayered graphene caused higher hardness, modulus, and bending strength in comparison to graphene oxide addition. However, the addition of graphene oxide and multilayered graphene resulted in lower mechanical properties but better electrical and tribological properties [ 172 ].
7.5. Building Materials and GO
Ordinary Portland cement (OPC) is one of the most used materials in the field of civil engineering thanks to the thirst for urbanization. The concrete is produced by mixing aggregates, binder (OPC), and water for hydration. Concrete has its advantages, such as unique compressive strength, as well as disadvantages, including poor crack formation resistance or low tensile strength [ 173 ]. Researchers have attempted many times to enhance the properties of cement-based materials by admixtures [ 174 , 175 , 176 ], fibers [ 177 , 178 ], and supplementary cementitious materials [ 179 , 180 ]. In more recent studies, newly produced nanomaterials such as nano-titanium oxide, nano-silica, nano-iron oxide, carbon nanotubes, and graphene oxide have been incorporated into the cement-like structures to enhance the mechanical properties of such materials. Such nanoparticles are able to fill even the smallest pores in the cement, providing a compact structure. Since GO is a two-dimensional material, it offers a large surface area for C-S-H nucleation [ 181 , 182 ]. The large surface area and the presence of functional groups make GO a highly reactive material. Mechanical properties of graphene are degraded by functionalization, meaning that GO shows lower elastic modulus and tensile strength than graphene. However, GO’s tensile strength and elastic modulus are still superior to those of cement—adding GO to cement-like materials enhances the mechanical properties of such building materials. Introducing small amounts of GO (0.05 wt.%) increases the flexural strength by 40–60% and compressive strength by 15–33% [ 182 ].
Magnesium oxychloride cements (MOCs) are promising alternatives to Portland cement. Let us note that OPC production is connected to high emissions of CO 2 during manufacturing [ 183 ]. An alternative building material that can reduce the impact of CO 2 during the carbonation is magnesium oxychloride cement (also known as Sorel cement) [ 184 ]. In order to enhance its flexural and compressive strength, carbonaceous nanomaterials, such as graphene, graphene oxide, or graphite oxide, can be added to the mixture (see Figure 9 ) [ 97 ]. Even the very poor water resistance can be improved by the addition of graphene [ 185 ].

SEM micrograph of MOC-GO composite.
8. Conclusions
In this article, the history, synthesis, properties, and application of graphene oxide were reviewed. There are many GO derivatives, including graphene acid, fluorographene, and graphene oxide reduced by thermal or chemical reduction (TRG or CRG). Graphene oxide as well as its derivatives have plenty of various applications. Thanks to the oxygen functional groups on the edges and basal plane, GO can be used as a solution for environmental problems such as excess of CO 2 and toxic gases such as ammonia, acetone, formaldehyde, H 2 S, SO 2, and NO x . There is also a possibility to convert CO 2 by photocatalytic reaction using GO-based composites. Organic dyes and inorganic heavy metal ions in water represent another worldwide environmental issue that can be solved by using GO. There are also medical applications such as gene delivery, which is a very promising way of treating genetic disorders; drug delivery for targeting cancer; and GO-based biosensors. GO-based membranes can be employed as molecular and ionic sieves or for selective gas transport. In high-temperature materials, GO works mainly as an additive used for reinforcement and general enhancement of mechanical properties. Employing GO into building materials such as ordinary Portland cement or magnesium oxychloride can enhance their flexural and compressive strength as well as MOC’s poor water resistance.
Author Contributions
Conceptualization, O.J. and D.S.; writing—original draft preparation, A.J., Z.S., D.S. and O.J.; visualization, A.J.; supervision, O.J. All authors have read and agreed to the published version of the manuscript.
This research was funded by the CZECH SCIENCE FOUNDATION, grant number 20-01866S. This work was also supported by the grant of Specific University Research—Grant MSMT No. 20-SVV/2022.
Institutional Review Board Statement
Not applicable.
Informed Consent Statement
Data availability statement, conflicts of interest.
The authors declare no conflict of interest.
Publisher’s Note: MDPI stays neutral with regard to jurisdictional claims in published maps and institutional affiliations.
Accessibility Links
- Skip to content
- Skip to search IOPscience
- Skip to Journals list
- Accessibility help
- Accessibility Help
Click here to close this panel.

The Electrochemical Society (ECS) was founded in 1902 to advance the theory and practice at the forefront of electrochemical and solid state science and technology, and allied subjects.
Find out more about ECS publications
Review—Progress of Research on the Preparation of Graphene Oxide via Electrochemical Approaches
Le Li 1 , Dan Zhang 2 , Jianping Deng 1 , Qin Kang 1 , Zhifeng Liu 2 , Junfei Fang 1 and Yuchun Gou 2
Published 5 October 2020 • © 2020 The Author(s). Published on behalf of The Electrochemical Society by IOP Publishing Limited Journal of The Electrochemical Society , Volume 167 , Number 15 Citation Le Li et al 2020 J. Electrochem. Soc. 167 155519 DOI 10.1149/1945-7111/abbbc0
Article metrics
6139 Total downloads
Share this article
Author e-mails.
Author affiliations
1 Shaanxi Key Laboratory of Industrial Automation, School of Mechanical Engineering, Shaanxi University of Technology, Hanzhong 723001, People's Republic of China
2 Shaanxi Province Key Laboratory of Catalytic Foundation and Application, School of Chemistry and Environment Science, Shaanxi University of Technology, Hanzhong 723001, People's Republic of China
Le Li https://orcid.org/0000-0002-9833-2294
- Received 12 August 2020
- Revised 22 September 2020
- Published 5 October 2020
Buy this article in print
Graphene oxide (GO), a derivative of graphene, has attracted widespread attention due to its easy functionalization and excellent water solubility. Therefore, a method for efficiently producing GO shoule be developed. Although the traditional chemical oxidation method is broadly employed for GO synthesis, it entails problems, such as long time-consuming, explosive danger and easy to pollute the environmental. Recent research on using electrochemical methods for GO synthesis has achieved a breakthrough, that is, the realization of pollution-free, safe and efficient large-scale preparation of high-quality GO within a few hours. This article introduces the principle of electrochemical GO synthesis and summarizes the progress of research on GO preparation via two-electrode, three-electrode and electrolyte exfoliation with focalize on product quality and quantity. The challenges in high-quality electrochemical GO production and future research directions are also presented.
Export citation and abstract BibTeX RIS
This is an open access article distributed under the terms of the Creative Commons Attribution 4.0 License (CC BY, http://creativecommons.org/licenses/by/4.0/ ), which permits unrestricted reuse of the work in any medium, provided the original work is properly cited.
As a functionalized 2D material for the synthesis of graphene and its composites, graphene oxide (GO) contains hydroxyl, carbonyl and epoxy groups on the basal plane in addition to carboxyl groups at the edges (Fig. 1 ). 1 – 5 These oxygen-containing functional groups endow GO with excellent dispersibility in various solvents. 6 , 7 Moreover, GO can be functionalized by chemical bonds, compounded with other materials and assembled into various large-scale graphene architectures (transparent conductive films, strong and stiff papers, multifunctional separation membranes, conductive and strong fibers, ultralight super-elastic aerogels, etc) through electrostatic interactions, van der Waals forces and hydrogen bonds. 8 – 12 These unique characteristics of GO and its derivatives have been used in electrochemical energy storage, seawater desalination and fabrication of transparent conductive films, printed electronics, sensors and polymer composite materials. 4 , 13 – 22
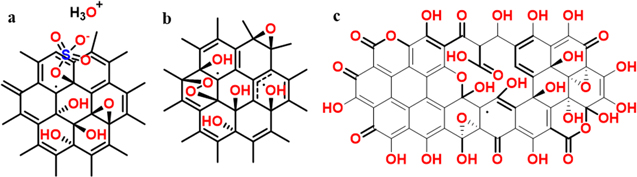
Figure 1. (a) Generalized chemical sketch of GO, ignoring the defects or functional groups on the edge of the GO sheet. (b) GO accounts for a chemical schematic diagram of free radical structures, such as carbon centers or allyl groups and cytotoxic endoperoxide groups. (c) Chemical sketch of GO accounting for π -system, butadiene structures, hydroxyl and epoxy groups and functional groups at defect sites and rims of flakes with phenol-like, carbonyl, hemiacetal, or lactol structures. 5
Download figure:
GO is usually prepared by exfoliating graphite oxide. 23 Since the first preparation of GO by Brodie in 1859, 24 the Brodie method and the subsequent Staudenmaier and Hummers methods have been the three main routes for the synthesis of graphite oxide. 25 , 26 Researchers have made extensive improvements to the three methods by fixating on enhancing the oxidation and exfoliation of graphite. However, these chemical oxidation approaches consume hours and even days and produce various toxic gases (e.g. NO x and ClO 2 ). 27 , 28 Moreover, the most broadly applied Hummers method entails a danger of explosion because of the production of intermediate Mn 2 O 7 , and the use of KMnO 4 causes permanent defects that cannot be repaired after GO reduction. 28 – 30 What's more, extra decontamination operations are needed to wipe off residuary acids and impurity metal ions in GO, and these steps produce a large volumes of wastewater. 28 Compared with graphene, GO has received considerably less attention in the investigation new synthetic methods. 31 New methods of GO synthesis, such as electrochemical stripping of graphite, 27 – 31 ball milling 32 , 33 and ultrasonic treatment, 34 have been widely used in recent years. Electrochemical exfoliation is regarded as a pollution-free, safe, efficient method of synthesising GO, and it has the following advantages: (1) no strong chemical oxidants are involved because the current is a pollution-free oxidant functionalized by graphene, (2) the process is usually completed within hours or even minutes and (3) the quality and yield of GO, such as the degree of oxidation and defect density, can be easily regulated by adjusting the electrochemical reaction conditions. 27 – 34 To improve the electrochemical process of GO synthesis, researchers have explored several strategies, including changing the raw materials, adjusting the electrolyte composition and optimising the application voltage/current. The ultra-fast large-scale preparation of high-quality GO with governable oxidation levels has become a hot spot recently. In this production process, which is a major breakthrough in this field, intercalation and oxidation are realized through electrochemical processes using different electrolytes and bias voltages. 8 , 28 , 35 As far as we know, no study has overviewed the appealing and prospective field of electrochemical GO preparation despite the valuable research results obtained in recent years.
This article describes the principle of electrochemical GO preparation via three methods, namely, two-electrode, three-electrode and electrolyte exfoliation, and summarizes the latest progress of research on electrochemical synthesis (Fig. 2 ). The electrochemical devices and conditions in two- and three-electrode exfoliation are discussed in detail. For electrolyte exfoliation, the design principles and operating procedures are described. Then, we present the current puzzles and future research directions in the field of electrochemical of GO preparation.
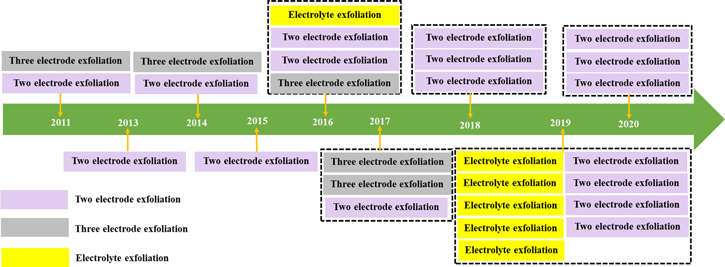
Figure 2. A brief history of the development of electrochemical preparation of GO.
Mechanism of Electrochemical GO Preparation
Graphite is composed of multiple layers of graphene with an interlayer spacing of 0.354 nm, and it is usually employed as a raw material for electrochemically preparing GO. 31 Electrochemical synthesis of GO uses the conductivity of graphite to insert anions and/or molecules in the electrolyte into graphite under a bias voltage or current to form a graphite intercalation compound. Immediately afterwards, the oxygenated material produced by the electrolyzed water further oxidizes the graphite intercalation compound to form graphite oxide, which is then subjected to ultrasonic treatment to obtain GO (Fig. 3 a). As early as 1995, F. Beck et al. discussed the formation mechanism of electrochemically prepared graphite oxide. 36 Subsequently, Parvez et al. presented the specific process of electrochemically oxidizing graphite (Fig. 3 b). 37 First, nucleophilic H 2 O molecules and the sp 2 hybridised carbon graphite to generate two hydroxyl groups under the action of electric field (Fig. 1 b [reaction 1]). These nearby hydroxyl groups can interact to generate an epoxy ring (Fig. 1 b [reaction 2]) or can be separated into two carbonyl groups by further oxidation (Fig. 1 b [reaction 3]). The evolution reaction of carbon dioxide (Fig. 1 b [reaction 4]) and oxygen (Fig. 1 b [reaction 5]) gas occurs, leading to the exfoliation of GO.
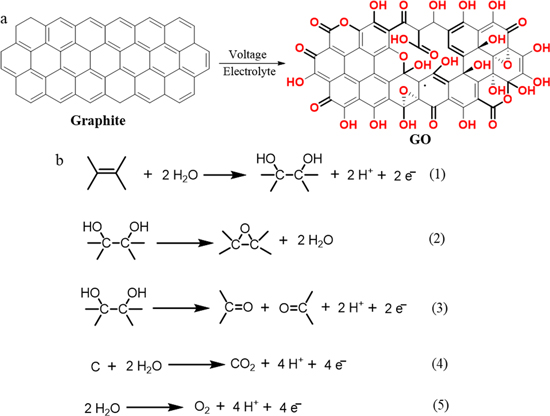
Figure 3. Schematic of (a) electrochemical preparation of GO using graphite as raw material, (b) the electrochemical graphite oxide process. 37
In accordance with the different positions of the graphite raw material in the electrochemical synthesis process, we divide exfoliation into two-electrode, three-electrode and electrolyte exfoliation. The most common amongst them is two-electrode exfoliation, which involves the intercalation, oxidation and exfoliation steps of graphite (Fig. 4 a). 28 At a low voltage (1.6 V), the HSO 4 2− intercalation produced by electrolytic concentrated H 2 SO 4 enters the graphite layer to form a graphite interlayer compound. The graphite interlayer compound oxidizes the graphite interlayer compound with 50 wt.% H 2 SO 4 as the electrolyte at a voltage of 5 V. Furthermore, O 2 and CO 2 produced by electrolysed water promote the exfoliation of the oxidized graphite interlayer compound. After further ultrasound, GO is generated (Fig. 4 b). 28
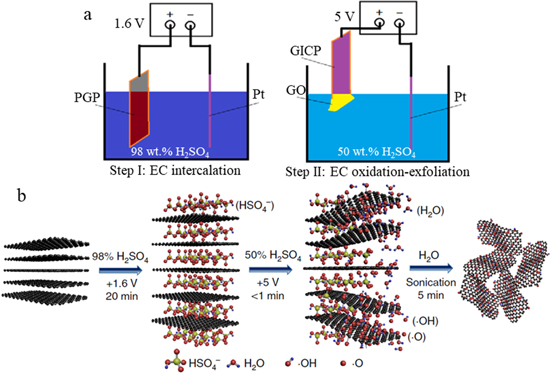
Figure 4. (a) Schematic and (b) mechanism of two electrode electrochemical exfoliation to prepare GO. 28
Three-electrode exfoliation uses an electrochemical workstation as a power source, and its schematic is shown in Fig. 5 a. The exfoliation mechanism is similar to that in two-electrode exfoliation (Fig. 5 b). In the first stage of graphite oxide formation, NO 3 – is embedded in the graphite matrix. Next, the second-stage HNO 3 –GIC is partially converted to first-stage HNO 3 –GIC and NO 3 – coexists in the synthesised GO structure. Through further oxidation, the water molecules are converted to an oxygen function combined with the graphene layer, resulting in the co-intercalation of water molecules. Potential oscillations are related to the embedding of water molecules and form adjacent OH, which is then converted into C–O and C=O bonds to form GO. 50
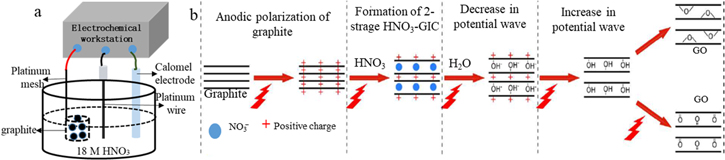
Figure 5. (a) Schematic and (b) mechanism of three electrode electrochemical exfoliation to prepare GO. 50
Electrolyte exfoliation entails the development of a suitable exfoliation device to realize GO preparation in the electrolyte (Fig. 6 a). GO is simultaneously oxidised and exfoliated in the electrolyte. In a fixed-bed electrochemical reactor, under the action of dilute H 2 SO 4 electrolyte, SO 4 2− intercalates into the graphite layer to form a graphite intercalation compound. The oxygen-containing free radicals generated by electrolyzed water form oxygen-containing functional groups in the graphite intercalation compound. In addition, the O 2 and CO 2 produced by electrolyzed water promote the exfoliation of graphite intercalation compounds to produce graphite oxide. After further ultrasound, GO is generated (Fig. 6 b). 51
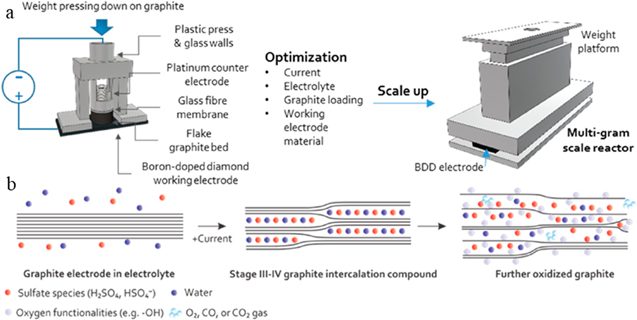
Figure 6. (a) Schematic and (b) mechanism of preparing GO by exfoliating in electrolyte. 51
Preparation of GO by Electrochemical Exfoliation
Preparation of go by two-electrode electrochemical exfoliation.
