Fabrication and synthesis of SnO X thin films: a review
- ORIGINAL ARTICLE
- Published: 03 November 2020
- Volume 111 , pages 2809–2831, ( 2020 )
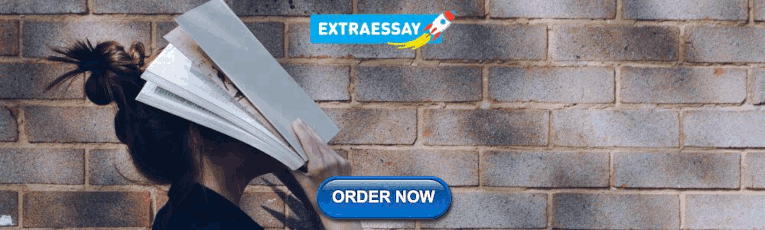
Cite this article
- Emeka Charles Nwanna 1 ,
- Patrick Ehi Imoisili 1 &
- Tien-Chien Jen ORCID: orcid.org/0000-0003-1743-4668 1
1160 Accesses
12 Citations
Explore all metrics
Due to its exceptional electrical, optical, chemical and magnetic properties, tin oxide (SnO and SnO 2 ), which is a functional material, has gained enormous attention for use in a variety of applications. Films of SnO X have a direct band gap between the ranges of 2.2 and 3.6 eV, with these films finding usefulness in various functions such as solar cells, transparent conducting oxides for gas sensors, lithium-ion batteries and microelectronics, and use in the optoelectronics industries. In order to satisfy the needs of a broad range of these applications, thin films with an extensive properties span defined by film composition, thickness, structural properties and morphology are required. This article explains the theory and research status of the various manufacturing processes of tin oxide. The purpose is to analyze the effects of the thin films through distinct forms of deposition. The general finding summarized in this research on SnO X showed that various researchers studied specific characteristics of tin oxide properties restricted by experimental conditions.
This is a preview of subscription content, log in via an institution to check access.
Access this article
Price includes VAT (Russian Federation)
Instant access to the full article PDF.
Rent this article via DeepDyve
Institutional subscriptions
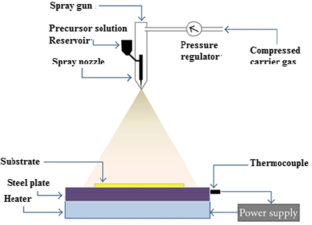
Similar content being viewed by others
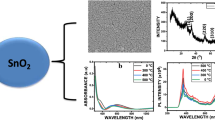
Synthesis and characterization of tin (IV) oxide thin films
M. Rahayi, M. H. Ehsani, … Fabian I. Ezema
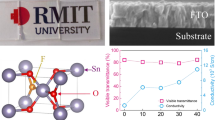
Structural, Optical, Electrical, and Nanomechanical Properties of F-Doped Sno2 Fabricated by Ultrasonic Spray Pyrolysis
Jaewon Kim, Gahui Kim & Young-Bae Park
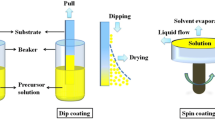
Influence of deposition techniques on quality and photodetection properties of tin disulfide (SnS2) thin films
Ankurkumar J. Khimani, Sunil H. Chaki, … M. P. Deshpande
Epifani M, Francioso L, Siciliano P, Helwig A, Mueller G, Díaz R, Arbiol J, Morante JR (2007) SnO2 thin films from metalorganic precursors: Synthesis, characterization, microelectronic processing and gas-sensing properties. Sensors Actuators B Chem 124(1):217–226. https://doi.org/10.1016/j.snb.2006.12.029
Article Google Scholar
Göpel W, Schierbaum KD (1995) SnO2 sensors: current status and future prospects. Sensors Actuators B Chem 26(1-3):1–12. https://doi.org/10.1016/0925-4005(94)01546-t
Aswathy BR, Vinay K, Arjun M, Manoj PK. (2019) Deposition of tin oxide thin film by sol-gel dip coating technique and its characterization. Proceedings of the international conference on advanced materials: ICAM 2019 [Internet]. AIP Publishing; 2019; Available from: https://doi.org/10.1063/1.5130344
McAleer JF, Moseley PT, Norris JOW, Williams DE, Taylor P, Tofield BC (1997) Tin oxide based gas sensors. Mater Chem Phys 17(6):577–583. https://doi.org/10.1016/0254-0584(87)90017-4
Zhitomirsky VN, David T, Boxman RL, Goldsmith S, Verdyan A, Soifer YM, Rapoport L (2005) Properties of SnO2 coatings fabricated on polymer substrates using filtered vacuum arc deposition. Thin Solid Films 492(1-2):187–194. https://doi.org/10.1016/j.tsf.2005.06.061
Albin D, Risbud S (1997) Spray pyrolysis processing of optoelectronic materials. Adv Ceram Mater(USA) 2(3A). https://doi.org/10.1111/j.1551-2916.1987.tb00089.x