Two-electrode exfoliation, as the main method of electrochemical exfoliation to prepare 2D layered materials, can be divided into anode and cathode exfoliation. 52 – 54 Anode exfoliation involves the generation of oxygen-containing free radicals, which is one of the conditions for electrochemical synthesis of GO. In 2011, You et al. successfully prepared GO by using KCI as the electrolyte and expanded graphite and platinum wire as the anode and cathode, respectively, at a voltage of 4 V (Fig. 7 a). 38 The as-prepared GO had a C/O of 2.77 and a thickness of 1–3 layers. During the peeling process, electrostatic force causes the intercalation of Cl – to enter the anode-expanded graphite layer. Cl – forms small bubbles between the graphite layers. The bubbles expand the gap between the graphite sheets and generate a separation force between adjacent graphite layers. This separation force overcomes the van der Waals force between adjacent graphite sheets and causes the graphite layer to peel from the initial graphite layer. Given that graphene is electrochemically oxidized by chloride during the peeling process, oxygen-containing functional groups are formed in the graphene and GO is generated (Fig. 7 b). Liu et al. used square wave voltage (+7 V and –7 V were applied repeatedly every 5–8 min) to exfoliate the pencil cores in aqueous electrolytes (H 2 SO 4 or H 3 PO 4 ) and obtain high-quality GO (lateral size of 1 μ m to several μ m with a thickness between 3 and 9 nm). 39 The as-exfoliated GO flakes were utilised as a metal-free catalyst for oxygen reduction reaction and exhibited excellent electrocatalytic activity and toxicity tolerance in alkaline solution. Meanwhile, Abdelkader et al. presented a green electrochemical oxidation method that can convert a large amount of graphite into GO. 40 By using graphite as the anode in an electrochemical cell containing 0.2 M sodium citrate, graphite oxidation and exfoliation occurred simultaneously and produced GO flakes with a carbon/oxygen ratio of 7.6. They found that the oxidation degree of oxidation products can be controlled by controlling the composition of the electrolyte. Furthermore, research on the reaction mechanism shows that this exfoliation process begins with the formation of 20–100 nanoparticles and graphite nanoribbons. Afterwards, given that the anion is embedded with graphite, the bonding between graphite sheets weakens, and the entire process proceeds rapidly. Ambrosi et al. used platinum wire as the cathode and graphite foil as the anode to exfoliate in different electrolytes (H 2 SO 4 , Na 2 SO 4 , and LiClO 4 ) and studied the products after exfoliation. 41 They found that using LiClO 4 as an electrolyte can form GO with a carbon/oxygen ratio of nearly 4.0 and utilised as an electrode of a supercapacitor with a capacitance of up to 21 F g –1 . High-quality GO (thickness of 1–4 layers) with high crystallinity and electrical conductivity co-doped with nitrogen and sulphur was prepared via electrochemical exfoliation of graphite in (NH 4 ) 2 SO 4 electrolyte under the protection of N 2 . 42 When used as an electrocatalyst for oxygen reduction reactions, the iron-containing GO flakes showed good catalytic activity and stable methanol tolerance. Tian et al. successfully prepared GO by using two-electrode Tee-cell in HClO 4 electrolyte and studied the electrochemical intercalation and oxidation process in detail. 43 Even when the reaction time was prolonged (in 11.6 M HClO 4 ), the electrochemical oxidation process did not exhibit a high degree of graphite oxidation (to produce carboxyl groups). In addition, reducing the concentration of HClO 4 (9.2 M and below) further limited the degree of oxidation; in this case, the oxidation group was limited to alcohol and epoxy. Meanwhile, Cao et al. performed two-electrode exfoliation to produce GO with high yield (>70 wt.%), high quality (>90%, monolayer) and reasonable oxygen content (17.7 at.%) through a two-step electrochemical intercalation oxidation method. 44 The as-prepared GO exhibited different characteristics from GO prepared by traditional chemical methods, and these characteristics included low degree of oxidation (oxygen content of 3.2 at.% and C/O ratio of 30.2), few C=O and −COOH, minimal damage to the graphene honeycomb lattice structure and even conductivity of up to 54600 s m −1 . Pei et al. developed a large-scale, ultra-fast, green, safe approach for the preparation of pure GO via water electrolytic oxidation of graphite. 28 They discovered that the pre-intercalation layer of graphite can effectively inhibit the anode electrocatalytic oxygen evolution reaction of water under high pressure and make the graphite lattice oxidize in a few seconds, which is more than 100 times faster than existing methods. The researchers also discussed the preparation mechanism and confirmed the continuous and manipulated preparation of GO and its application in transparent conductive films, strong paper and ultra-light elastic aerogels. Large GO flakes (≥10 μ m and I D /I G = 1.24) were successfully prepared through simple electrochemical peeling of graphite in an electrolyte composed of a mixed solution of H 2 SO 4 and H 3 PO 4 and then oxidized with KMnO 4 . 45 X-ray diffraction (XRD), Raman spectroscopy and Fourier transform infrared (FTIR) study revealed that the GO (6 h) batch had the largest degree of oxidation. The interlayer spacing of the graphene flakes in GO (6 h) was 0.995 nm with an I D /I G ratio of 1.24 and the relative percentage of oxygen-containing functional groups in GO (6 h) calculated with the normalized FTIR diagram was the highest (68.1%).
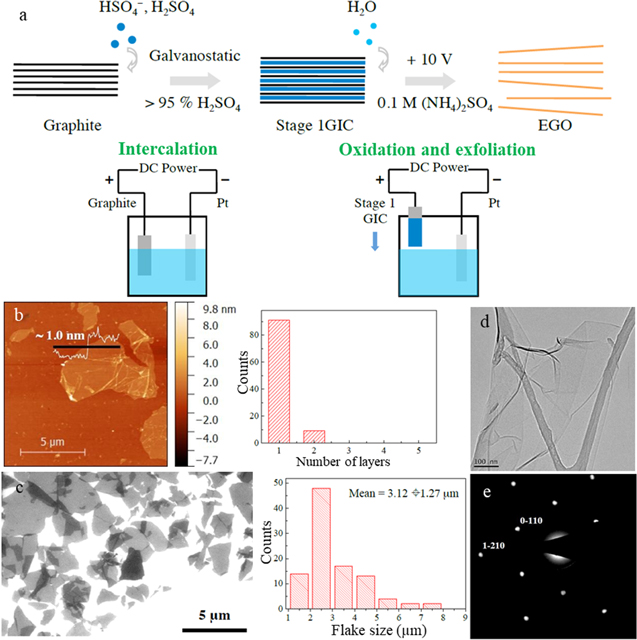
Figure 7. (a) Schematic and mechanism of preparing GO by two-step electrochemical intercalation and oxidation process, (b) AFM and flake sized distribution of GO, (c) SEM and flake sized distribution of GO, TEM (d) and SAED pattern (e) of GO sheet. 44
Campéon et al. proposed a one-step electrochemical intercalation-functionalized graphite foil to prepare GO by using HBF 4 diluted by H 2 O or CH 3 OH as an electrolyte. 46 Owing to the limited gas production, this method has a yield of 99%, thus preventing the destruction of the graphite skeleton and achieving uniform functionalization. The developed system presented two reaction mechanisms: traditional electrolysis in the electrolyte (within the first 5 min of the reaction) and capillary reaction outside the electrolyte (based on the length of the graphite electrode, after 5–60 min). The GOs prepared in H 2 O and CH 3 OH had 49.0 wt.% and 43.9 wt.% oxygen, respectively, which are nearly similar to the percentage for chemical GO prepared with the improved Hummers method (50.2 wt% oxygen). These GOs were prepared as a film for water purification, in which up to 90% of methyl orange could be eliminated. The electrical conductivity of thermally reduced GOs (tEGOs) also reached ∼40,000 S m −1 . At 20 °C, tEGOs were evaluated in the anodes of lithium-ion batteries with a capacity of 195 mAh g −1 , which exhibited 572% enhancement over the original graphite. Highly pure GO was prepared via a two-electrode electrochemical method by using graphite rod extracted from dry cell as a positive electrode, platinum cathode wire as a negative electrode and 0.1 M H 2 SO 4 as an electrolyte. 47 Raman and FTIR spectroscopy confirmed the formation of high-purity GO. The as-prepared GO fabricated from GO-based electric double layer capacitor electrodes had a high capacitance value of 78.82 F g −1 at 20 mV s −1 scan rate and the capacitance value was reduced by 10% after 30 days. Díez-Pascual prepared GO powders with different amounts of surface groups from graphite via an electrochemical exfoliation method. 48 In the case with 98% H 2 SO 4 as the electrolyte, 2 V was applied for 10 min, followed by the use of 20 V for 60 s. An unprecedented minimum carbon-to-oxygen ratio of 1.46 was then obtained. Scanning electron microscopy (SEM) and transmission electron microscopy (TEM) showed that good-quality, large, uniform, well-exfoliated GO sheets were formed. FTIR confirmed the formation of –OH, C–O–C, C=O and molecules on the surface of COOH and pointed out the strong intermolecular interaction between adjacent groups. Furthermore, the samples prepared by applying the first-stage voltage for a long time had high I D /I G , due to the high degree of swelling of the samples. The distance between adjacent slices estimated by XRD was not proportional to the oxygen content. Chen et al. used a strategy of radical oxidation reaction for high-efficiency production of GO via photo-synergetic electrosynthesis (Fig. 8 ). 8 Oxalate anion was used as intercalating ions. The co-reactants increased the effective interfacial concentration of OH•, especially under illumination, thereby enhancing the free radical oxidation reaction of graphite/graphene and OH•· and increasing the oxidation degree of GO, which is equivalent to that of CGO. In addition, crystallinity increased as the number of layers decreased and the grain size increased. The GO membrane co-assembled by aniline selectively permeated the water molecules through hydrogen bonding interactions, but due to electrostatic interactions, Na + , K + and Mg 2+ could not penetrate. The production of a few layers of GO via electrochemical exfoliation was investigated by adding the following custom-made graphene-philic anionic surfactants: a double-tailed surfactant, sodium bis(3,3,3-trimethyl-1-propyl) sulfosuccinate, and a triple-tailed surfactant, sodium 1,4-bis(neopentyloxy)-3-(neopentylcarbonyl)-1,4-dioxobutane-2-sulfonate. 49 To investigate the effect of surfactant chemical structure, the performance of the custom-synthesized surfactants was compared with that of the common single-tail anionic surfactant sodium dodecylsulfate. The results showed that the enhanced methylated and branched chain structures bestowed enhanced adsorption capacity on GO, with up to 82.7% MB removal from aqueous solutions. The summary of GO prepared by the two-electrode exfoliation is shown in Table I . The two-electrode exfoliation method for preparing GO is simple and controllable and can synthesize a large amount of GO quickly and efficiently. However, the two-electrode exfoliation method involves the use of precious metal platinum, which will increase the production cost. Even the use of graphite foil electrodes will increase the number of further processing steps from graphite powder.
Table I. Summary of preparation of GO by two electrodes exfoliation. Acronym definitions: Graphite (GE), Expanded graphite (EG), Pencil cores (PC), Graphite foil (GF), Graphite rod (GR), Graphite slices (GS), Graphite pellet (GP), Na3C6H5O7 (TCD), Ammonium sulfate (AS), oxalate acid (OA), Transparent conductive films (TCF), Strong papers (SP), Ultra-light elastic aerogels (UEA), Lithium-ion battery (LIB), Filtration membrane (FM), Sulfuric acid (SA), Sodium sulphate (SS), Phosphoric acid (PA), Sodium dodecylsulfate (SDS), Sodium bis(3,3,3-trimethyl-1-propyl) sulfosuccinate (AOT-14), sodium 1,4-bis (neopentyloxy)-3-(neopentylcarbonyl)-1,4-dioxobutane-2-sulfonate (TC14).
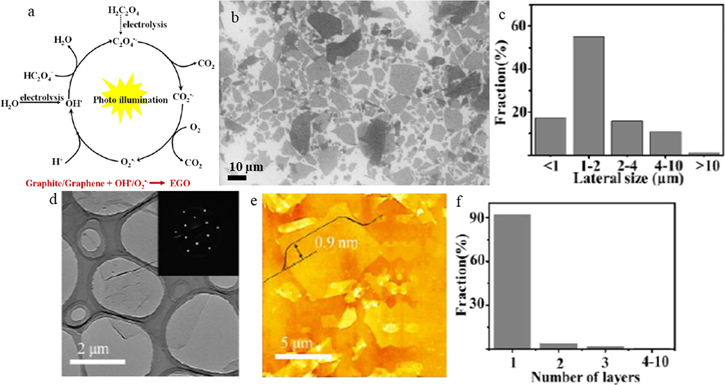
Figure 8. (a) Schematic and mechanism of preparing GO, SEM (b) and flake sized distribution (c) of GO, (d) TEM of GO, AFM (e) and flake sized distribution (f) of GO sheet. 8
Preparation of GO by Three-Electrode Electrochemical Exfoliation
GO preparation by three-electrode exfoliation commonly uses an electrochemical workstation as a power source to control the exfoliation process accurately. In 2011, Zeng et al. developed a new strategy for preparing GO nanosheet-modified electrodes by using the in-situ electrochemical graphite oxide method with a three-electrode device. 56 The exfoliation process was performed with graphite (1 cm 2 ) as the working electrode, platinum (2 cm 2 ) as the counter electrode and saturated calomel electrode as the reference electrode in 0.025 M phosphate buffer at pH 6.9. The modified electrode was suitable for the selective detection of ascorbic acid. In the range of 0.25–1.0 mM, it showed a rapid linear response to ascorbic acid concentration, and the detection limit was 0.5 μ M. The modified electrode could also detect ascorbic acid, uric acid, dopamine and acetaminophen electrochemically at the same time. Under the condition that cetyltrimethylammonium bromide (CTAB) is used as the electrolyte, the three-electrode method of exfoliation can be utilized to obtain GO through a fast, green electrochemical route. 57 After the electrochemical intercalation of CTAB on the graphite working electrode, the graphite electrode intercalated with CTAB was electrochemically exfoliated using electric current as an oxidant. The XRD pattern of the GO contained a broad band in 2 θ limits of approximately 5 and 15 ( d = 0.78 nm) and the thickness of a single-layer GO sheet measured by atomic force microscopy (AFM) was 2.5–4.5 nm. UV–vis absorption experiments showed that electron conjugation can be restored through continuous electrochemical intercalation and stripping processes, and many GO sheets can be obtained. In this work, GO was reduced on the surface of the glassy carbon electrode under ORR conditions, and the number of electrons transferred from the reduced GO surface was calculated to be 2.8. Gurzeda et al. successfully prepared GO by using graphite as the working electrode, platinum wire as the counter electrode and calomel electrode as the reference electrode in 8 M HClO 4 electrolyte. 58 The second-stage HClO 4 –graphite intercalation compound was formed under specific electrochemical oxidation conditions. Further oxidation of the HClO 4 –graphite intercalation compound allowed the researchers to complete the conversion of graphite to obtain the final product (GO). The XRD test results indicated that GO was formed. Moreover, SEM observation and X-ray photoelectron spectroscopy (XPS) analysis, revealed that the formation of GO is selective, and epoxy and alkoxy groups are the main products of this process. Gurzeda et al. used three-electrode exfoliation and linear scanning voltammetry to electrochemically peroxidize natural graphite in 11 M H 2 SO 4 to synthesize highly oxidized GO. 59 The heat treatment of GO leads to a significant reduction of oxygen functional groups; thus, graphene is obtained. Thermally reduced GO has a high specific surface area, the number of mesopores increases and the concentration of edges and surface defects is enhanced. These characteristics are very close to the characteristics of thermally reduced GO prepared by GO synthesized by the Hummers method. In this study, GO was synthesized using a three-electrode exfoliation method with natural graphite flakes in 18 M HNO 3 . 50 Further research showed that the efficiency of GO formation requires balance between acid concentration and the graphite intercalation compound as an intermediate product. The mechanism of exfoliation was also discussed. After exfoliation, the presence of functional groups on GO was confirmed by the XPS and FTIR analysis results. XRD analysis confirmed that the electro-oxidation of the graphene layer increased with d spacing to about 8.7 Å. SEM and TEM observations and Raman spectroscopy results showed that deep oxidation of graphite in 18 M HNO 3 influenced the increase in surface defect concentration.
Wei et al. used a three-electrode exfoliation method to efficiently synthesize GO nanosheets (yield of ∼40 wt. %) through one-step water electrolytic oxidation of glassy carbon (GC) electrode in 1 M H 2 SO 4 solution (Fig. 9 ). 55 After electrochemical exfoliation, GO exhibited a high oxidation degree and good dispersion stability. Its size could be adjusted in accordance with the degree of GC graphitization; hence, GO synthesis with different oxygen functional groups with average sizes of 4, 8, and 13 nm could be controlled. As a metal-free catalyst, 13 nm GO is beneficial to benzylamine oxidative coupling reaction. The conversion rate of 4 nm NGO to benzene oxidation reaction is 88 times higher than that of 13 nm GO. In this study, the electrolytic oxidation mechanism of graphite materials was systematically investigated. The study found that sulfuric acid has a protective effect on the graphite electrode during the electrolytic oxidation of water. A 50 wt.% H 2 SO 4 solution can effectively balance protection and oxidation, whilst producing the highest oxidation efficiency and yield. Chernysheva et al. successfully prepared GO by using the three-electrode exfoliation method with expanded graphite as the raw material. 61 The process of embedding expanded graphite can be divided into two stages. The first stage does not expand the inter-layer distance, and the second stage is accompanied with the simultaneous expansion of the inter-layer distance. In this study, the effective capacitance, characteristic time and acid consumption of expanded graphite foil during electrochemical intercalation were evaluated. An optimal (from an energy efficiency perspective) potential (1150 mV) was proposed, to insert expanded graphite for subsequent electrochemical GO production. SEM, Raman spectroscopy and thermogravimetric analysis were performed to prove the similarity between the synthesized self and GO. The summary of GO prepared by the three-electrode exfoliation is shown in Table II . The results showed that three-electrode synthetic GO exfoliation can precisely control the size, number of layers and loading of the GO sheet on the electrode surface for further application, but the low exfoliating yield is not conducive to industrial production. Similarly, the three-electrode exfoliating method involves the same problems as the two-electrode exfoliating method involving noble metal platinum electrodes and graphite foil electrodes.
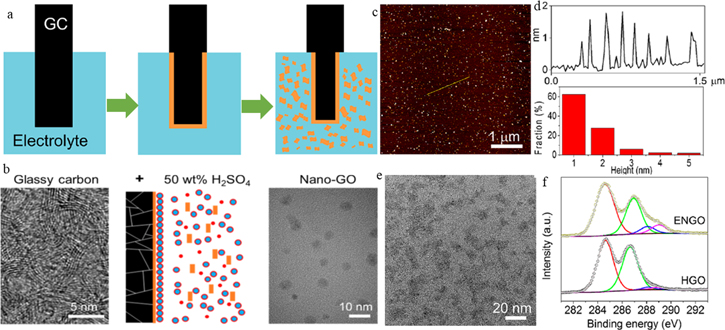
Figure 9. (a) Schematic and (b) mechanism of three electrode electrochemical exfoliation to prepare GO, AFM (c) and flake sized distribution (d) of GO sheet, (e) TEM of GO, (f) XPS of GO sheet. 55
Table II. Summary of preparation of GO by three electrodes exfoliation. Acronym definitions: Graphite (GE), Expanded graphite (EG), Graphite flakes (GFs), Graphite rod (GR), Phosphate buffer solution (PBS), Cetyltrimethylammonium bromide (CTAB), Sulfuric acid (SA), Ammonium sulfate (AS).
Conclusions and Outlook
GO has expanded the practical application of graphene-based materials due to its excellent water solubility and easy functionalization. However, several existing methods have limited applications in actual industrial production because of their serious environmental pollution and unsafe factors. GO preparation by electrochemical exfoliation is a green, efficient, simple, controllable new method that provides the possibility for industrial GO production. This review introduces the principle of electrochemical synthesis of GO and summarizes the latest research progress on GO from three aspects, namely, two-electrode, three-electrode and electrolyte exfoliation, with focalize on the quality and quantity of products. From the previous discussion, we can conclude that the method of exfoliating in the electrolyte is the most likely method to achieve industrial electrochemical preparation of GO (e.g. the preparation method of GO reported by Lowe et al.). However, the two-electrode exfoliation method will increase the cost due to the use of graphite electrodes and noble metal platinum electrodes (e.g. the preparation method of GO reported by Campéon et al.). In addition, the three-electrode exfoliation method is limited by the low yield (e.g. the preparation method of GO reported by Chernysheva et al.). Although some progress has been achieved by theoretical and practical research on electrochemical GO preparation, certain difficulties still restrict the large-scale manufacture of GO.
- (1) Electrochemical synthesis of GO using graphite as a raw material theoretically involves graphite intercalation, oxidation and exfoliation of graphite intercalation. These steps are indirectly confirmed through various characterization techniques. At present, on-site monitoring techniques for electrochemical GO preparation are lacking. Therefore, combining on-site monitoring of electrochemical GO preparation with a theoretical model is helpful in understanding basic mechanism of the process.
- (2) Regardless of the exfoliation procedure used for GO preparation, the precious metal platinum is used as the electrode, which adversely affects the actual industrial production control cost. This issue needs to be resolved in subsequent research.
- (3) The precursor of GO prepared by electrochemical exfoliation should use graphite powder, which is widely adopted in industries under a suitable exfoliating device. It can avoid the further processing of graphite foil and graphite rod.
- (4) A suitable electrolyte must be selected for electrochemical exfoliation. However, most of the electrolytes currently used to prepare GO have serious problems, such as insufficient oxidation, insufficient intercalation and difficulty in removing the electrolyte.
- (5) The separation and purification EGO from the as-produced product (mixture of un-exfoliated graphite and EGO) is one of the major challenges to solve before actual industrial production.
- (6) Electrochemical GO preparation involves many factors, such as working electrode, electrolyte, intercalation agent, power supply, electrolytic stripping device and other operating conditions. Electrochemical exfoliation can be further combined with ultrasonic exfoliation, photochemical exfoliation and other technologies to improve the quality and efficiency of GO preparation.
Acknowledgments
This work was financially supported by the Youth talent support program of science and technology association of colleges of Shaanxi Province (grant nos. 20190609), by the Special scientific research plan of education department of Shaanxi province (Grant Nos. 19JK0190, 18JK0171 and 20JK0556), by the Natural Science Foundation of Shaanxi Province (Grant Nos. 2019JQ508), by the Open project of Key laboratory of catalytic foundation and application of Shaanxi province (grant nos. SLGPT2019KF01-23), by the Opening Foundation of Shaanxi Key Laboratory of Industrial Automation (No. SLGPT2019KF01-10), by the School-level project of Shaanxi university of Technology (grant nos. SLGRC03, SLGRC02 and SLGKY2011).
Click through the PLOS taxonomy to find articles in your field.
For more information about PLOS Subject Areas, click here .
Loading metrics
Open Access
Peer-reviewed
Research Article
Fabrication and Characteristics of Reduced Graphene Oxide Produced with Different Green Reductants
Contributed equally to this work with: Changyan Xu, Xiaomei Shi
* E-mail: [email protected]
Affiliation Packaging Engineering Department, Nanjing Forestry University, Nanjing, Jiangsu, China
Current address: Packaging Engineering Department, Nanjing Forestry University, Nanjing, Jiangsu, China
¶ ‡ These authors also contributed equally to this work.
- Changyan Xu,
- Xiaomei Shi,
- An Ji,
- Lina Shi,
- Chen Zhou,
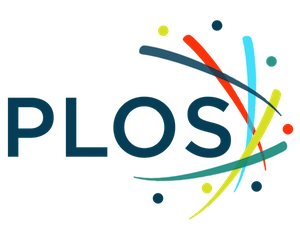
- Published: December 14, 2015
- https://doi.org/10.1371/journal.pone.0144842
- Reader Comments
There has been an upsurge of green reductants for the preparation of graphene materials taking consideration of human health and the environment in recent years. In this paper, reduced graphene oxides (RGOs) were prepared by chemical reduction of graphene oxide (GO) with three green reductants, L-ascorbic acid (L-AA), D-glucose (D-GLC) and tea polyphenol (TP), and comparatively characterized by X-ray photoelectron spectroscopy (XPS), Fourier transform infrared (FTIR) spectra, Raman spectra and electrical conductivity analysis. Results showed that all these three reductants were effective to remove oxygen-containing functional groups in GO and restore the electrical conductivity of the obtained RGO. The RGO sample with L-ascorbic acid as a reductant and reduced with the existence of ammonia had the highest electrical conductivity (9.8 S·cm -1 ) among all the obtained RGO samples. The mechanisms regarding to the reduction of GO and the dispersion of RGO in water were also proposed. It is the good dispersibility of reduced graphene oxide in water that will facilitate its further use in composite materials and conductive ink.
Citation: Xu C, Shi X, Ji A, Shi L, Zhou C, Cui Y (2015) Fabrication and Characteristics of Reduced Graphene Oxide Produced with Different Green Reductants. PLoS ONE 10(12): e0144842. https://doi.org/10.1371/journal.pone.0144842
Editor: Bing Xu, Brandeis University, UNITED STATES
Received: August 5, 2015; Accepted: November 24, 2015; Published: December 14, 2015
Copyright: © 2015 Xu et al. This is an open access article distributed under the terms of the Creative Commons Attribution License , which permits unrestricted use, distribution, and reproduction in any medium, provided the original author and source are credited
Data Availability: All relevant data are within the paper.
Funding: The first Project name is “A Project Funded by the Priority Academic Program Development of Jiangsu Higher Education Institutions (PAPD).” It can be accessed at http://jsycw.ec.js.edu.cn/ . The funding institution is The Education Department of Jiangsu Province. The second Project name is “Research and popularization of key techniques of functional electromagnetic shielding composites (Grant No. BC2013432).” It can be accessed at http://210.73.128.105/cpsb/CPSB/login.jsp?typ=nolog . The funding institution is Science and Technology Department of Jiangsu Province. CYX received these two fundings. The third Project name is “Research and innovation project of the academic degree graduate students in Jiangsu Province (KYLX15_0935).” It can be accessed at http://gsnfu.njfu.edu.cn/html/69/13965.html . The funding institution is Nanjing Forestry University. XMS received this funding. The funders had no role in study design, data collection and analysis, decision to publish, or preparation of the manuscript.
Competing interests: The authors have declared that no competing interests exist.
Introduction
Graphene, a burgeoning material with a honeycomb-like two-dimensional crystal structure formed by carbon atoms in sp 2 hybrid junction, exhibits incomparable electronic, thermal, and mechanical properties [ 1 – 3 ]. It has been paid much attention since its first appearance in 2004 when Geim et al. obtained one atomic layer thickness graphene from graphite by peeling with adhesive tape [ 4 ]. Graphene presents great potential applications in various fields, including electrodes [ 5 ], supercapacitors [ 6 ], composite materials [ 7 – 8 ] and sensors [ 9 ]. However, it is still a bottleneck to produce high-quality graphene on a large scale and at low cost so as to put graphene into practical applications effectively. There are several methods to obtain graphene, such as mechanical exfoliation [ 4 , 10 ], epitaxial growth [ 11 ], liquid-phase exfoliation of graphite [ 12 – 13 ], chemical vapor deposition [ 14 – 15 ], as well as chemical reduction of graphene oxide (GO) [ 16 – 18 ]. Among all these processes, chemical reduction of GO has been regarded as the fastest way to produce graphene in large quantities, and it also has an advantage of solution-processable [ 19 ]. This technique involves two steps [ 20 ]. First, graphite oxide is obtained by oxidation of graphite powder using the Staudenmaier process or Hummer’s method [ 21 – 22 ]. Second, graphite oxide is exfoliated to graphene oxide by ultrasonication and then various reductants were used to obtain reduced graphene oxide (RGO). Hydrazine [ 23 – 24 ], sodium borohydride and its derivatives [ 20 ], oxalic acid [ 25 ], as well as sodium hydrosulfite [ 26 ] have been reported as reductants to remove oxygen-containing functional groups of GO and thus to restore the conjugate structure of graphene. Considering the ease for functionalization and industrial scale production, as well as the suitability for applications in polymer composites and graphene conductive ink, this paper focused on the solution processable graphene prepared by green reductants. In the next stage, polymer composites and graphene conductive ink will be fabricated and characterized with the obtained RGO.
Some researches confirmed that hydrazine and sodium borohydride had the ability to remove oxygen functionalities of GO. Tung et al. [ 23 ] prepared chemically converted graphene sheets with the largest area reported to date (up to 20×40 mm) by reducing GO with pure hydrazine as a reductant. Shin et al. [ 27 ] compared the reducing effect of hydrazine and sodium borohydride, and found that with regard to the dose of reductants, 10 mM sodium borohydride was more useful than 50 mM hydrazine for preparation of RGO from GO in lowering the sheet resistance of RGO. However, both hydrazine and sodium borohydride are highly toxic to human beings as well as the environment. In addition, the hydrophobic nature of obtained reduced GO sheet would lead to irreversible agglomerate of RGO in solutions under van der Waals interactions [ 28 ]. In recent years, much attention has been paid to exploring environmentally friendly reductants for production of graphene from graphite oxide with good dispersibility in solvents. L-ascorbic acid (L-AA), D-glucose (D-GLC) and tea polyphenol (TP) are water-soluble and mild reductants. The first innocuous and safe reductant, which was comparable to hydrazine as to the deoxygenation of GO, was ascorbic acid (vitamin C), reported by Fernández-Merino et al. [ 29 ]. Also, Zhu et al. [ 30 ] successfully synthesized chemically converted graphene nanosheets with reducing saccharides (glucose, fructose and sucrose) as reductants. Wang et al. [ 19 ] proposed a facile method based on TP to reduce the exfoliated GO in a green tea solution. Considering the operability, low cost and harmlessness to environment, it is very urgent to optimize the reduction process parameters of GO reduction. He et al. [ 31 ] compared the reduction rate, density of small sp 2 domains, degree of reduction, and stability of the reduced GO suspension of L-ascorbic acid, D-fructose, glucose, sucrose, and Na 2 SO 3 . However, there’s no open reports referring to comparative studies of deoxygenation of GO by L-AA, D-GLC and TP. In addition, no comparative researches in open literatures were about the electronic properties of RGOs prepared by these environmental friendly reductants, which is the most important characteristic index for graphene and is the indicator to evaluate the efficiency of GO reduction [ 32 ].
In this paper, RGOs were produced from GO, which was synthesized firstly according to the modified Hummer’s method, and then reduced by three green reductants, L-AA, D-GLC and TP. The role of ammonia in L-AA and D-GLC reduction processes was also observed. Reduction processes and electrical properties of RGOs were comparatively investigated by XPS, FTIR spectra, Raman spectra and electrical conductivity analysis. Besides, considering that little literature was about the reduction mechanism of GO by environmentally friendly reductants, we also discussed possible reduction mechanisms of GO by L-AA, D-GLC and TP and their dispersion mechanisms in water with the intention of opening a new perspective in the research of graphene-based composite materials and graphene conductive ink.
Materials and Methods
Natural graphite powder (40 μm) was obtained from Qingdao Henglide Graphite Co., Ltd. Concentrated sulfuric acid (H 2 SO 4 , 98%), Potassium permanganate (KMnO 4 ), Hydrogen peroxide (H 2 O 2 , 30%), Hydrochloric acid (HCl), and ammonia solution were purchased from Nanjing Chemical Reagent Co., Ltd. L-ascorbic acid (L-AA), D-glucose (D-GLC), Potassium persulfate (K 2 S 2 O 8 ) and Phosphorus pentoxide (P 2 O 5 ) were purchased from Sinopharm Chemical Reagent Co., Ltd. Tea polyphenol (TP, purity of 80%) was generously provided by Ningbo Sinorigin Bio-Products Co., Ltd. All chemicals are of analytical grade and used as received without further purification. Deionized water was prepared by a Milli-Q Plus system (Millipore).
Synthesis of GO
GO was prepared by the modified Hummers’ method [ 22 , 33 ]. Briefly, graphite powder (10 g) was mixed with K 2 S 2 O 8 (8.4 g), P 2 O 5 (8.4 g) and H 2 SO 4 (60 mL) in a beaker. The mixture was heated to 80°C and kept for 5 h under vigorous stirring. After cooling to room temperature by adding distilled water, the mixture was vacuum-filtered to wash by deionized water on a 0.22 μm Nylon membrane until the insoluble substance was neutral. The solid was vacuum-dried overnight. Then the obtained insoluble matter (4 g) was moved into a beaker filled with H 2 SO 4 (240 mL) in an ice bath. After slowly adding KMnO 4 (30 g) under continuous stirring while keeping the temperature below 10°C, the mixture was then heated to 35°C with vigorous stirring and kept for 4 h, followed by being diluted with distilled water (0.5 L) and kept at 50°C for 2 h. Successively, deionized water (1 L) was again added into the mixture, followed by a dropwise addition of H 2 O 2 (20 mL) under vigorous stirring. With the gradual addition of H 2 O 2 , the color of the mixture gradually turned from dark brown into brilliant yellow. After being filtered with HCl (5 wt%) for 3 times while it was hot, the undissolved substance was washed with deionized water until it was neutral, resulting in graphite oxide. In order to remove the residual salts and acids, the resulting graphite oxide was dialyzed with a molecular weight cut-off membrane (MW 3500 Da) with deionized water for at least 2 weeks at room temperature. Exfoliation of graphite oxide to GO sheets was performed by sonicating the graphite oxide dispersion for 2 h (XO-1200, Xianqu Biological Technology Co., Ltd., China) in the condition of 20 kHz and 80 W. After centrifugation (H-1650, Hunan Xiangyi Laboratory Instrument Development Co., Ltd., China) at 4000 rpm for 30 min to remove the unexfoliated GO sheets, homogeneous GO dispersion was obtained.
Chemical reduction of GO
L-AA (300 mg) and the obtained GO suspension (300 mL, 0.1 mg·mL -1 ) were mixed, and then ammonia solution (20 μL, 25% w/w) was added to adjust the pH of the suspension to 9–10 to give full play of the colloidal stability of GO sheet through electrostatic repulsion in alkaline conditions [ 34 ]. After being sonicated for 30 min, the suspension was heated to 95°C under vigorous stirring and kept for 2 h, resulting in RGO. The produced RGO, designated as L-AA RGO-1, was then centrifuged at 3000 rpm for 15 min, followed by the wash process with distilled water until it was neutral.
The procedure for preparing RGO with D-glucose as a reductant, designated as D-GLC RGO-1, was the same as that for L-AA RGO-1.
The reduction processes without the addition of ammonia solution were also carried out. The resulting RGOs were designated as L-AA RGO-2 and D-GLC RGO-2, respectively.
In order to investigate the role of ammonia solution in the reduction process of GO, ammonia solution (25% w/w) was added to GO suspension (300 mL, 0.1 mg·mL -1 ) to adjust the pH to 9–10. Then the suspension was heated to 95°C under vigorous stirring and kept for 2 h, and the resulting RGO was designated as N RGO. After cooling to room temperature, the suspension was washed with distilled water until it was neutral.
However, the process for the sample reduced by TP, designated as TP RGO, was different. It has been reported that polyphenols are highly sensitive to high temperature and alkaline pH, which will deteriorate the activity of polyphenols [ 35 ]. Therefore, ammonia was not used in this case and the reaction temperature was lower than that of L-AA and D-GLC reactions in this paper. Referring to Liao’s method [ 36 ], TP (90 mg) was added to GO suspension (300 mL, 0.1 mg·mL -1 ), and then being sonicated for 30 min. After that, the mixture was maintained at 80°C for 8 h. After cooling to the ambient temperature, the resulting suspension was washed with deionized water by filtering through a nylon membrane to remove the residual TP. During this washing process, the faded color of the filtrate suggested the removal of the TP.
As shown in Fig 1 , it should be noted that except D-GLC RGO-2 (e), the colors of RGOs changed from brown to black, which was the evidence of the reduction process converting GO into RGO [ 37 ], and L-AA RGO-2 (c) was agglomerated. The phenomena will be discussed later in this article.
- PPT PowerPoint slide
- PNG larger image
- TIFF original image
https://doi.org/10.1371/journal.pone.0144842.g001
Characterization
GO, L-AA RGO-1, L-AA RGO-2, D-GLC RGO-1, D-GLC RGO-2, N RGO and TP RGO sample were freeze-dried and then grounded into powder. XPS spectra of graphite, GO and RGO samples were recorded on an AXIS UltraDLD (SHIMADZU, UK) with an Al Kα radiation (1486.6 eV). The FTIR spectra of graphite, GO and RGO samples were observed by a Nicolet iS10 spectrometer (Thermo Scientific Inc., USA) from 4000 cm -1 to 500 cm -1 at a resolution of 4 cm -1 . Raman spectra of graphite, GO and RGO samples were observed from 800 cm -1 to 2000 cm -1 on a Laser Micro-Raman spectroscope (DXR532, Thermo Scientific Inc., USA) using 532 nm He-Ne laser excitation. The electrical conductivities of graphite, GO and RGO samples were tested on a four-point probe system (RTS-8, Guangzhou Four-Point Probes Technology co., Ltd., China). All samples were tableted into “papers” (1 cm in diameter, 0.3 mm in thickness) by a tablet press (YP-2, Shanghai Shang Yue Scientific Instrument co., Ltd., China) under a pressure of 15Mpa, followed by being vacuum-dried at 80°C for 1 h. Electrical conductivity measurements were repeated on at least 3 different areas of the “papers”.
Results and Discussion
Chemical reduction degree of go.
XPS is generally used to investigate chemical reduction degree of GO in detail [ 38 ]. The C1s XPS spectra of graphite, GO and RGO samples were shown in Fig 2 . In Fig 2 , the spectrum of graphite presented one sharp peak corresponding to C-C stretching at 284.5 eV, suggesting that no oxygen containing groups exist in pristine graphite. While the spectrum of GO sample presented four deconvolution peaks, corresponding to the C = C/C-C in aromatic rings (284.5 eV), epoxy C (C-O, 286.8 eV), carbonyl C (C = O, 287.8 eV) and carboxyl C (-COOH, 289.0 eV), respectively [ 32 ]. For the RGO samples reduced by L-AA with or without the addition of ammonia, the intensity of peaks assigned to oxygen-containing functional groups decreased significantly after reduction, indicating a considerable deoxygenation of GO. However, the intensity of COOH in the C1s spectrum of L-AA RGO-2 was higher than that of GO. When L-AA was used as a reductant without the addition of ammonia, the COOH group in decomposition product of L-AA (oxalic acid) [ 39 ] cannot be neutralized by ammonia, which will be responsible for a higher intensity of COOH in L-AA RGO-2. Despite some oxygen group peaks existing in the C1s spectra of D-GLC RGO-1, N RGO and TP RGO, the peak intensity was greatly smaller than that of the GO sample. It should not be neglected that there’s no significant difference between the spectrum of D-GLC RGO-2 and GO, demonstrating that weak reduction was implemented by D-GLC in the absence of ammonia. In addition, it’s obvious to find that the peak intensity of carboxyl C in the spectrum of D-GLC RGO-1 was much higher than that of D-GLC RGO-2. The oxidization of the aldehyde group in glucose by GO in alkaline solution can produce carboxyl group [ 30 ], which further verified that the existence of ammonia can allow full play to the reducibility of D-GLC.
https://doi.org/10.1371/journal.pone.0144842.g002
In the XPS study of carbon materials, FWHM (Full Wave at Half Maximum) of C = C FWHM (C = C) is usually negatively related to the conductivity of the materials, that is the lowest FWHM (C = C) corresponding to the highest conductivity [ 40 ]. Table 1 showed the values of FWHM (C = C) of graphite, GO and RGO samples. The decrease of FWHM (C = C) after reduction indicated the increase in conductivity of the samples by restoration of sp 2 network. Meanwhile, the obvious intensity decrease of the peak C-O in the spectra of RGO samples in Fig 2 also implied the increase in conductivity, which is in line with Obata’s research [ 32 ].
https://doi.org/10.1371/journal.pone.0144842.t001
In XPS investigation, the change of C/O atomic ratio of RGO suggests the reduction degree [ 38 ]. As shown in Table 1 , the ratio derived from the XPS data showed various degree of increases after reduction, demonstrating that most oxygen-functionalized groups were removed during reduction process. As for graphite, its C/O atomic ratio was 17.87, which was in agreement with the report by Moon et al. [ 41 ]. The C/O atomic ratios of L-AA RGO-2 and D-GLC RGO-2 were a little lower than that of their corresponding RGO-1. In the absence of ammonia, deoxygenation of GO by L-AA and D-GLC was less effective, which was consistent with the observation that intensities of C-O and C = O peaks in RGO-2 were higher than those in RGO-1. And RGOs reduced by L-AA with or without the addition of ammonia showed much higher C/O atomic ratio than the other RGOs, which indicating that a better deoxygenation of GO can be achieved by L-AA. However, C/O atomic ratio of L-AA RGO-1 (5.15) was lower than that of RGO reduced by vitamin C (~12.5) [ 29 ]. It can be deduced that the difference of C/O atomic ratio may be ascribed to not only a few differences between the production processes of GO in two researches, but also the different content of GO used in the reduction process. Some oxygen groups remaining on the RGO surface may be benefit for chemical functionalization in preparation of composite materials and conductive ink in the next stage. For all RGO samples in this research, C/O atomic ratios were lower than that of RGO reduced by hydrohalic acid (about 12) [ 16 ]. However, hydrohalic acid is corrosive and harmful to human being and the environment. Some residual oxygen groups still exist in RGOs, showing the intrinsic defect of RGO. This result was consistent with the former report [ 29 ].