Manifacier J, Fillard J, Bind J (1991) Deposition of In2O3 SnO2 layers on glass substrates using a spraying method. Thin Solid Films 77(1-3):67–80. https://doi.org/10.1016/0040-6090(81)90361-8
Chopra K, Major S, Pandya D (1993) Transparent conductors—a status review. Thin Solid Films 102(1):1–46. https://doi.org/10.1016/0040-6090(83)90256-0
Bel Hadj Tahar R, Ban T, Ohya Y, Takahashi Y (1998) Tin doped indium oxide thin films: Electrical properties. J Appl Phys 83(5):2631–2645. https://doi.org/10.1063/1.367025
Studenikin S, Golego N, Cocivera M (1998) Optical and electrical properties of undoped ZnO films grown by spray pyrolysis of zinc nitrate solution. J Appl Phys 83(4):2104–2111. https://doi.org/10.1063/1.366944
Coutts TJ, Wu X, Mulligan WP, Webb JM (1996) High-performance, transparent conducting oxides based on cadmium stannate. J Electron Mater 25(6):935–943. https://doi.org/10.1007/bf02666727
Omura K, Veluchamy P, Tsuji M, Nishio T, Murozono M (1999) A pyrosol technique to deposit highly transparent, low-resistance SnO2: F Thin films from dimethyltin dichloride. J Electrochem Soc 146(6):2113. https://doi.org/10.1002/chin.199939215
Kar A, Kundu S, Patra A (2011) Surface defect-related luminescence properties of SnO2 nanorods and nanoparticles. J Phys Chem C 115(1):118–124. https://doi.org/10.1021/jp110313b
Vijayarangamuthu K, Rath S (2014) Nanoparticle size, oxidation state, and sensing response of tin oxide nanopowders using Raman spectroscopy. J Alloys Compd 610:706–712. https://doi.org/10.1016/j.jallcom.2014.04.187
Calestani D, Zha M, Zappettini A, Lazzarini L, Salviati G, Zanotti L, Sberveglieri G (2005) Structural and optical study of SnO2 nanobelts and nanowires. Mater Sci Eng C 25(5-8):625–630. https://doi.org/10.1016/j.msec.2005.07.014
Nemade KR, Waghuley SA (2014) Comparative study of carbon dioxide sensing by Sn-doped TiO2 nanoparticles synthesized by microwave-assisted and solid-state diffusion route. Appl Nanosci 5(4):419–424. https://doi.org/10.1007/s13204-014-0333-2
Kinumoto T, Nagano K, Yamamoto Y, Tsumura T, Toyoda M (2014) Anticorrosion properties of tin oxide coatings for carbonaceous bipolar plates of proton exchange membrane fuel cells. J Power Sources 249:503–508. https://doi.org/10.1016/j.jpowsour.2013.10.065
Tosun BS, Feist RK, Gunawan A, Mkhoyan KA, Campbell SA, Aydil ES (2012) Sputter deposition of semicrystalline tin dioxide films. Thin Solid Films 520(7):2554–2561. https://doi.org/10.1016/j.tsf.2011.10.169
Behrendt A, Friedenberger C, Gahlmann T, Trost S, Becker T, Zilberberg K, Polywka A, Görrn P, Riedl T (2015) Highly robust transparent and conductive gas diffusion barriers based on tin oxide. Adv Mater 27(39):5961–5967. https://doi.org/10.1002/adma.201502973
Beneking C, Rech B, Wieder S, Kluth O, Wagner H, Frammelsberger W, Geyer R, Lechner P, Rübel H, Schade H (1999) Recent developments of silicon thin film solar cells on glass substrates. Thin Solid Films 351(1-2):241–246. https://doi.org/10.1016/s0040-6090(98)01793-3
Rosental A, Tarre A, Gerst A, Uustare T, Sammelselg V (2001) Atomic-layer chemical vapor deposition of SnO2 for gas-sensing applications. Sensors Actuators B Chem 77(1-2):297–300. https://doi.org/10.1016/s0925-4005(01)00746-8
Jin C, Yamazaki T, Ito K, Kikuta T, Nakatani N (2006) H 2 S sensing property of porous SnO2 sputtered films coated with various doping films. Vacuum 80(7):723–725. https://doi.org/10.1016/j.vacuum.2005.11.002
Yang H, Zhang X, Tang A (2006) Mechanosynthesis and gas-sensing properties of In 2 O 3 /SnO 2 nanocomposites. Nanotechnology 17(12):2860. https://doi.org/10.1088/0957-4484/17/12/006
Hagemeyer A, Hogan Z, Schlichter M, Smaka B, Streukens G, Turner H, Volpe Jr A, Weinberg H, Yaccato K (2007) High surface area tin oxide. Appl Catal A Gen 317(2):139–148. https://doi.org/10.1016/j.apcata.2006.09.040