Changes of functional groups in the reduction process
FTIR spectroscopy is regarded as an important tool for characterization of functional groups [ 42 ]. The FTIR spectra of graphite and its corresponding GO, RGOs with different preparation methods were shown in Fig 3A , and the specific comparative FTIR spectra of RGOs prepared with or without ammonia and N RGO were shown in Fig 3B and 3C . In Fig 3A , the characteristic peaks at 3223, 1727, 1620, 1368, 1221, and 1046 cm -1 in the GO spectrum (b) were due to O-H stretching, C = O stretching, aromatic C = C stretching, O-H deformation, epoxy C-O stretching, and alkoxy C-O stretching vibrations [ 30 , 43 ], respectively. These peaks did not appear in the spectrum of graphite sample (a), indicating that the oxidation step introduced a large number of oxygen-containing functional groups, and these groups should include -COOH and C = O located at the sheet edge, -OH and epoxy C-O on the basal planes of the GO sheet [ 44 ]. After reduction by L-AA with the addition of ammonia (c), almost all the characteristic peaks weakened and some even disappeared, which was consistent with the result of the XPS analysis in this paper. The curve of L-AA RGO-1 was similar to that of graphite, showing the restoration of electronic conjugation within graphene sheets. In the spectra of D-GLC RGO-1 (d) and TP RGO (f), the intensity of peaks at 3223, 1727, and 1368 cm -1 decreased dramatically, demonstrating the removal of oxygen-containing functional groups to a certain degree. In the spectrum of N RGO (e), the intensity of oxygen-containing functional groups decreased to some extent, and this result was consistent with Fan’s report [ 34 ]. Besides, the existing C = C peak at 1620 cm -1 in the spectra of all RGO samples suggested that the sp 2 structure of carbon atoms was remained, and this result was in line with the finding of SU et al. [ 45 ]. In Fig 3B , no significant differences presented between the spectra of L-AA RGO-1 (c) and L-AA RGO-2 (g). It demonstrated that the addition of ammonia in suspension had little influence on the functional groups of resulting RGO; however, the existence of ammonia would prevent the agglomeration of graphene sheets to a great extent, which was proved by the well-dispersed suspension of L-AA RGO-1 in Fig 1 . In Fig 3C , the intensity of oxygen-containing functional groups in D-GLC RGO-1 (d) decreased more considerably than that in D-GLC RGO-2 (h), indicating the weak reducibility of D-GLC in the absence of ammonia. Considering this result and the different colors of D-GLC RGO-1 and D-GLC RGO-2 shown in Fig 1 , it can be deduced that the synergistic effect caused by the introduction of ammonia benefited the deoxygenation of GO, and this result was in line with the result of the XPS analysis in this paper and previous researches [ 30 , 34 ]. So, a conclusion can also be drawn that the reducing ability of L-AA was the highest among all the used reductants according to the removal of oxygen-containing functional groups, and the addition of ammonia would prevent resultant RGO from agglomerating.
https://doi.org/10.1371/journal.pone.0144842.g003
Raman spectroscopy investigation of the samples
Raman spectroscopy is one of the most common and effective techniques for analyzing the structure changes of graphene-based materials, including disorder and defect structures, defect density, and doping levels [ 30 , 46 ]. Two prominent features are usually observed in the Raman spectra of graphene, namely the G band (~1580 cm -1 ) and the D band (1270–1450 cm -1 , depending on laser wavelength [ 47 ]). The G band, relating to the graphite carbon structure, is corresponding to the first order scattering of E 2g phonon of sp 2 C atoms at the Brillouin zone center, while the D band, indicating typical defects attributed to the structural edge effects, is arising from a breathing mode of rings or K-point photons of A 1g symmetry [ 3 , 30 , 48 ]. Fig 4 showed the Raman spectroscopy spectra of graphite, GO and RGOs prepared with different reductants. Compared with the spectrum of graphite, a broad G band and a blue-shift (from 1565 cm -1 in the spectrum of graphite to 1584 cm -1 in that of GO) were observed in the spectrum of the GO sample because of the isolated double bonds resonate at higher frequencies than the G band of graphite [ 49 ], indicating that the oxidation took place. Meanwhile, the intensity of D band (1344 cm -1 ) increased dramatically, which was ascribed to the extensive oxidation introducing defects in graphite [ 50 ]. After reduction, the spectra of RGO samples with different reductants had similar peaks, however they showed different intensity ratio of the D to G bands (I D /I G ), which is usually an important parameter to characterize the disorder or the extent of covalent modification of the graphene surface [ 51 ]. Different I D /I G values of GO and RGO samples were shown in Table 2 . Compared to GO, all RGO samples except D-GLC RGO-2 presented increased I D /I G values. It indicated that the reduction step removed most of the oxygenated functional groups and a reduction in the average size of sp 2 domains [ 43 , 52 ]. L-AA RGO-1 sample had the highest I D /I G value (1.19), showing that the reduction process using L-AA RGO as a reductant with the addition of ammonia produced the most abundant and smallest graphitic domains among all samples. This finding was in accordance with the result of He et al. [ 31 ]. The lower I D /I G value of D-GLC RGO-2 indicated that insufficient reduction was carried out without the addition of ammonia, which was consistent with the XPS and FTIR analysis in this paper and the report of Venkanna [ 53 ]. Loryuenyong et al. [ 54 ] reported that they prepared RGO with I D /I G value of 1.12 only by stirring and heating GO in distilled water at 95°C for 4 days. Herein when L-AA was used as a reductant, it only took 2 hours to reduce GO and the I D /I G value of the resulting L-AA RGO-2 was up to 1.17, showing that L-AA as a reductant to prepare RGO can largely save time and energy. As regards D-GLC, a similar result was obtained. The I D /I G value of the RGO produced only using D-GLC (D-GLC RGO-2) was 0.86; however, with the help of synergistic effects caused by the introduction of ammonia, the I D /I G value of the RGO (D-GLC RGO-1) was increased to 1.1, which was close to that of the RGO fabricated by Loryuenyong et al. The increasing values of I D /I G for all RGO samples compared with GO’s in our experiment were lower than those of He’s finding, which demonstrated that the RGO samples produced in this experiment were less-defected, corresponding to the disorder characteristic for the D band. Also, L-AA RGO samples had better restoration of sp 2 network than RGOs reduced by D-GLC and TP in reference to XPS analysis. Therefore, L-AA is the most effective reductant for GO to obtain RGO among the used reductants in this study.
https://doi.org/10.1371/journal.pone.0144842.g004
https://doi.org/10.1371/journal.pone.0144842.t002
Electrical conductivity of the obtained reduced graphene oxide
Previous research has revealed that GO shows electrical insulation and chemical reduction can remove oxygen-containing functional groups to regain electrical conductivity of RGO [ 49 ]. Electrical conductivity of reduced graphene oxide has usually been used to indicate the restoration extent of electronic conjugation in deoxidating GO [ 29 ]. As shown in Table 3 , the electrical conductivity of GO was quite low, and 5 orders of magnitude lower than that of graphite, indicating its electrical insulation. Meanwhile, there was a great disparity among the conductivity values of RGO samples prepared by different reductants, revealing that the conductivity of the obtained reduced graphene oxide was greatly influenced by reduction process and reductants. This result was in good agreement with the statement of Zhao et al. [ 55 ]. In our experiment, the L-AA RGO-1 showed the best conductivity among all the obtained RGO samples, 9.8 S·cm -1 , which was much higher than that of RGO sample produced by Gao et al. (0.141 S·cm -1 ) [ 37 ]. The addition of ammonia provided the possibility of GO deoxygenating to some extend [ 34 ], which will be beneficial to the increment of electronic conductivity of resulting RGO. In addition, L-tryptophan, which Gao et al. used as a stabilizer to avoid the agglomeration and precipitation of the resulting graphene sheets, would be absorbed on the surface of reduced graphene oxide, resulting in the degradation of electronic property of the obtained RGO. As for L-AA RGO-2 prepared without the addition of ammonia, the electrical conductivity was a bit lower than that of L-AA RGO-1. Under the condition of no electrostatic stabilization provided by alkali media, RGO would aggregate and the aggregation would further hinder the complete interconnection between conductive paths, resulting in weaker electronic conductivity. In Table 3 , the D-GLC RGO-2 sample presented the lowest conductivity value compared with other samples due to the weak reducibility of D-GLC without the synergistic effect offered by ammonia. This phenomenon was discussed in the XPS, FTIR and Raman analysis in this research. Meanwhile, the impressive electronic conductivity of N RGO (3.73) also proved Fan’s opinion that simply heating GO suspension under strongly alkaline conditions can be a green route to prepare graphene [ 34 ]. The conductivity value of TP RGO was 1.36 S·cm -1 , pretty lower than that of RGOs prepared by L-AA, D-GLC (with the addition of ammonia) and ammonia. Wang et al. [ 19 ] believed that the adsorbed TP molecules on the graphene surface limited the conductivity of TP RGO. In addition, the conductivity value, 1.36 S·cm -1 , was comparatively lower than that of Liao (23.85 S·cm -1 ) [ 36 ], which may be caused by the fact that the purity of tea polyphenol they used was higher than ours. Furthermore, I D /I G value of TP RGO in this paper was lower than that of Liao, indicating less oxygenated functional groups were removed in our case, corresponding to lower electrical conductivity. When it comes to the restoration of the π-conjugated structure, D-GLC (with the addition of ammonia) was somewhat more efficient than TP, but not as good as L-AA. This result was in line with the findings of XPS, FTIR analysis as well as Raman analysis in our experiment.
https://doi.org/10.1371/journal.pone.0144842.t003
Possible reduction mechanism of GO and dispersion mechanism of RGO
Fig 5 represented the possible reaction mechanism of GO reduced by L-AA. On the basis of aforementioned XPS and FTIR analysis, hydroxyl and epoxide groups on the basal planes of the GO sheets eliminated, indicating that those groups reacted with hydrogen atoms in the 5-membered ring of L-AA to yield H 2 O. During the reduction process, SN2 nucleophilic attack and subsequent thermal elimination will restore the C = C bond, which indicates that graphene oxide has been converted to reduced graphene. And acidic L-AA is easy to deprotonate to form dehydroascorbic acid [ 56 ], which will further be converted into guluronic acid and oxalic acid [ 39 ]. And the intermediate acids can be neutralized by ammonia to prevent the accumulation of dehydroascorbic acid products so as to facilitate the reduction process [ 31 ]. Meanwhile, the alkaline condition may offer electrostatic repulsion between RGO sheets [ 34 ] and the intermediates may form hydrogen bonds with the residual oxygen groups, including -COOH on the edge of the GO sheets, which will hinder the π-π stacking between the RGO sheets and prohibit the formation of aggregations, resulting in a stable L-AA RGO suspension [ 39 ].
https://doi.org/10.1371/journal.pone.0144842.g005
The reduction mechanism of TP is similar to that of L-AA. Epigallocatechin gallate (EGCG), accounting for 50–60 wt% of TP [ 19 ], is the most abundant component in TP and gives TP the unique reducibility. As shown in Fig 6 , through an SN2 nucleophilic attack, the oxygen anion of EGCG will open the ring of epoxide on GO sheets, which was proved by the phenomenon that the intensity of epoxy C was decreased in XPS analysis. And a backside SN2 nucleophilic attack will form intermediate and yield H 2 O, resulting in reduced graphene and galloyl-derived orthoquinone after thermal elimination. The oxidized TP strongly absorbed on RGO leading to π-π interactions, which is responsible for the stability of TP RGO suspension [ 36 ].
https://doi.org/10.1371/journal.pone.0144842.g006
Different from L-AA and EGCG, D-GLC cannot deprotonate to form nucleophile. With the existence of both aldehyde group and hydroxyl group in its molecule, D-GLC can form a ring hemiacetal in the interior of the molecule. Therefore, the ring-like structure and the open chain structure coexistence in D-GLC solution. As shown in Fig 7 , the reducibility of D-GLC lies in aldehyde group on the open chain structure, which can be oxidized to carboxyl group by GO in the presence of ammonia. In this case, GO can be seen as the oxidant, and ammonia played the role of stimulator to oxidization process. This process turns glucose into aldonic acid, which can be converted into lactone containing a large number of hydroxyl groups and carboxyl groups, corresponding to the increasing peak intensity of carboxyl C in D-GLC RGO-1 in XPS analysis. The oxidized products of D-GLC can form hydrogen bonds with residual oxygen groups on the GO sheets, preventing the formation of agglomerates, thus resulting in stable D-GLC RGO suspension [ 30 ].
https://doi.org/10.1371/journal.pone.0144842.g007
Conclusions
There has been an upsurge of environmentally friendly reductants for preparation of graphene materials. In this research, the reducibility of three green reductants, L-ascorbic acid, D-glucose and tea polyphenol, for graphene oxide was comparatively investigated. The process involved preparation of graphene oxide and its chemical reduction with different reductants to obtain reduced graphene oxide. XPS, FTIR, Raman and electrical conductivity analysis showed that all reductants except D-GLC without the addition of ammonia were effective to convert GO into RGO. The RGO sample using L-ascorbic acid as a reductant with the existence of ammonia had the highest electrical conductivity, 9.8 S·cm -1 , among all the obtained RGO samples. The existence of ammonia in the reduction process of L-AA provides the possibility of deoxygenation in GO to some extend and prevents the agglomeration of resulting RGO sheets. However, in the reduction process of D-GLC, ammonia offers the synergistic effect to stimulate to the oxidization process, so as to give full play to the activity of aldehyde group on the open chain structure in D-GLC. Possible reduction mechanisms of GO by L-AA and TP are due to SN2 nucleophilic reaction, while the aldehyde group on the open chain structure of D-GLC gives D-GLC the unique reducibility. The good dispersibility of resulting RGOs in water will facilitate the further use of graphene in a larger range of possible applications.
Acknowledgments
This work was financially supported by “A Project Funded by the Priority Academic Program Development of Jiangsu Higher Education Institutions (PAPD)”, “Research and popularization of key techniques of functional electromagnetic shielding composites (Grant No. BC2013432)” and “Research and innovation project of the academic degree graduate students in Jiangsu Province (KYLX15_0935).”
Author Contributions
Conceived and designed the experiments: CYX XMS. Performed the experiments: XMS AJ LNS CZ. Analyzed the data: XMS CYX. Contributed reagents/materials/analysis tools: AJ YQC. Wrote the paper: XMS.
- View Article
- PubMed/NCBI
- Google Scholar
- 56. Marks J. The Vitamins:Their Role in Medical Practice. 1st ed. Springer, New York:1985.

Environmental Science: Nano
Enhanced plasmid-mediated conjugative transfer of resistance genes across bacterial species promoted by graphene oxide †.

* Corresponding authors
a Key Laboratory of Pollution Exposure and Health Intervention of Zhejiang Province, Interdisciplinary Research Academy (IRA), Zhejiang Shuren University, Hangzhou, China E-mail: [email protected]
b College of Environmental Science and Engineering, Zhejiang Gongshang University, Hangzhou, China
The rapid proliferation of antibiotic resistance genes (ARGs) in the environment has become a major concern. However, the effect of graphene oxide (GO), a newly recognized pollutant, on the transfer of ARGs among bacteria remains poorly understood. In this study, we investigated the influence of GO at concentrations ranging from 25 to 400 mg L −1 on the frequency of conjugative transfer of ARGs mediated by the RP4 plasmid. Our results demonstrated a significant increase in the transfer of ARGs between Gram-negative Escherichia coli DH5α and Gram-positive Bacillus subtilis WB600, compared to the control without GO. Experimental validation through PCR and gel electrophoresis confirmed the successful transfer of the RP4 plasmid between the two bacterial strains. Additionally, GO treatment led to a significant decrease in cell viability for both donor and recipient bacteria, with their activities reaching approximately 42% and 45% of the control, respectively. Microscopic observations and physiological analyses demonstrated that GO induced oxidative stress, resulting in blurred or damaged cell membrane edges, increased membrane permeability, and enhanced adhesion and contact between bacterial cells. These factors likely contributed to the heightened frequency of RP4 plasmid transfer facilitated by GO. Transcriptomic analysis uncovered notable changes in gene expression in response to GO treatment. In both bacteria, genes such as ompA , ompW , and bamC associated with the cell membrane; oxyR , gor , and ahpC involved in oxidative stress; atpA , atpB , and atpE related to ATP generation regulation; as well as metastasis-related genes like korA , korB , and trbB were found to be altered. Overall, this study provides evidence that GO has the potential to promote the transfer of ARGs among diverse bacteria, highlighting the ecological risks and potential hazards associated with its presence.
- This article is part of the themed collection: Environmental Science: Nano Recent HOT Articles
Supplementary files
- Supplementary information PDF (269K)
Article information
Download citation, permissions.

Enhanced plasmid-mediated conjugative transfer of resistance genes across bacterial species promoted by graphene oxide
S. Zhang, J. Fang, H. Liu, Z. Li, L. Liu and S. Du, Environ. Sci.: Nano , 2024, 11 , 831 DOI: 10.1039/D3EN00951C
To request permission to reproduce material from this article, please go to the Copyright Clearance Center request page .
If you are an author contributing to an RSC publication, you do not need to request permission provided correct acknowledgement is given.
If you are the author of this article, you do not need to request permission to reproduce figures and diagrams provided correct acknowledgement is given. If you want to reproduce the whole article in a third-party publication (excluding your thesis/dissertation for which permission is not required) please go to the Copyright Clearance Center request page .
Read more about how to correctly acknowledge RSC content .
Social activity
Search articles by author, advertisements.
Thank you for visiting nature.com. You are using a browser version with limited support for CSS. To obtain the best experience, we recommend you use a more up to date browser (or turn off compatibility mode in Internet Explorer). In the meantime, to ensure continued support, we are displaying the site without styles and JavaScript.
- View all journals
- My Account Login
- Explore content
- About the journal
- Publish with us
- Sign up for alerts
- Open access
- Published: 13 February 2024
Photocatalytic degradation of organic dyes using reduced graphene oxide (rGO)
- Mizaj Shabil Sha 1 ,
- Hayarunnisa Anwar 1 ,
- Farzana N. Musthafa 1 ,
- Hamad Al-Lohedan 2 ,
- Sarya Alfarwati 1 ,
- Jothi Ramalingam Rajabathar 2 ,
- Johaina Khalid Alahmad 1 ,
- John-John Cabibihan 3 ,
- Muthusamy Karnan 4 &
- Kishor Kumar Sadasivuni 1 , 3
Scientific Reports volume 14 , Article number: 3608 ( 2024 ) Cite this article
918 Accesses
Metrics details
- Biochemistry
- Environmental sciences
Photocatalysts have developed into a successful strategy for degrading synthetic and organic toxins, such as chemicals and dyes, in wastewater. In this study, graphene oxide was reduced at different temperatures and used for degrading indigo carmine and neutral red dyes. The wide surface areas, strong adsorption sites, and oxygen functionalities of reduced graphene oxide (rGO) at 250 °C (rGO-250) produced more photocatalytic degradation efficiency and adsorption percentage. The catalyst dosage, initial dye concentration, solution pH and recyclability were all used to optimize the photocatalytic activity of rGO-250. This research presents a capable nano-adsorbent photocatalyst for the efficient degradation of organic dyes. GO and rGOs were also investigated for carbon dioxide (CO 2 ) absorption properties. Results showed that rGO-250 has better CO 2 adsorption properties than other rGOs. Overall, it was observed that rGO-250 has better photocatalytic and CO 2 adsorption capabilities compared to graphene oxide reduced at different temperatures.
Similar content being viewed by others
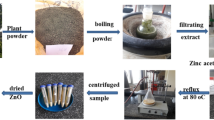
Enhanced photocatalytic degradation of methylene blue dye using eco-friendly synthesized rGO@ZnO nanocomposites
Asfaw Negash, Said Mohammed, … Minbale Gashu
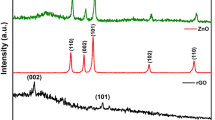
Effectual visible light photocatalytic reduction of para-nitro phenol using reduced graphene oxide and ZnO composite
Sasireka Velusamy, Anurag Roy, … Tapas K. Mallick
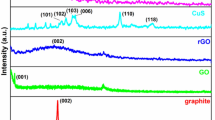
Experimental and DFT study of photocatalytic activity of reduced graphene oxide/copper sulfide composite for removal of organic dyes from water
Mohamed S. Sadek, Ghada E. Khedr, … Mohamed Abdel Hay Ismail
Introduction
Different facets of the textile manufacturing sectors depend heavily on dyes. However, because the dyes cannot degrade, they are frequently dumped carelessly into the aqueous environment 1 , 2 . Most dyes contain poisonous and carcinogenic characteristics that could harm ecosystems and people’s health. Due to their complex aromatic structures, dyes resist natural processes that cause degradation 3 , 4 . Because of this, various treatment methods have been used to remove dyes. Due to the availability of low-cost adsorbents with significant adsorption capacities, the adsorption method is the most popular strategy utilised in the industry 5 . Due to its simplicity, photocatalysis is yet another widely utilised technology. Reactive oxygen species (ROS) are generally acknowledged to include both superoxide (·O2 − ) and hydroxyl (·OH − ) radicals in the photooxidation of dye pollution. The amount of surface area of the materials utilised significantly impacts the removal of dyes in both reactions 6 , 7 .
Reduced graphene oxide (rGO), a chemically changed graphene, is more economically suited for mass production than pristine graphene. rGO frequently finds use in the production of composites based on graphene 8 . Various techniques, including microwave, thermal, photo-thermal, chemical, photo-chemical, and microbial/bacterial, can create rGO 9 . The chemical technique seems advantageous due to its low cost, ease of use, and large output. In particular, there are three key processes in this method's preparation of rGO 10 . The first stage entails the oxidation of graphite to produce graphite oxide, which introduces surface oxygen functions to the graphene layers 11 . Because graphite oxide has oxygenated surface functions such as carboxyls, hydroxyls, and epoxides, it may disperse in polar solvents and form stable dispersions 12 . Then, graphite oxide is exfoliated using mechanical stirring or sonication to produce graphene oxide (GO) with one or a few layers. Finally, by removing the surcial oxygen functions, GO is converted to rGO 11 . Adsorption reactions to remove organic and inorganic pollutants have historically used carbon-based compounds. The most often used adsorbent is activated carbon. Recently, rGO utilisation in dye adsorption applications has increased 13 , 14 , 15 . Because of some flaws in the graphitic domains and the remaining surficial oxygen functions, rGO is considered effective for the adsorptive removal of dyes. In general, rGO interacts with dyes by structural conjugation, hydrophobic association, electrostatic interaction, and π–π interaction 16 , 17 . Several different dyes can adsorb on rGO thanks to these interactions. It is ideal to have rGO with a wide surface area and high porosity to increase the dye adsorption capacity, and this is possible by managing the quality of the GO precursor and the reduction process 18 , 19 .
The technological advantages of photocatalysis are currently manifested through the photocatalytic degradation of dyes. Typically, electrons are stimulated, forming electrons and holes, when the energy of the light absorbed is equal to or less than the band gap of the photocatalyst. The photogenerated electron–hole pairs then use the nearby oxygen and water molecules to make ROS, which destroy the dye molecules 20 , 21 . Numerous studies have described using rGO in the photocatalytic removal of dye contaminants. The novelty of using reduced graphene oxide (rGO) for dye degradation lies in its remarkable catalytic properties and potential to revolutionize wastewater treatment methods. Unlike traditional approaches, rGO-based catalysts offer several unique advantages:
Enhanced catalytic activity: rGO exhibits high surface area and excellent electron transfer properties, enabling it to catalyze the degradation of dyes and other organic pollutants efficiently.
Improved reaction kinetics: Using rGO can significantly accelerate the degradation kinetics of dyes, reducing the time required for wastewater treatment.
Sustainability: rGO can be synthesized from abundant and inexpensive graphite sources, making it a sustainable and cost-effective option for environmental remediation.
Versatility: rGO can be functionalized and tailored to target specific dye molecules, increasing its versatility for various wastewater treatment applications.
Low environmental impact: Compared to some traditional chemical catalysts, rGO-based systems are often less harmful to the environment, aligning with the growing emphasis on green and sustainable technologies.
In summary, using rGO as a catalyst for dye degradation represents a novel and promising approach that addresses both the efficiency and sustainability challenges associated with wastewater treatment. This innovation has the potential to contribute significantly to cleaner water resources and reduced environmental pollution.
In this study, rGO was produced using a straightforward, eco-friendly solvothermal method without any harmful reducing chemicals, and it demonstrated outstanding adsorption capabilities and photoactivity towards the removal of IC and NR dye. The investigation examined the optimisation of extraneous elements such as catalyst concentration, initial dye concentration, light intensity, and pH.
All the chemicals used (graphite powder, potassium permanganate (KMnO 4 ), sulphuric acid (H 2 SO 4 , 98%), hydrogen peroxide (H 2 O 2 , 30%) and ethanol (C 2 H 5 OH) were of analytical grade, procured from Sigma Aldrich. Deionized water was used as the solvent for preparing practical solutions.
Methodology
Synthesis of go.
Graphite powder pretreatment is initially carried out to prepare Graphene oxide (GO). In the beginning, graphite (1 g) was added to H 2 SO 4 (25 ml). It was stirred in an ice bath for uniform suspension for a few minutes. After 20 min, the ice bath was removed.
A modified Hummer method is used to prepare GO from graphene. KMnO 4 (3 g) was added slowly to the solution and stirred for 3 h. 50 ml of distilled water was added slowly into the solution after 3 h. 50 ml of H 2 O 2 was added to cease the process. When a brown colour is observed, add 100 ml of distilled water. Add 5 ml of H 2 O 2 and centrifuge the mixture. Wash with H 2 O to collect the GO. For drying, it was placed in an oven at 60 °C for 2 h.
Preparation of reduced Graphene oxide (rGO) at different temperatures
To reduce GO to rGO, the solvothermal method was used. The mixture is then heated at different temperatures for the reduction process. Initially, 20 ml of C 2 H 5 OH and 200 mg of GO were mixed with 10 ml of deionized water. After vigorously sonicating the mixture for 30 min, a stable GO dispersion was produced. After that, the mixture was heated for 2 h at 100, 150, 200, and 250 °C in an 80 cc stainless steel autoclave lined with Teflon. The samples were designated rGO-x, with x standing for the investigated reduction temperatures. The rGO samples were subsequently filtered, washed, and dried.
Characterization of reduced graphene oxide
The X-ray diffractometer, a Malvern Panalytical Xpert (45 kV, 40 mA), was utilised to collect the XRD pattern from a Cu target. The materials were dispersed in ethanol before being put onto the copper grid for transmission electron microscopy (TEM) with an FEI Tecnai G2 S-Twin FEG 200 kV TEM. The sample was prepared for transmission electron microscopy (TEM) by dissolving the powder in isopropanol using a bath sonicator for 10–20 min. After being drop cast onto 200-grit carbon film, the sample was examined. An FTIR spectrometer called the Thermo Nicolet Nexus 670 was used to assess the purity of nanopowder. A Biochrom UV–Vis Spectrophotometer with a 190–1100 nm scanning range was used for the characterisation. A 300–750 nm scanning range and a medium scan speed were used in this case. The surface areas, pore diameters, and pore volumes of GO and rGOs were examined using Brunauer–Emmett–Teller (BET) analysis using the Micromeritics ASAP 2020 Surface Area and Porosity Analyzer (Georgia, USA). Before measurement, the samples were degassed at 150 °C for 24 h to eliminate moisture.
Dye degradation analysis
To 20 ml of distilled water, 15 mg of the photocatalyst (GO&rGO' s) were measured and added. The fluid was sonicated for around 30 min to produce a consistent suspension. Approximately 5 ml of this solution is added to the 40 ml of NR and IC aqueous solutions. The sample solution was left in the dark for roughly 30 min, allowing the reaction to reach adsorption equilibrium. After being maintained in the dark for 30 min, three millilitres of the sample are removed and subjected to UV–vis spectroscopy examination. Magnetic stirring was used to keep the solution stable while it was in the sun. A sample of the supernatant solution was taken every 20 min until three hours into the procedure. At this point, it was examined with UV–vis technology. The absorbance and wavelength were plotted. It was also investigated how varied catalyst loadings (1, 2, 3, 4, and 5 mg) of GO and rGOs, pH effect (3, 7, 10), recyclability, and initial dye concentrations (25, 40, 50, and 75 ppm) of two dyes would affect the process 22 .
The following equations were used to calculate the photodegradation efficiency (% Cdeg) and adsorption percentage (% Cads):
The dye concentrations at the start, after attaining adsorption–desorption equilibrium, and at time t are designated as C initial , C 0 , and C t , respectively. The pseudo-first-order kinetic equation shown below was then used to simulate the photocatalytic degradation reaction:
where k (time −1 ) is the rate constant for pseudo-first-order.
Evaluation of CO 2 adsorption properties
To evaluate the CO 2 adsorption properties, we dissolved 50 mg of GO and rGOs in 10 ml water. After passing CO 2 for 15 s in all the samples, it is titrated against 0.1 N NaOH, adding 10 drops of indicator. Noted the colour change and volume of NaOH for calculating the amount of CO 2 absorbed using the following equations.
where V 1 —Volume of the sample used; N 1 —Normality of the sample; V 2 —Volume of NaOH; N 2 —Normality of NaOH
The amount of CO 2 adsorbed was calculated using the following equation,
Results and discussion
Characterization.
In Fig. 1 a, at 2 = 26.570° and 2 = 54.700°, respectively, graphite showed an intense (002) peak and a very modest (101) peak. Both peaks were associated with JCPDS card 41-148713 and supported graphite structure. After oxidation, the (002) peak moved to 2 = 11.030°, caused by incorporating oxygenated functionalities and water molecules into the graphene layers. Despite this, the (002) peak of GO was still discernible during reduction at 100 and 150 °C, maybe due to the low reduction temperatures that only slightly converted GO to rGO. The (002) peak of GO started to fade as the reduction temperature rose, but a new, broader (002) peak of rGO started to appear at 2 = 24.890° for rGO-200 and 2 = 23.850° for rGO-250. The shifting of the (002) peak indicated that the sp 2 carbon structure had recovered partially 23 . Also, the (100) GO peak at 2θ = 42.630° was detected in all rGOs, resembling the turbostratic band of disordered carbon materials.
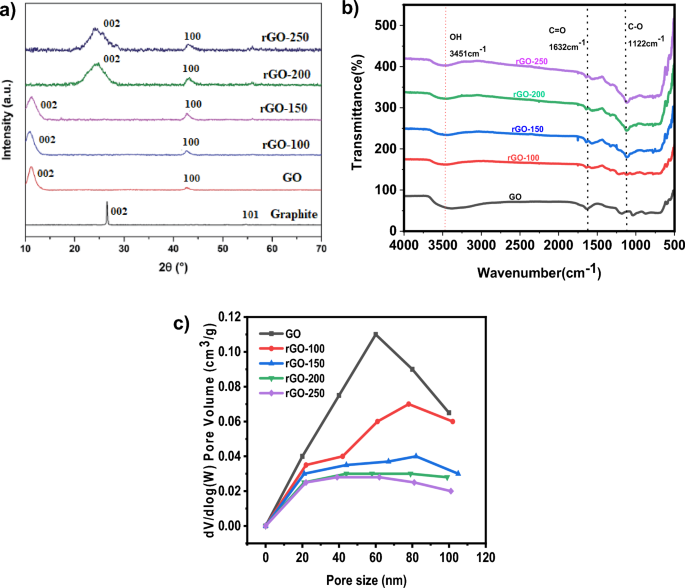
( a ) XRD patterns and ( b ) FTIR spectra of graphite, GO, rGO-100, rGO-150, rGO-200 and rGO-250 ( c ) Pore size distributions of GO,rGO-100, rGO-150, rGO-200 and rGO-250.
Figure 1 b displays the FTIR spectra of rGO samples produced at various reaction temperatures. The GO surface contains a large number of functional groups. Figure 1 b shows the peaks of C–O (alkoxy) stretch, O–H (hydroxyl) vibration, C=C aromatic stretch vibration, and C=O stretch on rGO surfaces at 1122, 1632, and 3451 cm −1 . As the temperature rose, so did the peak intensity corresponding to the C=O vibration band at 1632 cm −1 . From 100 to 250 °C, there was a decrease in the hydroxyl group's (3451 cm −1 ) permeability intensity 15 . As the temperature rises, the absorbed water molecules intercalate and vaporise. Furthermore, the hydrophilic characteristics of GO are lost, as well as the presence of functional groups containing oxygen 24 .
Figure 1 c shows the pore size distribution curves for GO and rGOs. According to Fig. 1 c, the total pore volume decreased from 0.11 to 0.037 cm 3 g −1 when the GO was lowered from 100 to 150 °C, decreasing the surface area (Table 1 ). This resulted most likely from the GO's aggregation action following the decrease. The considerable loss of oxygen functional groups led to the production of greater pore volume, which in turn resulted in a pronounced increase in surface area for rGO-250 at higher reduction temperatures 25 .
The UV–vis absorption spectra of GO and rGOs are shown in Fig. 2 a. The aromatic C=C bond π–π* transition of GO results in an absorption peak at 284 nm. The temperature ranged from 100 to 250 °C, and as a result, the peak location gradually shifted to lower wavelengths 13 . Peak shifting partially restored the sp 2 conjugation, leading to a narrower band gap 17 . The optical band gaps of GO and rGOs were subsequently determined using the linear fits of the Tauc plots, as seen in Fig. 2 b. Table 2 shows the band gap of the samples. The band gap of GO was 2.80 eV, which was in line with results from earlier literature 26 .
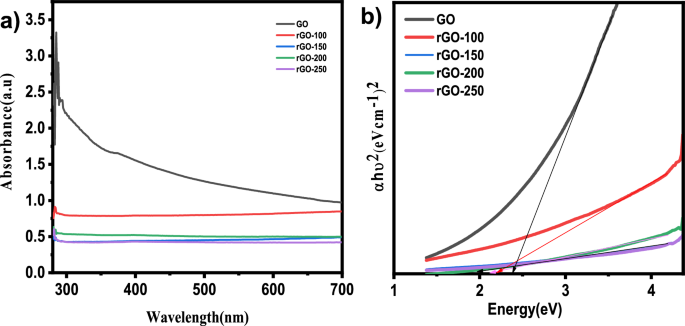
( a ) UV–vis absorption spectra and ( b ) Tauc plots of graphite, GO, rGO-100, rGO-150, rGO-200 and rGO-250.
The TEM micrographs in Fig. 3 a and b show the sheet-like morphology of GO and wrinkled rGO caused by functional group evaporation and thermal instability of GO during annealing 19 . The GO displayed a pristine surface and a closely packed lamellar and plate structure consistent with previously made GO. The distinctive sheet-like silky waves, wrinkled appearance, and clumped structure of rGO, in contrast to GO, were between 20 and 100 nm in size 27 .
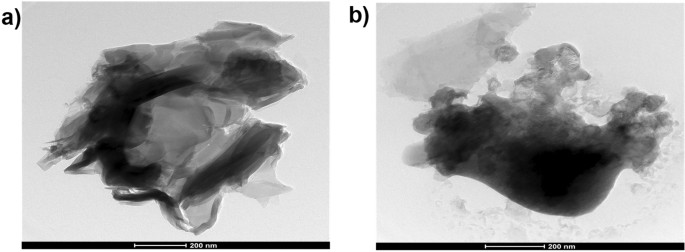
TEM image of ( a ) GO and ( b ) rGO-250.
Photocatalytic activity of rGOs
Structural conjugation, hydrophobic association, electrostatic interaction, and -interaction is how rGO interacts with dyes. These interactions allow a variety of dyes to adsorb on rGO. To maximise the dye adsorption capacity, it is essential to have rGO with a large surface area and high porosity. This is achievable by carefully controlling the GO precursor's quality and the reduction process. The mechanism of dye degradation involves the excitation of dye from its ground state (Dye) to its triplet excited state (Dye*) under visible light photon (λ > 400 nm). An electron injection into the conduction band transforms this excited state dye species into a semi-oxidized radical cation (Dye +· ). Superoxide radical anions (O 2 -· ) are produced as a result of an interaction between these trapped electrons and the dissolved oxygen in the system, which in turn causes the creation of hydroxyl radicals (OH · ) (Fig. 4 ).
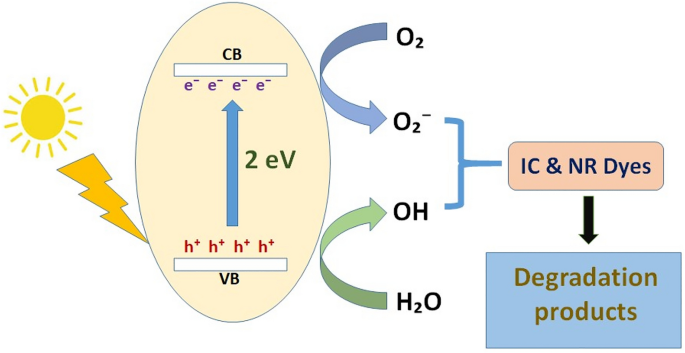
Mechanism of dye degradation.
Figure 5 illustrates the photocatalytic activity of different rGOs in IC and NR, respectively. The solution is collected after the dyes have been exposed to the sun for a predetermined time. It is measured how much UV–vis is absorbed (Figure S1 , S2 , S3 and S4 ). Table 3 shows the concentration–time profile (C t /C 0 ) and degradation efficiency (%), which were derived using the absorbance values obtained for the initial dye concentration (C 0 ) and the absorbance values acquired at certain time intervals (C t ). The photodegradation of dyes was found to be much more effective with rGO-250.It may be attributed to the fact that the rGO-250 may have the large and optimum surface area for the highest adsorption of dyes 28 , 29 . Figure 6 a and c depict GO and rGO photoactivity, respectively, while Fig. 6 b and d depict the pseudo-first-order fitting of photocatalytic dye degradation. Table 3 shows the values for % C ads , % C deg , k, and R 2 . rGO-100, rGO-150, and rGO-200 behaved similarly to GO in the dye adsorption process to other rGOs because low reduction temperatures were insufficient for GO reduction. Because of its large surface area, rGO-250 had the highest dye adsorption 30 , with an adsorption percentage of 29.26 for IC and 25.23 for NR, respectively. The photocatalytic degradation efficiency of rGO-250 was 36.28 and 25.73 for IC and NR, respectively.