Hosono H, Ohta H, Orita M, Ueda K, Hirano M (2002) Frontier of transparent conductive oxide thin films. Vacuum 66(3-4):419–425. https://doi.org/10.1016/s0042-207x(02)00165-3
Wang GF, Tao XM, Huang HM (2005) Light-emitting devices for wearable flexible displays. Color Technol 121(3):132–138. https://doi.org/10.1111/j.1478-4408.2005.tb00263.x
Vergöhl M, Malkomes N, Matthee T, Bräuer G, Richter U, Nickol FW, Bruch J (2001) In situ monitoring of optical coatings on architectural glass and comparison of the accuracy of the layer thickness attainable with ellipsometry and photometry. Thin Solid Films 392(2):258–264. https://doi.org/10.1016/s0040-6090(01)01040-9
Chen JS, Lou XW (2013) SnO 2 -based nanomaterials: synthesis and application in lithium-ion batteries. Small 9(11):1877–1893. https://doi.org/10.1002/smll.201202601
Nazarov DV, Maximov MY, Novikov PA, Popovich AA, Silin AO, Smirnov VM et al (2017) Atomic layer deposition of tin oxide using tetraethyltin to produce high-capacity Li-ion batteries. J Vac Sci Technol A 35(1):01B137. https://doi.org/10.1116/1.4972554
Roy P, S.K. (2015) Srivastava, Nanostructured anode materials for lithium ion batteries. J Mater Chem A 3(6):2454–2484. https://doi.org/10.1039/c4ta04980b
Reddy M, Subba Rao G, B. (2013) Chowdari, Metal oxides and oxysalts as anode materials for Li ion batteries. Chem Rev 113(7):5364–5457. https://doi.org/10.1021/cr3001884
Shrotriya V, Li G, Yao Y, Chu CW, Yang Y (2006) Transition metal oxides as the buffer layer for polymer photovoltaic cells. Appl Phys Lett 88(7):073508. https://doi.org/10.1063/1.2174093
Betz U, Olsson MK, Marthy J, Escolá MF, Atamny F (2006) Thin films engineering of indium tin oxide: large area flat panel displays application. Surf Coat Technol 200(20-21):5751–5759. https://doi.org/10.1016/j.surfcoat.2005.08.144
Adnane M, Cachet H, Folcher G, Hamzaoui S (2005) Beneficial effects of hydrogen peroxide on growth, structural and electrical properties of sprayed fluorine-doped SnO2 films. Thin Solid Films 492(1-2):240–247. https://doi.org/10.1016/j.tsf.2005.06.085
Viirola H, Niinistö L (1994) Controlled growth of antimony-doped tin dioxide thin films by atomic layer epitaxy. Thin Solid Films 251(2):127–135. https://doi.org/10.1016/0040-6090(94)90677-7
Aguir K, Bernardini S, Lawson B, Fiorido T (2020) Trends in metal oxide thin films: Synthesis and applications of tin oxide. Tin Oxide Mater:219–246. https://doi.org/10.1016/b978-0-12-815924-8.00008
Benhaoua A, Rahal A, Benhaoua B, Jlassi M (2014) Effect of fluorine doping on the structural, optical and electrical properties of SnO 2 thin films prepared by spray ultrasonic. Superlattice Microst 70:61–69. https://doi.org/10.1016/j.spmi.2014.02.005
Mishra S, Ghanshyam C, Ram N, Singh S, Bajpai RP, Bedi RK (2002) Alcohol sensing of tin oxide thin film prepared by sol-gel process. Bull Mater Sci 25(3):231–234. https://doi.org/10.1007/bf02711159
Batzill M, Diebold U (2005) The surface and materials science of tin oxide. Prog Surf Sci 79(2-4):47–154. https://doi.org/10.1016/j.progsurf.2005.09.002
Das S, Jayaraman V (2014) SnO 2 : A comprehensive review on structures and gas sensors. Prog Mater Sci 66:112–255. https://doi.org/10.1016/j.pmatsci.2014.06.003
Krivetskiy VV, Rumyantseva MN, Gaskov AM (2013) Chemical modification of nanocrystalline tin dioxide for selective gas sensors. Russ Chem Rev 82(10):917. https://doi.org/10.1070/rc2013v082n10abeh004366
Arafat MM, Dinan B, Akbar SA, Haseeb ASMA (2012) Gas sensors based on one dimensional nanostructured metal-oxides: a review. Sensors 12(6):7207–7258. https://doi.org/10.3390/s120607207
Choi G, Satyanarayana L, Park J (2006) Effect of process parameters on surface morphology and characterization of PE-ALD SnO 2 thin films for gas sensing. Appl Surf Sci 252(22):7878–7883. https://doi.org/10.1016/j.apsusc.2005.09.069