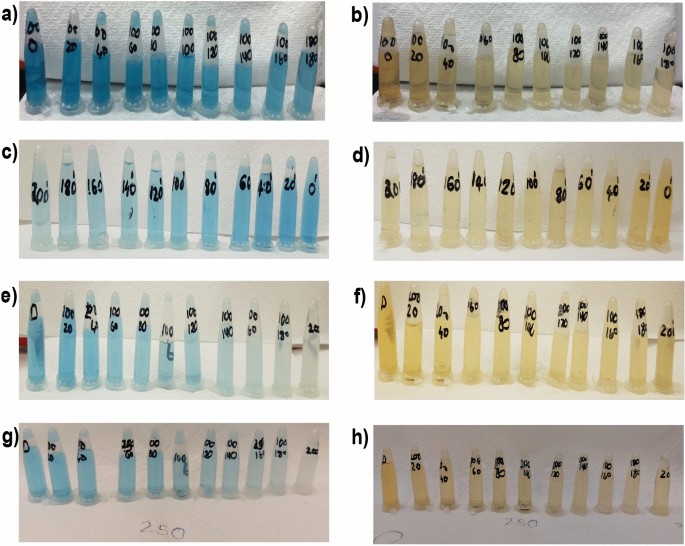
Dye degradation analysis of ( a , b ) rGO-100 in IC and NR respectively ( c , d ) rGO-150 in IC and NR respectively ( e , f ) rGO-200 in IC and NR respectively ( a , b ) rGO-250 in IC and NR respectively.
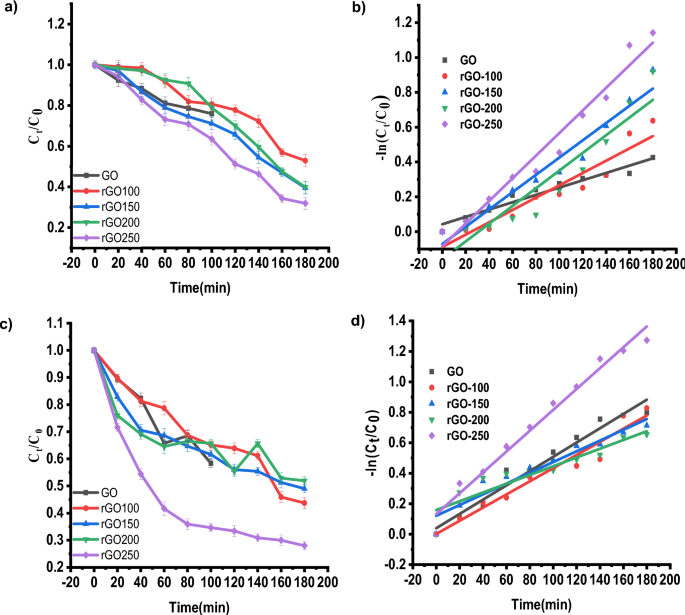
( a , c ) Degradation of IC and NR dyes normalized against adsorption, ( b , d ) Pseudo-first-order kinetic plot of IC and NR dyes, respectively, in the presence of GO, rGO-100, rGO-150, rGO-200 and rGO-250.
The statistical analysis of linear curve fit was performed using origin software. This statistical analysis aimed to regulate factors affecting dye photodegradation performance. The statistical analysis results of fitted curves for indigo carmine and neutral dye are represented in Table 4 . Statistical evidence, such as Fisher variation ratio (F-value) and probability value ( P value), was used to assess the linear fit for estimating photodegradation performance. Additionally, degrees of freedom (DF), mean squares (MS), and sum of squares (SS) were calculated. The F-value of 14.55 indicates the model is significant. Noise has a 0.01% probability of causing a ''Model F-value". Significant model terms are indicated by “'Probability > F” values < 0.0500.
Effect of pH
15 mg of rGO-250 in 20 ml distilled water kept for sonication of about 30 min. Two sets of dye solutions (IC&NR) were made of pH 3, 7 and 10. 5 ml from the catalyst solution was added to 40 ml of aqueous solutions of dyes of 25 ppm of concentrations (Fig. 7 ). The degradation (91.85% for IC and 90.17% for NR) is much more pronounced in the basic medium (pH 10) for both dyes than in the acidic (pH 3, 85.15% for IC and 84.58%for NR) and neutral medium (87.92% for IC and 86.47% for NR) (Table 5 ). This may be because the solution contained more hydrogen ions under acidic conditions. Many hydrogen ions competed with the dye molecules for unoccupied adsorption sites on the catalyst surface. In this case, IC is an anionic dye, and NR is a neutral dye 28 .
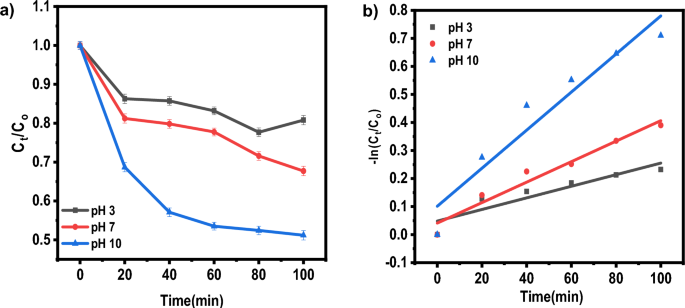
( a ) Effect of pH on photocatalytic degradation of NR normalized under adsorption. ( b ) The plot of the log of concentration with time.
Effect of initial dye concentrations
The photocatalytic degradation of dyes was studied using various initial concentrations such as 25, 40, 50 and 75 ppm.15 mg of catalyst in 20 ml of DI water was added to 40 ml of each dye (IC, NR) of varied initial concentration (Table 6 ). An initial concentration of 25 ppm was observed to have greater dye degradation efficiency than other initial dye concentrations for both dyes (91.85% for IC and 90.17% for NR). As the initial concentration increases, degradation efficiency decreases. This may be because the number of dye molecules contacting the catalyst surface and the number of dye molecules in the solution rises. This leads to the active sites on the catalyst surface being covered by dye molecules, which interferes with photon arrival and reduces catalytic efficiency. As a result, less OH radical generation occurs on the catalyst surface 23 , 31 . As a result, increased dye concentration can reduce removal efficiency and reaction rate (Fig. 8 ).
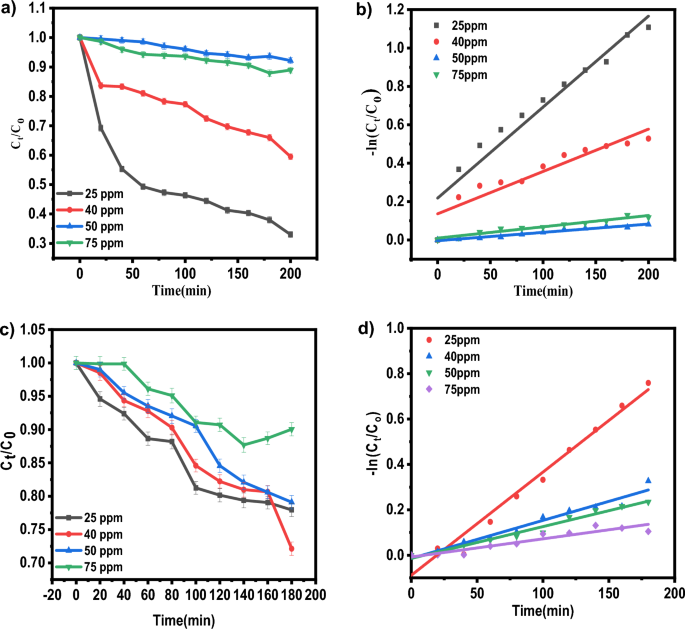
( a , c ) Effect of initial dye concentration of IC, NR on photocatalytic degradation of dyes normalized against adsorption. ( c , d ) The plot of the log of concentration with time.
effect of catalyst loading
An essential practical factor affecting the photocatalytic reaction's effectiveness is catalyst loading. The number of active sites in the reaction system rises due to increased catalyst loading since it increases the concentration of the active material overall. When it comes to the photodegradation removal process, increasing the dose often results in higher removal efficiencies up until a point at which adding more catalyst results in lower light transmittance of the suspension and lower removal efficiencies.
rGO-250 is dissolved in 50 ml of distilled water in a variety of ratios, including 15, 25, 35, 45, and 55 mg. 10 ml of dye solutions with an IC and NR of 25 ppm each and a pH of 10 were combined with 5 ml of this solution. For 30 min, the sample solutions were left in the dark. 3 ml are withheld after 30 min, and UV–vis recordings are made. For roughly 3 h, the remaining sample solution was exposed to sunshine. After every hour, the aliquots were removed, and UV–vis measurements were taken.
As the catalyst loading increased, it was found that the deterioration grew (Table 7 ). For 15 mg of catalyst, degradation efficiency was ~ 92 for both dyes. The degradation efficiency was determined to be 98.74% for IC and 97.56% for NR, respectively, with a catalyst dosage of 55 mg. Herein, The overall surface area of the photocatalyst grew along with the catalyst concentration. Thus, there were more accessible adsorption sites, which improved the dye adsorption procedure (Fig. 9 ). Additionally, when the total amount of catalyst particles rose, photon absorption for generating electrons and holes and the rate of the synthesis of reactive oxygen species (ROS) for degrading dye molecules both improved 32 , 33 .
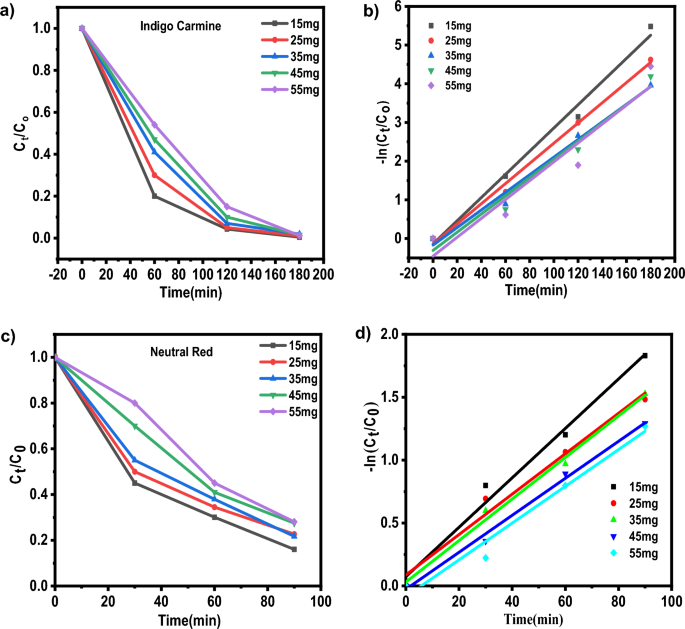
( a , c ) Effect of catalyst loading on photocatalytic degradation of dyes normalized against adsorption. ( c , d ) The plot of the log of concentration with time.
Table 8 compares the present work with the existing literature values. The proposed work has the highest degradation efficiency with lower catalyst dosage compared to existing literature for the degradation of IC. In the case of NR dye, it was observed that the rGO catalyst only exhibits a degradation efficiency higher than 90%, whereas the other literature exhibits a degradation efficiency less than 85%. In conclusion, using rGO as a catalyst for both IC and NR degradation demonstrates significant promise in enhancing the efficiency and kinetics of dye removal compared to the existing literature. This innovative approach not only offers an eco-friendly and cost-effective solution for wastewater treatment but also opens up avenues for further research and development in the field of advanced materials and catalysis.
Recyclability
Recyclability studies in photocatalysis are essential for assessing the practicality and sustainability of photocatalytic processes for various applications, such as water purification, air pollution control, and organic pollutant degradation. Recyclability studies aim to determine the ability to reuse the photocatalyst multiple times without significantly losing its catalytic activity. According to the set of photocatalytic tests, rGO-250 is a useful material for the breakdown of both dye molecules. As a result, the ability of this decreased graphene oxide material to degrade dye molecules across consecutive cycles of degradation is tested. The catalyst is removed from each photocatalytic experiment and used for the subsequent cycle of the degradation investigation for this reason. The rGO-250 recovered from each cycle is thoroughly cleaned with deionized water before being added to the catalytic process.
Interestingly, its stability is maintained during the five degradation cycles with barely perceptible alterations. Figure 10 shows the stability of rGO-250 in the photocatalytic dye degradation reaction as a function of cycle number. The percentage degradation was still greater than 90% after five consecutive runs. It attests to the catalyst's structural stability even after five cycles of degradation tests. Under exposure to direct sunshine, it can be employed as a possible catalyst for the degradation of IC and NR dyes 25 , 28 .
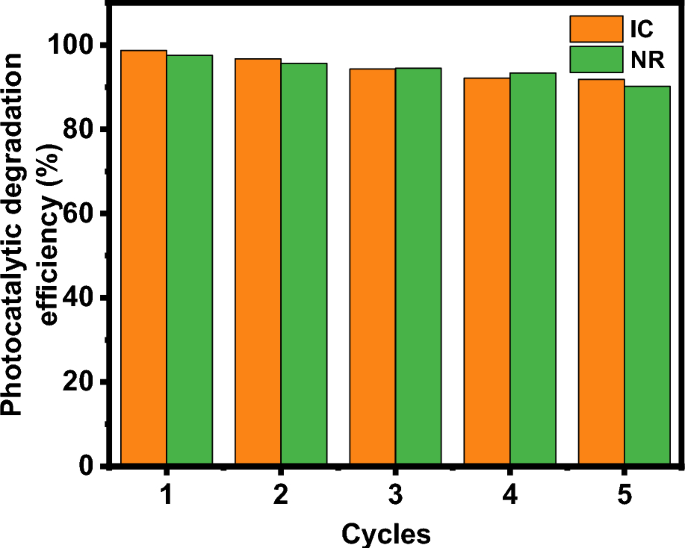
Recyclability analysis of rGO-250 for photodegradation of IC and NR dyes, respectively.
CO 2 adsorption performance of rGOs
Table 9 summarizes the comparison of CO 2 uptakes by GOs and rGOs. The rGO-250 was the best compared to other adsorbents (Fig. 11 ). This excellent adsorption performance is attributed to increased surface area at higher reduction temperatures, owing to the significant removal of oxygen functional groups and increased pore volume. After removing surcial oxygen functionalities, graphene's sp 2 - hybridized structure was partially restored at 250 °C 41 , 42 .
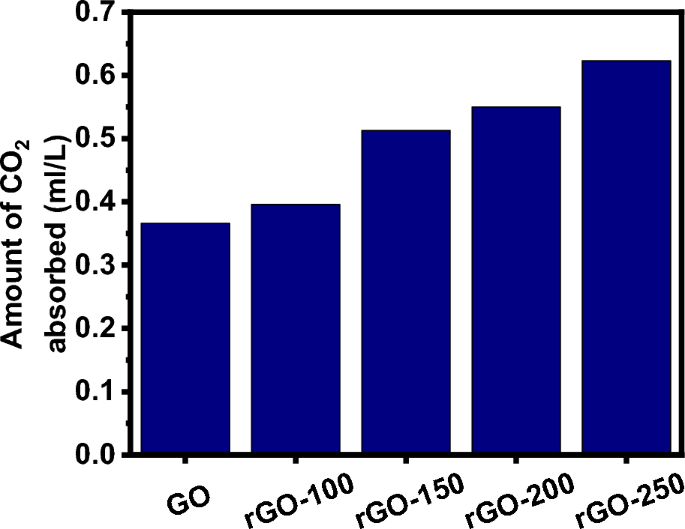
CO 2 adsorption by GO and rGOs.
A simple one-pot solvothermal approach was used to fabricate reduced graphene oxide at different annealing temperatures. These rGOs were tested for dye adsorption and CO 2 adsorption properties. rGO-250 then achieved the best adsorptive removal (29.26% for IC and 25.23% for NR) and photocatalytic degradation (36.28% for IC and 25.73% for NR) of dyes, revealing that the main reasons were increased surface area and narrowing of the band gap. The optimal adsorption (91.74% for IC and 94.81% for NR) and photocatalytic activity (98.74% for IC and 98.56% for NR) of rGO-250 were improved by increasing the amount of catalyst to 55 mg, the initial dye concentration to 25 ppm, and the solution pH to 10. rGO-250 was able to degrade more than 90% of dyes after repeating the reaction up to five times, indicating that it was highly stable and reusable. It was also observed that rGO-250 possesses better CO 2 adsorption properties than other rGOs.
Data availability
The data supporting this study's findings are available at the request of the corresponding author.
Aldalbahi, A. et al. Effects of technical textiles and synthetic nanofibers on environmental pollution. Polymers (Basel) 13 , 155 (2021).
Article CAS PubMed PubMed Central Google Scholar
Kabir, S. M. F. et al. Removal of acid dyes from textile wastewaters using fish scales by absorption process. Clean Technol. 1 , 311–324 (2019).
Article Google Scholar
Lellis, B., Fávaro-Polonio, C. Z., Pamphile, J. A. & Polonio, J. C. Effects of textile dyes on health and the environment and bioremediation potential of living organisms. Biotechnol. Res. Innov. 3 , 275–290 (2019).
Al-Tohamy, R. et al. A critical review on the treatment of dye-containing wastewater: Ecotoxicological and health concerns of textile dyes and possible remediation approaches for environmental safety. Ecotoxicol. and Environ. Saf. 231 , 113160 (2022).
Article CAS Google Scholar
Dutta, S., Gupta, B., Kumar Srivastava, S. & Kumar Gupta, A. Recent advances on removing dyes from wastewater using various adsorbents: A critical review. Mater. Adv. 2 , 4497–4531 (2021).
Pavel, M. et al. Photocatalytic degradation of organic and inorganic pollutants to harmless end products: Assessment of practical application potential for water and air cleaning. Catalysts 13 , 380 (2023).
Bi, Y. & Westerhoff, P. High-throughput analysis of photocatalytic reactivity of differing TiO 2 formulations using 96-well microplate reactors. Chemosphere 223 , 275–284 (2019).
Article CAS PubMed ADS Google Scholar
Ahmed, A. et al. Synthesis techniques and advances in sensing applications of reduced graphene oxide (rGO) Composites: A review. Compos. Part A Appl. Sci. Manuf. 165 , 107373 (2023).
Melo, J. F. et al. Industrial waste reuse: An alternative source to reduced graphene oxide for preparing electrochemical sensors. Electrochim. Acta 454 , 142382 (2023).
Mombeshora, E. T. & Muchuweni, E. Dynamics of reduced graphene oxide: Synthesis and structural models. RSC Adv. 13 , 17633–17655 (2023).
Article CAS PubMed PubMed Central ADS Google Scholar
Majumder, P. & Gangopadhyay, R. Evolution of graphene oxide (GO)-based nanohybrid materials with diverse compositions: An overview. RSC Adv. 12 , 5686–5719 (2022).
Faniyi, I. O. et al. The comparative analyses of reduced graphene oxide (RGO) prepared via green, mild and chemical approaches. SN Appl. Sci. 1 , 1181 (2019).
Wang, B., Lan, J., Bo, C., Gong, B. & Ou, J. Adsorption of heavy metal onto biomass-derived activated carbon: Review. RSC Adv. 13 , 4275–4302 (2023).
Article PubMed PubMed Central ADS Google Scholar
Bazan-Wozniak, A., Cielecka-Piontek, J., Nosal-Wiercińska, A. & Pietrzak, R. Adsorption of organic compounds on adsorbents obtained with the use of microwave heating. Materials (Basel) 15 , 5664 (2022).
Wang, J., Wang, R., Ma, J. & Sun, Y. Study on the application of shell-activated carbon for the adsorption of dyes and antibiotics. Water 14 , 3752 (2022).
Kumari, M. & Pulimi, M. Sulfate radical-based degradation of organic pollutants: A review on application of metal–organic frameworks as catalysts. ACS Omega 8 , 34262–34280 (2023).
Ramesha, G. K., Ashok, V., Muralidhara, H. B. & Sampath, S. Graphene and graphene oxide as effective adsorbents toward anionic and cationic dyes. J. Colloid Interface Sci. 361 , 270–277 (2011).
Nizam, N. U. M., Hanafiah, M. M., Mahmoudi, E., Mohammad, A. W. & Oyekanmi, A. A. Effective adsorptive removal of dyes and heavy metal using graphene oxide based Pre-treated with NaOH/H 2 SO 4 rubber seed shells synthetic graphite Precursor: Equilibrium Isotherm, kinetics and thermodynamic studies. Sep. Purif. Technol. 289 , 120730 (2022).
Ab Aziz, N. A. H. et al. Non-functionalized oil palm waste-derived reduced graphene oxide for methylene blue removal: Isotherm, kinetics, thermodynamics, and mass transfer mechanism. Arab. J. Chem. 16 , 104387 (2023).
Porcu, S., Secci, F. & Ricci, P. C. Advances in hybrid composites for photocatalytic applications: A review. Molecules 27 , 6828 (2022).
Tahir, M. B., Sohaib, M., Sagir, M. & Rafique, M. Role of nanotechnology in photocatalysis. Encycl. Smart Mater. https://doi.org/10.1016/B978-0-12-815732-9.00006-1 (2022).
Sha, M. S. et al. Antibacterial and catalytic performance of rGO-CNT-ZrO 2 composite. Int. J. Environ. Anal. Chem. https://doi.org/10.1080/03067319.2023.2230146 (2023).
Kumar, M. et al. Sonophotocatalytic dye degradation using rGO-BiVO 4 composites. Glob. Chall. 6 , 2100132 (2022).
Article PubMed PubMed Central Google Scholar
Çeti̇nkaya Gürer, S. & Kütük, N. Effect of reduction temperature and time on the reduction of graphene oxide with white cabbage extract. Bitlis Eren Üniversitesi Fen Bilimleri Dergisi 10 , 1314–1323 (2021).
Er Siong, V. L. et al. Removal of methylene blue dye by solvothermally reduced graphene oxide: A metal-free adsorption and photodegradation method. RSC Adv. 9 , 37686–37695 (2019).
Article ADS Google Scholar
Habte, A. T. & Ayele, D. W. Synthesis and characterization of reduced graphene oxide (rGO) started from graphene oxide (GO) using the tour method with different parameters. Adv. Mater. Sci. Eng. 2019 , e5058163 (2019).
Yu-guo, Y. & Gurunathan, S. Combination of graphene oxide–silver nanoparticle nanocomposites and cisplatin enhances apoptosis and autophagy in human cervical cancer cells. Int. J. Nanomed. 12 , 6537–6558 (2017).
Parthipan, P., Cheng, L., Rajasekar, A., Govarthanan, M. & Subramania, A. Biologically reduced graphene oxide as a green and easily available photocatalyst for degradation of organic dyes. Environ. Res. 196 , 110983 (2021).
Article CAS PubMed Google Scholar
Zhang, S. et al. Carbon dots as metal-free photocatalyst for dye degradation with high efficiency within nine minutes in dark. Opt. Mater. 123 , 111914 (2022).
Wang, J. et al. Degradation of organic dyes by P25-reduced graphene oxide: Influence of inorganic salts and surfactants. J. Environ. Chem. Eng. 3 , 1437–1443 (2015).
Rajendran, R. et al. Dimensionally integrated nanoarchitectonics for a novel composite from 0D, 1D, and 2D nanomaterials: RGO/CNT/CeO2 ternary nanocomposites with electrochemical performance. J. Mater. Chem. A 2 , 18480–18487 (2014).
Sodeinde, K. O., Olusanya, S. O., Lawal, O. S., Sriariyanun, M. & Adediran, A. A. Enhanced adsorptional-photocatalytic degradation of chloramphenicol by reduced graphene oxide–zinc oxide nanocomposite. Sci. Rep. 12 , 17054 (2022).
Yu, L., Xu, W., Liu, H. & Bao, Y. Titanium dioxide–reduced graphene oxide composites for photocatalytic degradation of dyes in water. Catalysts 12 , 1340 (2022).
Ziyaadini, M. & Ghashang, M. Sunlight-induced photocatalytic degradation of indigo carmine using Bi 5 Ti 3 FeO 15 layered structure. Optik 228 , 166207 (2021).
Article CAS ADS Google Scholar
Lubis, S. & Sitompul, D. W. Photocatalytic degradation of indigo carmine dye using α-Fe2O3/bentonite nanocomposite prepared by mechanochemical synthesis. IOP Conf. Ser. Mater. Sci. Eng. 509 , 012142 (2019).
Devarahosahalli Veeranna, K., Theeta Lakshamaiah, M. & Thimmasandra Narayan, R. Photocatalytic degradation of indigo carmine dye using calcium oxide. Int. J. Photochem. 2014 , e530570 (2014).
Taie, A. A. & Dah, H. M. Photocatalytic degradation of indigo carmine by ZnO photocatalyst under visible light irradiation. Baghdad Sci. J. 14 , 0582–0582 (2017).
Mahfoozi, F., Mahmoudi, A., Sazegar, M. R. & Nazari, K. High-performance photocatalytic degradation of neutral red over cobalt grafted-mesoporous silica under UV irradiation. J. Sol Gel Sci. Technol. 100 , 170–182 (2021).
Singh, P., Hasija, A., Thakur, C., Chopra, D. & Siddiqui, K. A. Exploring the pH reliant high photocatalytic degradation of organic dyes using H-bonded Ni(II) coordination network. J. Mol. Struct. 1276 , 134784 (2023).
Sharma, O. & Sharma, M. K. Use of cobalt hexacyanoferrate(II) semiconductor in photocatalytic degradation of neutral red dye. Int. J. ChemTech Res. 5 , 1615–1622 (2013).
CAS Google Scholar
Mehra, P. & Paul, A. Decoding carbon-based materials’ properties for high CO 2 capture and selectivity. ACS Omega 7 , 34538–34546 (2022).
Politakos, N., Cordero-Lanzac, T. & Tomovska, R. Understanding the adsorption capacity for CO 2 in reduced graphene oxide (rGO) and modified ones with different heteroatoms in relation to surface and textural characteristics. Appl. Sci. 11 , 9631 (2021).
Download references
Acknowledgements
This work was supported by the Qatar National Research Fund under the Grant No. MME03-1226-210042 and the authors (Hamad Al-lohedan) extend his appreciation to financial support form Researchers Supporting Project number (RSP2024R54), King Saud University, Saudi Arabia. The statements made herein are solely the responsibility of the authors.Open Access funding provided by the Qatar National Library.
Open Access funding provided by the Qatar National Library.
Author information
Authors and affiliations.
Center for Advanced Materials, Qatar University, PO Box 2713, Doha, Qatar
Mizaj Shabil Sha, Hayarunnisa Anwar, Farzana N. Musthafa, Sarya Alfarwati, Johaina Khalid Alahmad & Kishor Kumar Sadasivuni
Chemistry Department, College of Science, King Saud University, P.O. Box. 2455, Riyadh, 11451, Kingdom of Saudi Arabia
Hamad Al-Lohedan & Jothi Ramalingam Rajabathar
Department of Mechanical and Industrial Engineering, College of Engineering, Qatar University, P.O. Box. 2713, Doha, Qatar
John-John Cabibihan & Kishor Kumar Sadasivuni
Grassland and Forage Division, National Institute of Animal Science, Rural Development Administration, Wanju, South Korea
Muthusamy Karnan
You can also search for this author in PubMed Google Scholar
Contributions
All authors contributed to the study's conception and design. J.K.A. and S.A. did material preparation and characterization. Analysis and data collection and evaluation were performed by M.S.S., H.A. and F.N.M. M.S.S. wrote the first draft of the manuscript, and all authors commented on previous versions. All authors read and approved the final manuscript.
Corresponding authors
Correspondence to Jothi Ramalingam Rajabathar or Kishor Kumar Sadasivuni .
Ethics declarations
Competing interests.
The authors declare no competing interests.
Additional information
Publisher's note.
Springer Nature remains neutral with regard to jurisdictional claims in published maps and institutional affiliations.
Supplementary Information
Supplementary information., rights and permissions.
Open Access This article is licensed under a Creative Commons Attribution 4.0 International License, which permits use, sharing, adaptation, distribution and reproduction in any medium or format, as long as you give appropriate credit to the original author(s) and the source, provide a link to the Creative Commons licence, and indicate if changes were made. The images or other third party material in this article are included in the article's Creative Commons licence, unless indicated otherwise in a credit line to the material. If material is not included in the article's Creative Commons licence and your intended use is not permitted by statutory regulation or exceeds the permitted use, you will need to obtain permission directly from the copyright holder. To view a copy of this licence, visit http://creativecommons.org/licenses/by/4.0/ .
Reprints and permissions
About this article
Cite this article.
Shabil Sha, M., Anwar, H., Musthafa, F.N. et al. Photocatalytic degradation of organic dyes using reduced graphene oxide (rGO). Sci Rep 14 , 3608 (2024). https://doi.org/10.1038/s41598-024-53626-8
Download citation
Received : 18 July 2023
Accepted : 02 February 2024
Published : 13 February 2024
DOI : https://doi.org/10.1038/s41598-024-53626-8
Share this article
Anyone you share the following link with will be able to read this content:
Sorry, a shareable link is not currently available for this article.
Provided by the Springer Nature SharedIt content-sharing initiative
By submitting a comment you agree to abide by our Terms and Community Guidelines . If you find something abusive or that does not comply with our terms or guidelines please flag it as inappropriate.
Quick links
- Explore articles by subject
- Guide to authors
- Editorial policies
Sign up for the Nature Briefing newsletter — what matters in science, free to your inbox daily.

Synthesis and characterization of zinc selenide/graphene oxide (ZnSe/GO) nanocomposites for electrochemical detection of cadmium ions
- Published: 08 April 2024
- Volume 130 , article number 297 , ( 2024 )
Cite this article
- Bhawani Sharma 1 ,
- Anoop Singh 2 , 3 ,
- Asha Sharma 4 ,
- Aman Dubey 5 ,
- Vinay Gupta 6 ,
- Rashad Gabil Oglu Abaszade 7 ,
- Ashok K. Sundramoorthy 8 ,
- Navdeep Sharma 9 &
- Sandeep Arya ORCID: orcid.org/0000-0001-5059-0609 5
Cadmium (Cd), a heavy metal that is very harmful to both the environment and human health, makes it difficult to detect in environmental and analytical research. However, given the adverse impacts, a remedy is urgently required. Due to its special benefits, electrochemical approaches have recently attracted a lot of interest for the detection of Cd(II) ions. We have prepared zinc selenide/graphene oxide (ZnSe/GO) nanocomposites and characterized its structural, compositional, and morphological properties using X-ray diffraction, elemental mapping, field emission scanning electron microscopy, Fourier transform infrared spectroscopy, X-ray photoelectron spectroscopy and energy dispersive X-ray spectroscopy, to fabricate an efficient electron mediator for electrochemical sensor. Results of the experiments proved that the device is reliable in Cd(II) detection with 45.54 µM limit of detection (LOD) and 138.00 µM limit of quantification (LOQ). The sensor showed a linear range of output current at 100–700 µM Cd(II) with the coefficient of correlation (R 2 = 0.996). Interferents have a negligible effect on the response of the sensor.
Graphical abstract
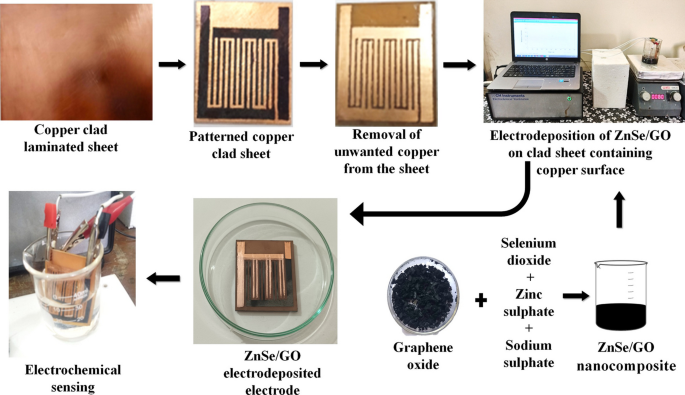
This is a preview of subscription content, log in via an institution to check access.
Access this article
Price includes VAT (Russian Federation)
Instant access to the full article PDF.
Rent this article via DeepDyve
Institutional subscriptions
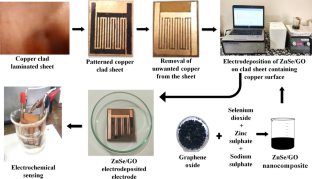
Data and code availability
The raw/processed data required to reproduce these findings will be available on the reasonable request.
B. Gao, H. Liu, Z. Gu, An exothermic chip for point-of-care testing using a forehead thermometer as a readout. Lab Chip 16 (3), 525–531 (2016)
Article Google Scholar
S. Thakur, L. Singh, Z.A. Wahid, M.F. Siddiqui, S.M. Atnaw, M.F.M. Din, Plant-driven removal of heavy metals from soil: uptake, translocation, tolerance mechanism, challenges, and future perspectives. Environ. Monit. Assess. 188 (4), 1–11 (2016)
B. Braune, J. Chételat, M. Amyot, T. Brown, M. Clayden, M. Evans, A. Fisk, A. Gaden, C. Girard, A. Hare, J. Kirk, Mercury in the marine environment of the Canadian Arctic: review of recent findings. Sci. Total. Environ. 509 , 67–90 (2015)
Article ADS Google Scholar
K. Nogawa, M. Sakurai, M. Ishizaki, T. Kido, H. Nakagawa, Y. Suwazono, Threshold limit values of the cadmium concentration in rice in the development of itai-itai disease using benchmark dose analysis. J. Appl. Toxicol. 37 (8), 962–966 (2017)
M. Nishijo, H. Nakagawa, Y. Suwazono, K. Nogawa, T. Kido, Causes of death in patients with Itai-itai disease suffering from severe chronic cadmium poisoning: a nested case–control analysis of a follow-up study in Japan. BMJ Open 7 (7), e015694 (2017)
M. Ghaedi, F. Ahmadi, A. Shokrollahi, Simultaneous preconcentration and determination of copper, nickel, cobalt and lead ions content by flame atomic absorption spectrometry. J. Hazard. Mater. 142 (1–2), 272–278 (2007)
J.S. Becker, M. Zoriy, A. Matusch, B. Wu, D. Salber, C. Palm, J.S. Becker, Bioimaging of metals by laser ablation inductively coupled plasma mass spectrometry (LA-ICP-MS). Mass Spectrom. Rev. 29 (1), 156–175 (2010)
C. Huang, Hu. Bin, Silica-coated magnetic nanoparticles modified with γ-mercaptopropyltrimethoxysilane for fast and selective solid phase extraction of trace amounts of Cd, Cu, Hg, and Pb in environmental and biological samples prior to their determination by inductively coupled plasma mass spectrometry. Spectrochim. Acta Part B 63 (3), 437–444 (2008)
C.B. Ojeda, F.S. Rojas, Recent developments in derivative ultraviolet/visible absorption spectrophotometry. Anal. Chim. Acta 518 (1–2), 1–24 (2004)
F. Panfili, A. Manceau, G. Sarret, L. Spadini, T. Kirpichtchikova, V. Bert, A. Laboudigue, M.A. Marcus, N. Ahamdach, M.-F. Libert, The effect of phytostabilization on Zn speciation in a dredged contaminated sediment using scanning electron microscopy, X-ray fluorescence, EXAFS spectroscopy, and principal components analysis. Geochim. Cosmochim. Acta 69 (9), 2265–2284 (2005)
T.A. Kirpichtchikova, A. Manceau, L. Spadini, F. Panfili, M.A. Marcus, T. Jacquet, Speciation and solubility of heavy metals in contaminated soil using X-ray microfluorescence, EXAFS spectroscopy, chemical extraction, and thermodynamic modeling. Geochim. Cosmochim. Acta 70 (9), 2163–2190 (2006)
A. Sharma, A. Ahmed, A. Singh, S.K. Oruganti, A. Khosla, S. Arya, Recent advances in tin oxide nanomaterials as electrochemical/chemiresistive sensors. J. Electrochem. Soc. 168 (2), 027505 (2021)
A. Singh, A. Sharma, A. Ahmed, A.K. Sundramoorthy, H. Furukawa, S. Arya, A. Khosla, Recent advances in electrochemical biosensors: applications, challenges, and future scope. Biosensors 11 (9), 336 (2021)
A. Singh, A. Ahmed, A. Sharma, S. Arya, Graphene and its derivatives: synthesis and application in the electrochemical detection of analytes in sweat. Biosensors 12 (10), 910 (2022)
S. Kumar, W. Ahlawat, R. Kumar, N. Dilbaghi, Graphene, carbon nanotubes, zinc oxide and gold as elite nanomaterials for fabrication of biosensors for healthcare. Biosens. Bioelectron. 70 , 498–503 (2015)
A. Ahmed, A. Singh, S.J. Young, V. Gupta, M. Singh, S. Arya, Synthesis techniques and advances in sensing applications of reduced graphene oxide (rGO) composites: a review. Compos. Part A: Appl. Sci. Manuf. 165 , 107373 (2023)
A.F. Memon, S. Ameen, N. Qambrani, J.A. Buledi, N.H. Khand, A.R. Solangi, S.I.H. Taqvi, C. Karaman, F. Karimi, E. Afsharmanesh, An improved electrochemical sensor based on triton X-100 functionalized SnO 2 nanoparticles for ultrasensitive determination of cadmium. Chemosphere 300 , 134634 (2022)
Q. Shan, J. Tian, Q. Ding, W. Wu, Electrochemical sensor based on metal-free materials composed of graphene and graphene oxide for sensitive detection of cadmium ions in water. Mater. Chem. Phys. 284 , 126064 (2022)
L. Wen, J. Dong, H. Yang, J. Zhao, Z. Hu, H. Han, C. Hou, X. Luo, D. Huo, A novel electrochemical sensor for simultaneous detection of Cd 2+ and Pb 2+ by MXene aerogel-CuO/carbon cloth flexible electrode based on oxygen vacancy and bismuth film. Sci. Total. Environ. 851 , 158325 (2022)
F. Miao, X. Guan, B. Tao, Y. Zang, Pd/ZnS/ZnO sensitive selective detection photoelectrochemical sensor for the detection of Cd 2+ . Vacuum 211 , 111972 (2023)
V.K. Gupta, L.P. Singh, R. Singh, N. Upadhyay, S.P. Kaur, B. Sethi, A novel copper (II) selective sensor based on dimethyl 4, 4′(o-phenylene) bis (3-thioallophanate) in PVC matrix. J. Mol. Liq. 174 , 11–16 (2012)
T.M. Lima, P.I. Soares, L.A. do Nascimento, D.L. Franco, A.C. Pereira, L.F. Ferreira, A novel electrochemical sensor for simultaneous determination of cadmium and lead using graphite electrodes modified with poly (p-coumaric acid). Microchem. J. 168 , 106406 (2021)
D.C. Marcano, D.V. Kosynkin, J.M. Berlin, A. Sinitskii, Z. Sun, A. Slesarev, L.B. Alemany, W. Lu, J.M. Tour, Improved synthesis of graphene oxide. ACS Nano 4 (8), 4806–4814 (2010)
B. Feng, J. Cao, D. Han, H. Liang, S. Yang, X. Li, J. Yang, ZnSe nanoparticles of different sizes: optical and photocatalytic properties. Mater. Sci. Semicond. Process. 27 , 865–872 (2014)
P. Kumar, J. Singh, M.K. Pandey, C.E. Jeyanthi, R. Siddheswaran, M. Paulraj, K.N. Hui, K.S. Hui, Synthesis, structural, optical and Raman studies of pure and lanthanum doped ZnSe nanoparticles. Mater. Res. Bull. 49 , 144–150 (2014)
L. Yang, J. Zhu, D. Xiao, Microemulsion-mediated hydrothermal synthesis of ZnSe and Fe-doped ZnSe quantum dots with different luminescence characteristics. RSC Adv. 2 (21), 8179–8188 (2012)
B. Canava, J. Vigneron, A. Etcheberry, J.F. Guillemoles, D. Lincot, High resolution XPS studies of Se chemistry of a Cu (In, Ga) Se2 surface. Appl. Surf. Sci. 202 (1–2), 8–14 (2002)
D. Briggs, G. Beamson, XPS studies of the oxygen 1s and 2s levels in a wide range of functional polymers. Anal. Chem. 65 (11), 1517–1523 (1993)
P. Mondal, A. Sinha, N. Salam, A.S. Roy, N.R. Jana, S.M. Islam, Enhanced catalytic performance by copper nanoparticle–graphene based composite. RSC Adv. 3 (16), 5615–5623 (2013)
M.B. Gumpu, S. Sethuraman, U.M. Krishnan, J.B.B. Rayappan, A review on detection of heavy metal ions in water—an electrochemical approach. Sens. Actuators B Chem. 213 , 515–533 (2015)