Dutaive M, Lalauze R, Pijolat C (1995) Sintering, catalytic effects and defect chemistry in polycrystalline tin oxide. Sensors Actuators B 26:26–27. https://doi.org/10.1016/0925-4005(94)01552-s
Ray SC, Karanjai MK, DasGupta D (1998) Tin dioxide based transparent semiconducting films deposited by the dip-coating technique. Surf Coat Technol 102(1-2):73–80. https://doi.org/10.1016/s0257-8972(97)00561-6
Rahal A, Benhaoua A, Jlassi M, Benhaoua B (2015) Structural, optical and electrical properties studies of ultrasonically deposited tin oxide (SnO 2 ) thin films with different substrate temperatures. Superlattice Microst 86:403–411. https://doi.org/10.1016/j.spmi.2015.08.003
Ichimura M, Shibayama K, Masui K (2004) Fabrication of SnO 2 thin films by a photochemical deposition method. Thin Solid Films 466(1-2):34–36. https://doi.org/10.1016/j.tsf.2004.01.117
Abdul-Hamead AA (2018) Properties of SnO 2 thin films deposited by chemical spray pyrolysis using different precursor solutions. https://doi.org/10.1063/1.5039204
Park JJ, Kim KK, Roy M, Song JK, Park SM (2015) Characterization of SnO2thin films grown by pulsed laser deposition under transverse magnetic field. Rapid Commun Photosci 4(3):50–53. https://doi.org/10.5857/rcp.2015.4.3.50
Shamala K, Murthy L, Rao KN (2004) Studies on tin oxide films prepared by electron beam evaporation and spray pyrolysis methods. Bull Mater Sci 27(3):295–301. https://doi.org/10.1007/bf02708520
Monteiro OC, Mendonca MHM, Pereira MIS, Nogueira JMF (2006) Preparation of lead and tin oxide thin films by spin coating and their application on the electrodegradation of organic pollutants. J Solid-State Electrochem 10(1):41–47. https://doi.org/10.1007/s10008-005-0652-z
Van Mol AMB, Chae Y, McDaniel AH, Allendorf MD (2006) Chemical vapor deposition of tin oxide: fundamentals and applications. Thin Solid Films 502(1-2):72–78. https://doi.org/10.1016/j.tsf.2005.07.247
Ponraj JS, Attolini G, Bosi M (2013) Review on atomic layer deposition and applications of oxide thin films. Critic Rev Solid State Mater Sci 38(3):203–233. https://doi.org/10.1080/10408436.2012.736886
Akl AA (2004) Microstructure and electrical properties of iron oxide thin films deposited by spray pyrolysis. Appl Surf Sci 221(1-4):319–329. https://doi.org/10.1016/s0169-4332(03)00951-6
Murthy L, Rao KK (1999) Thickness dependent electrical properties of CdO thin films prepared by spray pyrolysis method. Bull Mater Sci 22(6):953–957. https://doi.org/10.1007/bf02745685
Blunden SJ, Cusack PA, Hill R (1995) The industrial uses of tin chemicals. Vol. 337. Royal Society of Chemistry, London. https://doi.org/10.1002/ange.19860981142
Book Google Scholar
Pengyi L, Junfang C, Wangdian S (2004) Sheet resistance and gas-sensing properties of tin oxide thin films by Plasma enhanced chemical vapor deposition. Plasma Sci Technol 6(2):2259. https://doi.org/10.1088/1009-0630/6/2/015
Brinzari V, Korotcenkov G, Golovanov V, Schwank J, Lantto V, Saukko S (2002) Morphological rank of nano-scale tin dioxide films deposited by spray pyrolysis from SnCl 4 ·5H 2 O water solution. Thin Solid Films 408(1-2):51–58. https://doi.org/10.1016/s0040-6090(02)00086-x
Saadie J (2014) Influence of thickness on electrical and optical properties of tellurium thin films deposited by chemical spray pyrolysis. Int J Appl Math Electron Comput 3, 96(2):–101. https://doi.org/10.18100/ijamec.27133
Akl AA (2004) Optical properties of crystalline and non-crystalline iron oxide thin films deposited by spray pyrolysis. Appl Surf Sci 233(1-4):307–319. https://doi.org/10.1016/j.apsusc.2004.03.263
Yadav AA (2015) SnO 2 thin film electrodes deposited by spray pyrolysis for electrochemical supercapacitor applications. J Mater Sci Mater Electron 27(2):1866–1872. https://doi.org/10.1007/s10854-015-3965-4
Patil GE, Kajale DD, Gaikwad VB, Jain GH (2012) Spray pyrolysis deposition of nanostructured tin oxide thin films. ISRN Nanotechnol 2012:2012–2015. https://doi.org/10.5402/2012/275872
Sears W, Gee MA (1998) Mechanics of film formation during the spray pyrolysis of tin oxide. Thin Solid Films 165(1):265–277. https://doi.org/10.1016/0040-6090(88)90698-0
Thiagarajan S, Sanmugam A, Vikraman D (2017) Facile methodology of sol-gel synthesis for metal oxide nanostructures. Recent Applications in Sol-Gel Synthesis, p. 1-17. https://doi.org/10.5772/intechopen.68708
Levy D, Zayat M (Eds.). (2015). The Sol-Gel Handbook. https://doi.org/10.1002/9783527670819
López TM, Avnir D, Aegerter MA (2013) Emerging fields in sol-gel science and technology: Springer Science & Business Media. doi https://doi.org/10.1007/978-1-4615-0449-8
Lee SC, Lee JH, Oh TS, Kim YH (2003) Fabrication of tin oxide film by sol–gel method for photovoltaic solar cell system. Sol Energy Mater Sol Cells 75(3-4):481–487. https://doi.org/10.1016/s0927-0248(02)00201-5
Korotcenkov G, Brinzari V, Ivanov M, Cerneavschi A, Rodriguez J, Cirera A, Morante J (2005) Structural stability of indium oxide films deposited by spray pyrolysis during thermal annealing. Thin Solid Films 479(1-2):38–51. https://doi.org/10.1016/j.tsf.2004.11.107
Dislich H, Hussmann E (1991) Amorphous and crystalline dip coatings obtained from organometallic solutions: procedures, chemical processes and products. Thin Solid Films 77(1-3):129–140. https://doi.org/10.1016/0040-6090(81)90369-2
Chatelon JP, Terrier C, Bernstein E, Berjoan R, Roger JA (1994) Morphology of SnO 2 thin films obtaibed by the sol-gel technique. Thin Solid Films 247(2):162–168. https://doi.org/10.1016/0040-6090(94)90794-3
Kumar A, Nanda D (2019) Methods and fabrication techniques of superhydrophobic surfaces, in Superhydrophobic Polymer Coatings. Elsevier. p. 43-75. doi https://doi.org/10.1016/b978-0-12-816671-0.00004-7
Neacşu IA, Nicoară AI, Vasile OR, Vasile BŞ (2016) Inorganic micro- and nanostructured implants for tissue engineering. Nanobiomater Hard Tissue Eng 4:271–295. https://doi.org/10.1016/b978-0-323-42862-0.00009-2
Karanjai MK, Dasgupta D (1997) Preparation and study of sulphide thin films deposited by the dip technique. Thin Solid Films 155(2):309–315. https://doi.org/10.1016/0040-6090(87)90075-7
Zhao J, Huo LH, Gao S, Zhao H, Zhao JG (2006) Alcohols and acetone sensing properties of SnO 2 thin films deposited by dip-coating. Sensors Actuators B Chem 115(1):460–464. https://doi.org/10.1016/j.snb.2005.10.024
Dominguez M, Luna-Lopez JA, Flores FJ (2017) Semiconductor materials by ultrasonic spray pyrolysis and their application in electronic devices. Pyrolysis, p. 251. doi https://doi.org/10.5772/67548
Alarcon-Flores G, Aguilar-Frutis MIGUEL, García-Hipolito MANUEL, Guzman-Mendoza J, Canseco MA, Falcony C (2008) Optical and structural characteristics of Y 2 O 3 thin films synthesized from yttrium acetylacetonate. J Mater Sci 43(10):3582–3588. https://doi.org/10.1007/s10853-008-2566-5
Sánchez-García MA, Maldonado A, Castañeda L, Silva-González R, de la Luz Olvera M (2012) Characteristics of SnO 2 : F thin films deposited by ultrasonic spray pyrolysis: effect of water content in solution and substrate temperature. Mater Sci Appl 3(10):690–696. https://doi.org/10.4236/msa.2012.310101
Jadsadapattarakul D, Euvananont C, Thanachayanont C, Nukeaw J, Sooknoi T (2008) Tin oxide thin films deposited by ultrasonic spray pyrolysis. Ceram Int 34(4):1051–1054. https://doi.org/10.1016/j.ceramint.2007.09.096
Park H-H, Park H-H, Hill RH (2004) Stacking effect on the ferroelectric properties of PZT/PLZT multilayer thin films formed by photochemical metal-organic deposition. Appl Surf Sci 237(1-4):427–432. https://doi.org/10.1016/j.apsusc.2004.06.103