Q. Ding, C. Li, H. Wang, C. Xu, H. Kuang, Electrochemical detection of heavy metal ions in water. Chem. Commun. 57 (59), 7215–7231 (2021)
A. Singh, A. Sharma, S. Arya, Human sweat-based wearable glucose sensor on cotton fabric for real-time monitoring. J. Anal. Sci. Technol. 13 (1), 1–13 (2022)
M. Abdulla, A. Ali, R. Jamal, T. Bakri, W. Wu, T. Abdiryim, Electrochemical sensor of double-thiol linked PProDOT@ Si composite for simultaneous detection of Cd (II), Pb (II), and Hg (II). Polymers 11 (5), 815 (2019)
F. Gao, N. Gao, A. Nishitani, H. Tanaka, Rod-like hydroxyapatite and Nafion nanocomposite as an electrochemical matrix for simultaneous and sensitive detection of Hg 2+ , Cu 2+ , Pb 2+ and Cd 2+ . J. Electroanal. Chem. 775 , 212–218 (2016)
M. Liu, Q. Guan, S. Liu, Nitrogen-doped hollow carbon spheres for electrochemical detection of heavy metal ions. Ionics 24 (9), 2783–2793 (2018)
M.B. Gumpu, M. Veerapandian, U.M. Krishnan, J.B.B. Rayappan, Simultaneous electrochemical detection of Cd (II), Pb (II), As (III) and Hg (II) ions using ruthenium (II)-textured graphene oxide nanocomposite. Talanta 162 , 574–582 (2017)
Y.L. Xie, S.Q. Zhao, H.L. Ye, J. Yuan, P. Song, S.Q. Hu, Graphene/CeO 2 hybrid materials for the simultaneous electrochemical detection of cadmium (II), lead (II), copper (II), and mercury (II). J. Electroanal. Chem. 757 , 235–242 (2015)
S. Xiong, B. Yang, D. Cai, G. Qiu, Z. Wu, Individual and simultaneous stripping voltammetric and mutual interference analysis of Cd 2+ , Pb 2+ and Hg 2+ with reduced graphene oxide-Fe 3 O 4 nanocomposites. Electrochim. Acta 185 , 52–61 (2015)
S. Ding, A. Ali, R. Jamal, L. Xiang, Z. Zhong, T. Abdiryim, An electrochemical sensor of poly (EDOT-pyridine-EDOT)/graphitic carbon nitride composite for simultaneous detection of Cd 2+ and Pb 2+ . Materials 11 (5), 702 (2018)
Z. Zhong, A. Ali, R. Jamal, R. Simayi, L. Xiang, S. Ding, T. Abdiryim, Poly (EDOT-pyridine-EDOT) and poly (EDOT-pyridazine-EDOT) hollow nanosphere materials for the electrochemical detection of Pb 2+ and Cu 2+ . J. Electroanal. Chem. 822 , 112–122 (2018)
H. Xing, J. Xu, X. Zhu, X. Duan, L. Lu, W. Wang, Y. Zhang, T. Yang, Highly sensitive simultaneous determination of cadmium (II), lead (II), copper (II), and mercury (II) ions on N-doped graphene modified electrode. J. Electroanal. Chem. 760 , 52–58 (2016)
Y. Ma, Y. Wang, D. Xie, Y. Gu, X. Zhu, H. Zhang, G. Wang, Y. Zhang, H. Zhao, Hierarchical MgFe-layered double hydroxide microsphere/graphene composite for simultaneous electrochemical determination of trace Pb (II) and Cd (II). Chem. Eng. J. 347 , 953–962 (2018)
L. Cao, J. Jia, Z. Wang, Sensitive determination of Cd and Pb by differential pulse stripping voltammetry with in situ bismuth-modified zeolite doped carbon paste electrodes. Electrochim. Acta 53 (5), 2177–2182 (2008)
E. Tesarova, L. Baldrianova, S.B. Hocevar, I. Svancara, K. Vytras, B. Ogorevc, Anodic stripping voltammetric measurement of trace heavy metals at antimony film carbon paste electrode. Electrochim. Acta 54 (5), 1506–1510 (2009)
A. Afkhami, T. Madrakian, S.J. Sabounchei, M. Rezaei, S. Samiee, M. Pourshahbaz, Construction of a modified carbon paste electrode for the highly selective simultaneous electrochemical determination of trace amounts of mercury (II) and cadmium (II). Sens. Actuators B Chem. 161 (1), 542–548 (2012)
P. Zhang, S. Dong, G. Gu, T. Huang, Simultaneous determination of Cd 2+ , Pb 2+ , Cu 2+ and Hg 2+ at a carbon paste electrode modified with ionic liquid-functionalized ordered mesoporous silica. Bull. Korean Chem. Soc. 31 (10), 2949–2954 (2010)
P.K.Q. Nguyen, S.K. Lunsford, Electrochemical response of carbon paste electrode modified with mixture of titanium dioxide/zirconium dioxide in the detection of heavy metals: lead and cadmium. Talanta 101 , 110–121 (2012)
M.I. Ansari, A. Malik, Biosorption of nickel and cadmium by metal resistant bacterial isolates from agricultural soil irrigated with industrial wastewater. Bioresour. Technol. 98 (16), 3149–3153 (2007)
K.K. Singh, R. Rastogi, S.H. Hasan, Removal of cadmium from wastewater using agricultural waste ‘rice polish.’ J. Hazard. Mater. 121 (1–3), 51–58 (2005)
V.K. Gupta, C.K. Jain, I. Ali, M. Sharma, V.K. Saini, Removal of cadmium and nickel from wastewater using bagasse fly ash—a sugar industry waste. Water Res. 37 (16), 4038–4044 (2003)
Download references
Acknowledgements
This work was supported by the Science and Engineering Research Board (SERB), India (File no. EEQ/2021/000172).
Author information
Authors and affiliations.
School of Sciences, Cluster University of Jammu, Jammu, Jammu and Kashmir, 180001, India
Bhawani Sharma
Department of Humanities and Sciences, Guru Nanak Institutions Technical Campus, Hyderabad, 501506, India
Anoop Singh
Centre for Global Health Research, Saveetha Medical College and Hospitals, Saveetha Institute of Medical and Technical Sciences, Chennai, Tamil Nadu, 602105, India
Department of Physics, G.L.D.M Degree College, Hiranagar, Kathua, Jammu and Kashmir, 184142, India
Asha Sharma
Department of Physics, University of Jammu, Jammu, Jammu and Kashmir, 180006, India
Aman Dubey & Sandeep Arya
Department of Physics, Khalifa University, 127788, Abu Dhabi, United Arab Emirates
Vinay Gupta
Azerbaijan State Oil and Industry University, Azadlig Ave. 20, AZ-1010, Baku, Azerbaijan
Rashad Gabil Oglu Abaszade
Department of Prosthodontics, Saveetha Dental College and Hospitals, Saveetha Institute of Medical and Technical Sciences, Poonamallee High Road, Velappanchavadi, Chennai, Tamil Nadu, 600077, India
Ashok K. Sundramoorthy
Department of Physics, School of Basic Sciences, Abhilashi University, Chailchowk, Teh. Chachyot, Mandi, HP, 175045, India
Navdeep Sharma
You can also search for this author in PubMed Google Scholar
Contributions
Bhawani Sharma: conceptualization, validation, visualization, writing—review and editing. Anoop Singh: conceptualization, methodology, analysis, writing. Asha Sharma: characterization, writing—review and editing. Aman Dubey: writing—review and editing. Vinay Gupta: writing—review and editing. Rashad Gabil Oglu Abaszade: writing—review and editing. Ashok K. Sundramoorthy: writing—review and editing. Navdeep Sharma: writing—review and editing. Sandeep Arya: conceptualization, supervision, methodology, writing—review and editing.
Corresponding author
Correspondence to Sandeep Arya .
Ethics declarations
Conflict of interest.
The authors declare that they have no known competing financial interests or personal relationships that could have appeared to influence the work reported in this paper.
Ethical approval
Not applicable.
Additional information
Publisher's note.
Springer Nature remains neutral with regard to jurisdictional claims in published maps and institutional affiliations.
Supplementary Information
Below is the link to the electronic supplementary material.
Supplementary file1 (DOCX 397 KB)
Rights and permissions.
Springer Nature or its licensor (e.g. a society or other partner) holds exclusive rights to this article under a publishing agreement with the author(s) or other rightsholder(s); author self-archiving of the accepted manuscript version of this article is solely governed by the terms of such publishing agreement and applicable law.
Reprints and permissions
About this article
Sharma, B., Singh, A., Sharma, A. et al. Synthesis and characterization of zinc selenide/graphene oxide (ZnSe/GO) nanocomposites for electrochemical detection of cadmium ions. Appl. Phys. A 130 , 297 (2024). https://doi.org/10.1007/s00339-024-07472-0
Download citation
Received : 12 February 2024
Accepted : 21 March 2024
Published : 08 April 2024
DOI : https://doi.org/10.1007/s00339-024-07472-0
Share this article
Anyone you share the following link with will be able to read this content:
Sorry, a shareable link is not currently available for this article.
Provided by the Springer Nature SharedIt content-sharing initiative
- Electrochemical sensing
- Nanocomposite
- Graphene oxide
- Find a journal
- Publish with us
- Track your research
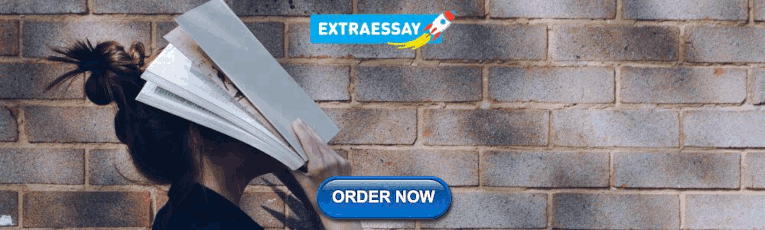
IMAGES
VIDEO
COMMENTS
Graphene oxide (GO) has attracted much attention in the past few years because of its interesting and promising electrical, thermal, mechanical, and structural properties. These properties can be altered, as GO can be readily functionalized. Brodie synthesized the GO in 1859 by reacting graphite with KClO3 in the presence of fuming HNO3; the reaction took 3-4 days to complete at 333 K. Since ...
4. Comparison of Graphene Oxide with Other 2D Materials. Recent research has centered on the creation of semiconductor nanocomposite materials based on 2D reduced GO (rGO) to enhance its catalytic uses (Table 4). Apple juice and zinc acetate were used to produce in situ 2D rGO-ZnO (rGZn) nanocomposites, which is a commonly reported green ...
While graphite oxide is a multilayered system, the graphene oxide is a few- or a single-layer system [1, 14, 15]. The graphite oxide interlayer separation is 6.35 Å. However, in liquid, it increases from 6.35 to 11.6 Å. The characteristics of graphene oxide can be altered by functionalization on the basis of the specific application.
The earliest synthesis of graphene oxide (GO) was achieved by chemist B. Brodie at the University of Oxford, in 1859 4 — over a century before the discovery of modern carbon materials such as ...
In this review, graphene oxide (GO), reduced graphene oxide (rGO), and functionalized GO (FGO) are noted among the various allotropic forms of carbons due to their significant surface area, strength, light weight, chemical stability, enhanced cell adhesion, proliferation, differentiation, and application in the repair of tissue [4,5].The basic structure of all carbon allotropes is graphene; it ...
The primary driver of intense research into graphene centres on its highly desirable electrical, thermal, optical, and mechanical properties and the possibility of tuning these for desired ...
Graphene oxide (GO) has attracted intensive research interest, owing to remarkable physicochemical properties. Nevertheless, its high chemical reactivity and low stability may lead to uncontrolled ...
1. Introduction. Graphene oxide (GO) is the oxidized analogy of graphene, recognized as the only intermediate or precursor for obtaining the latter in large scale, [1] since the English chemist, sir Brodie first reported about the oxidation of graphite centuries ago [2].About thirty years ago, the term graphene was officially claimed to define the single atom-thin carbon layer of graphite [3 ...
1. INTRODUCTION. Graphene (G), the two‐dimensional and hexagonally bonded sp 2 hybridized carbon structure with extraordinary characteristics, has garnered huge interdisciplinary attention in different fields of science and engineering over the last half‐century. The single, multi‐layered (less than 10) and flatted honeycomb structure of G possesses unique attributes namely high hardness ...
Graphene oxide (GO) is a two-dimensional (2D) nanomaterial comprising single-layer sheets of sp 2 hybridized carbons, sites of sp 3 hybridized carbons, and oxygenated groups, obtained from the oxidation and exfoliation of graphite [].First synthesized by British chemist B.C. Brodie in 1859, GO is obtained by chemical treatment of graphite flakes using strong oxidizers followed by dispersion ...
Research team introduces new non-toxic method for producing high-quality graphene oxide. Feb 20, 2024.
1. Introduction. Graphene is an atomically-thin, 2-dimensional (2D) sheet of sp 2 carbon atoms in a honeycomb structure. It has been shown to have many desirable properties such as high mechanical strength [1], electrical conductivity [2], molecular barrier abilities [3] and other remarkable properties.For these reasons, it has been the goal of countless research efforts to incorporate ...
With a fast-rising population, the amplification of new resources, skills, and devices that can provide safe potable water and clean energy remains one of the vital research prominences for the scientific community. Owing to this, new materials with graphene functionalized derivatives show significant improv Recent Review Articles
Abstract— We review state-of-the-art progress in the field of graphene oxide, a material that has a wide range of applications due to its unique properties, for example, the dielectric properties of graphene oxide can be used in memristors, the use of different degrees of reduction allows creating multipolar electrodes of lithium-ion batteries and supercapacitors, various oxygen-containing ...
Microwave heating is widely used for the exfoliation and reduction of graphitic precursor materials. With microwave treatments, the preparation of graphene can be performed by solid-state reduction of dry graphite oxide, the reduction of graphite oxide in suspension, or by heating graphite interca-lated compounds (GICs).
Graphene-based nanomaterials have attracted remarkable attention during the last decade in nanotechnology. In this review, we discuss the hybrid nanomaterials based on graphene oxide (GO) and nanoparticles (NPs). We review the synthesis, properties, and applications of immobilized transition metal oxide (TMO) NPs on graphene/GO. We present the TMO NPs immobilizing methods on graphene/GO using ...
Graphene oxide (GO), a derivative of graphene, is a novel carbon material that has attracted a lot of attention in the field of membrane materials as its ability to achieve layer-by-layer stacking and the formation of nanochannels between the lamellae makes it excellent for selective separation of substances. In this paper, the separation mechanism of the GO membrane is summarized. According ...
Due to their unique physicochemical properties, graphene-family nanomaterials (GFNs) are widely used in many fields, especially in biomedical applications. Currently, many studies have investigated the biocompatibility and toxicity of GFNs in vivo and in intro. Generally, GFNs may exert different degrees of toxicity in animals or cell models by following with different administration routes ...
2.3. Preparation of Reduced Graphene Oxide via Thermal Reduction. The thermal reduction was carried out by heating the lyophilized GO in an atmo-sphere of N2 (flow of 0.12 m3/h) using an oven at 750 C and 1000 C. After reaching the temperature, the GO was kept at a constant temperature for 45 s [37]. 2.4.
Graphene oxide (GO) is a member of a family of two-dimensional (2D) materials, derived from the oxidation of 2D graphitic structures (sp 2 to sp 3 carbon conversion). As any other 2D material, in ...
1. Introduction. Graphene is one of the most studied materials in the world; thanks to its unique properties, it was called a "material of the future" [].Graphene consists only of carbon atoms where every carbon atom is attached to three other carbon atoms with sp 2 hybridized orbitals making a honeycomb lattice [].Graphene's rare properties make it a very promising material for a huge ...
As a functionalized 2D material for the synthesis of graphene and its composites, graphene oxide (GO) contains hydroxyl, carbonyl and epoxy groups on the basal plane in addition to carboxyl groups at the edges (Fig. 1). 1-5 These oxygen-containing functional groups endow GO with excellent dispersibility in various solvents. 6,7 Moreover, GO can be functionalized by chemical bonds, compounded ...
Between the sheets: The Intercalation-Exfoliation (I-E) method was successfully applied to produce graphene oxide (GO) materials with adjustable oxidation levels, defect density, and crystallite size by tuning applied potential and electrolyte concentration.Investigating the electrochemical properties of these GO samples via CV and EIS measurements with a selected redox probe establishes ...
There has been an upsurge of green reductants for the preparation of graphene materials taking consideration of human health and the environment in recent years. In this paper, reduced graphene oxides (RGOs) were prepared by chemical reduction of graphene oxide (GO) with three green reductants, L-ascorbic acid (L-AA), D-glucose (D-GLC) and tea polyphenol (TP), and comparatively characterized ...
The rapid proliferation of antibiotic resistance genes (ARGs) in the environment has become a major concern. However, the effect of graphene oxide (GO), a newly recognized pollutant, on the transfer of ARGs among bacteria remains poorly understood. In this study, we investigated the influence of GO at concen Environmental Science: Nano Recent HOT Articles
Research Article. A critical review on the effect of graphene oxide in fiber reinforced cementitious systems. Raj Samraj a School of Civil Engineering, ... Recently, the Graphene Oxide (GO) nanomaterial has grabbed a lot of attention in the material science of building materials. These graphene oxide-based cementitious composites have been ...
Researchers led by Long Ju, a condensed-matter physicist at the Massachusetts Institute of Technology (MIT) in Cambridge, saw the effect when they sandwiched five layers of graphene between sheets ...
Titanate whiskers/cardanol-based epoxy coatings with ultra-high anticorrosion performance using graphene oxide (GO) as a 2D lightweight anti-settling agent were prepared. ... Ding JH, Yu HB. Research in graphene-based anticorrosion coatings. Prog Chem 2016; 28: 737-743. Crossref. Google Scholar. 10. Zhou LS, Zhang PB, Shen LM, et al. Modified ...
In this study, graphene oxide was reduced at different temperatures and used for degrading indigo carmine and neutral red dyes. The wide surface areas, strong adsorption sites, and oxygen ...
It consists of the chemical exfoliation of graphite powder and is a standard method for the production of graphene oxide. 0.9 g graphite powder and 5.4 g potassium permanganate (KMnO 4) were, for a limited time, brought into a highly concentrated acid combination of 162 mL H 2 SO 4 and 18 mL H 3 PO 4, resulting in a mild exothermic reaction at ...