Gao M, Hill RH (1998) High efficiency photoresist-free lithography of UO 3 patterns from amorphous films of uranyl complexes. J Mater Res 13(5):1379–1389. https://doi.org/10.1557/jmr.1998.0196
Park H-H, Kim WS, Yang J-K, Park H-H, Hill RH (2004) Characterization of PLZT thin film prepared by photochemical deposition using photosensitive metal-organic precursors. Microelectron Eng 71(2):215–220. https://doi.org/10.1016/j.mee.2003.11.004
Park H-H, Park H-H, Hill RH (2006) Direct-patterning of SnO 2 thin film by photochemical metal-organic deposition. Sensors Actuators A Phys 132(2):429–433. https://doi.org/10.1016/j.sna.2006.02.030
Ichimura M, Goto F, Arai E (1999) Photochemical deposition of CdS from aqueous solutions. J Electrochem Soc 146(3):1028. https://doi.org/10.1149/1.1391716
Ichimura M, Takeuchi K, Nakamura A, Arai E (2001) Photochemical deposition of Se and CdSe films from aqueous solutions. Thin Solid Films 384(2):157–159. https://doi.org/10.1016/s0040-6090(00)01826-5
Sasaki H, Shibayama K, Ichimura M, Masui K (2002) Preparation of (Bi, Sb) 2S3 semiconductor films by photochemical deposition method. J Cryst Growth 237:2125–2129. https://doi.org/10.1016/s0022-0248(01)02280-1
Bravo-Vasquez JP, Hill RH (2000) Photolithographic deposition of conducting gold films from thin amorphous films of AuPR 3 X (X = NO 3 , RCO 2 ) on silicon surfaces. Polyhedron. 19(3):343–349. https://doi.org/10.1016/s0277-5387(99)00364-2
Avey AA, Hill RH (1996) Solid state photochemistry of Cu 2 (OH 2 ) 2 (O 2 C (CH 2 ) 4CH 3 ) 4 in thin films: The photochemical formation of high-quality films of copper and copper (I) oxide. Demonstration of a novel lithographic technique for the patterning of copper. J Am Chem Soc 118(1):237–238. https://doi.org/10.1021/ja952937j
Park HH, Yoon S, Park HH, Hill RH (2004) Electrical properties of PZT thin films by photochemical deposition. Thin Solid Films 447:669–673. https://doi.org/10.1016/j.tsf.2003.09.005
Law W, Hill RH (2000) Photolithographic deposition of insulating Al 2 O 3 films from thin amorphous films of aluminum complexes on silicon surfaces. Thin Solid Films 375(1-2):42–45. https://doi.org/10.1016/s0040-6090(00)01177-9
Ando M, Suto S, Suzuki T, Tsuchida T, Nakayama C, Miura N, Yamazoe N (1994) H2S-sensitive thin film fabricated from hydrothermally synthesized SnO 2 sol. J Mater Chem 4(4):631. https://doi.org/10.1039/jm9940400631
Baik NS, Sakai G, Miura N, Yamazoe N (2000) Hydrothermally treated sol solution of tin oxide for thin-film gas sensor. Sensors Actuators B Chem 63(1-2):74–79. https://doi.org/10.1016/s0925-4005(99)00513-4
Ren X, Pang L, Zhang Y, Ren X, Fan H, Liu SF (2015) One-step hydrothermal synthesis of monolayer MoS 2 quantum dots for highly efficient electrocatalytic hydrogen evolution. J Mater Chem A 3(20):10693–10697. https://doi.org/10.1039/c5ta02198g
Chen Q, Qian Y, Chen Z, Zhou G, Zhang Y (1995) Fabrication of ultrafine SnO 2 thin films by the hydrothermal method. Thin Solid Films 264(1):25–27. https://doi.org/10.1016/0040-6090(95)06586-5
Baik, Seok N, Sakai G, Miura N, Yamazoe N (2000) Preparation of stabilized nanosized tin oxide particles by hydrothermal treatment. J Am Ceram Soc 83(12):2983–2987. https://doi.org/10.1111/j.1151-2916.2000.tb01670.x
Jackson T, Palmer S (1994) Oxide superconductor and magnetic metal thin film deposition by pulsed laser ablation: a review. J Phys D Appl Phys 27(8):1581. https://doi.org/10.1088/0022-3727/27/8/001
Torrisi L, Margarone D (2006) Investigations on pulsed laser ablation of Sn at 1064 nm wavelength. Plasma Sources Sci Technol 15(4):635. https://doi.org/10.1088/0963-0252/15/4/007
Handbook of Physical Vapor Deposition (PVD) Processing. (2010) https://doi.org/10.1016/c2009-0-18800-1
Koinkar V, Ogale S (1991) Pulsed excimer laser processing of optical thin films. Thin Solid Films 206(1-2):259–263. https://doi.org/10.1016/0040-6090(91)90432-w
Dawar AL, Kumar A, Sharma S, Tripathi KN, Mathur PC (1993) Effect of laser irradiation on structural, electrical and optical properties of SnO 2 films. J Mater Sci 28(3):639–644. https://doi.org/10.1007/bf01151238
Galindo H, Vincent AB, Sánchez-R JC, Laude LD (1993) Excimer laser processing for surface improvement of tin oxide thin films. J Appl Phys 74(1):645–648. https://doi.org/10.1063/1.355226
Kunz R, Rothschild M, Ehrlich D (1998) Selective—area laser photodeposition of transparent conductive SnO 2 films. MRS Online Proceedings Library Archive. 129. doi https://doi.org/10.1557/proc-129-447
Treece RE, Horwitz JS, Claassen JH, Chrisey DB (1994) Pulsed laser deposition of high-quality NbN thin films. Appl Phys Lett 65(22):2860–2862. https://doi.org/10.1063/1.112516
Kim H, Gilmore AC, Pique A, Horwitz JS, Mattoussi H, Murata H, ..., Chrisey DB (1999) Electrical, optical, and structural properties of indium–tin–oxide thin films for organic light-emitting devices. J Appl Phys 86(11):6451-6461. doi https://doi.org/10.1063/1.371708
Kim H, Horwitz JS, Kushto GP, Kafafi ZH, Chrisey DB (2001) Indium tin oxide thin films grown on flexible plastic substrates by pulsed-laser deposition for organic light-emitting diodes. Appl Phys Lett 79(3):284–286. https://doi.org/10.1063/1.1383568
Phillips HM, Li Y, Bi Z, Zhang B (1996) Reactive pulsed laser deposition and laser induced crystallization of SnO 2 transparent conducting thin films. Appl Phys A 63(4):347–351. https://doi.org/10.1007/s003390050397
Dolbec R, El Khakani MA, Serventi AM, Trudeau M, Saint-Jacques RG (2002) Microstructure and physical properties of nanostructured tin oxide thin films grown by means of pulsed laser deposition. Thin Solid Films 419(1-2):230–236. https://doi.org/10.1016/s0040-6090(02)00769-1
Xu NS, Huq SE (2005) Novel cold cathode materials and applications. Mater Sci Eng R Rep 48(2-5):47–189. https://doi.org/10.1016/j.mser.2004.12.001
Electron Beam Physical Vapor Deposition (EBPVD). (2019) Encyclopedia of Nanotechnology, 1057–1057. doi https://doi.org/10.1007/978-94-017-9780-1_100290
George J, Menon C (2000) Electrical and optical properties of electron beam evaporated ITO thin films. Surf Coat Technol 132(1):45–48. https://doi.org/10.1016/s0257-8972(00)00726-x
Kachouane A, Addou M, Bougrine A, Messoussi R, Regragui M, Bérnede JC (2001) Preparation and characterisation of tin-doped indium oxide films. Mater Chem Phys 70(3):285–289. https://doi.org/10.1016/s0254-0584(00)00492-2
Park YC, Kim YS, Seo HK, Ansari SG, Shin HS (2002) ITO thin films deposited at different oxygen flow rates on Si (100) using the PEMOCVD method. Surf Coat Technol 161(1):62–69. https://doi.org/10.1016/s0257-8972(02)00476-0
Wang CP, Do KB, Beasley MR, Geballe TH, Hammond RH (1997) Deposition of in-plane textured MgO on amorphous Si 3 N 4 substrates by ion-beam-assisted deposition and comparisons with ion-beam-assisted deposited yttria-stabilized-zirconia. Appl Phys Lett 71(20):2955–2957. https://doi.org/10.1063/1.120227
Ektessabi A, Sato S, Kitamura H, Masaki Y (1993) Simulation of ion beam assisted deposition—a comparison with experimental results. Vacuum 44(3-4):213–217. https://doi.org/10.1016/0042-207x(93)90156-5
Ektessabi AM, Kimura H (1995) Characterization of the surface of bio-ceramic thin films. Thin Solid Films 270(1-2):335–340. https://doi.org/10.1016/0040-6090(95)06714-0
Fursey G (1996) Early field emission studies of semiconductors. Appl Surf Sci 94-95:44–59. https://doi.org/10.1016/0169-4332(95)00518-8
Hossain MF, Naka S, Okada H (2018) Annealing effect of E-beam evaporated TiO 2 films and their performance in perovskite solar cells. J Photochem Photobiol A Chem 360:109–116. https://doi.org/10.1016/j.jphotochem.2018.04.025
Pan XQ, Fu L, Dominguez JE (2001) Structure–property relationship of nanocrystalline tin dioxide thin films grown on (1̄012) sapphire. J Appl Phys 89(11):6056–6061. https://doi.org/10.1063/1.1368866
Nam SC, Kim YH, Cho WI, Cho BW, Chun HS, Yun KS (1998) Charge-discharge performance of electron-beam-deposited tin oxide thin-film electrodes. Electrochem Solid-State Lett 2(1):9. https://doi.org/10.1149/1.1390717
Vuong DD, Sakai G, Shimanoe K, Yamazoe N (2004) Preparation of grain size-controlled tin oxide sols by hydrothermal treatment for thin film sensor application. Sensors Actuators B Chem 103(1-2):386–391. https://doi.org/10.1016/j.snb.2004.04.122
Adedokun O, Odebunmi BM, Sanusi YK (2018) Effect of fluorine doping on the structural, optical and electrical properties of spin coated tin oxide thin films for solar cells application. (2019). Sci Focus J. https://doi.org/10.36293/sfj.2019.0002
Yilbas BS, Al-Sharafi A, Ali H (2019) Surfaces for self-cleaning. Self-Cleaning Surf Water Droplet Mobil 45–98. doi https://doi.org/10.1016/b978-0-12-814776-4.00003-3
Boudrioua A, Chakaroun M, Fischer A (2017) Introduction. Org Lasers, Elsiver. https://doi.org/10.1016/b978-1-78548-158-1.50009-2
Senez V, Thomy V, Dufour R (2014) Nanotechnologies for synthetic super non-wetting surfaces. Nanotechnol Synth Super Non-Wetting Surf:1–12. https://doi.org/10.1002/9781119015093.ch1
Cavicchi RE, Walton RM, Aquino-Class M, Allen JD, Panchapakesan B (2001) Spin-on nanoparticle tin oxide for microhotplate gas sensors. Sensors Actuators B Chem 77(1-2):145–154. https://doi.org/10.1016/s0925-4005(01)00686-4
Gu F, Wang SF, Lü MK, Cheng XF, Liu SW, Zhou GJ, Xu D, Yuan DR (2004) Luminescence of SnO2 thin films prepared by spin-coating method. J Cryst Growth 262(1-4):182–185. https://doi.org/10.1016/j.jcrysgro.2003.10.028
Kääriäinen T, Cameron D, Kääriäinen M-L, Sherman A (2013) Atomic layer deposition: principles, characteristics, and nanotechnology applications. doi https://doi.org/10.1002/9781118747407
Babu Krishna Moorthy S (Ed.) (2015) Thin Film Structures in Energy Applications. doi https://doi.org/10.1007/978-3-319-14774-1
Morosanu CE (1990) Thin film structure. Thin Films by Chemical Vapour Deposition 177–200. doi https://doi.org/10.1016/b978-0-444-98801-0.50013-6
Seshan K, Schepis D (2018) Handbook of thin film deposition, 103. William Andrew. doi https://doi.org/10.1016/b978-0-12-812311-9.00030-x
Manawi Y, Ihsanullah, Samara A, Al-Ansari T, Atieh M (2018) A Review of carbon nanomaterials’ synthesis via the chemical vapor deposition (CVD) method. Materials 11(5):822. https://doi.org/10.3390/ma11050822
Pierson HO (1999) Fundamentals of chemical vapor deposition. Handbook of Chemical Vapor Deposition (CVD), 36–67. doi https://doi.org/10.1016/b978-081551432-9.50005-x
Ho P (1998) Chemical Vapor Deposition for microelectronics: principles, technology and applications. Arthur Sherman (Noyes Publications, 1987). MRS Bull 13(11):78–78. https://doi.org/10.1557/s0883769400064046
George SM, Park BK, Kim CG, Chung T-M (2014) Heteroleptic group 2 metal precursors for metal oxide thin films. Eur J Inorg Chem 2014(11):2002–2010. https://doi.org/10.1002/ejic.201301296
Giunta CJ, Strickler DA, Gordon RG (1993) Kinetic modeling of the chemical vapor deposition of tin oxide from dimethyltin dichloride and oxygen. J Phys Chem 97(10):2275–2283. https://doi.org/10.1021/j100112a032
Yadava Y, Denicoló G, Arias A, Roman L, Hümmelgen I (1997) Preparation and characterization of transparent conducting tin oxide thin film electrodes by chemical vapour deposition from reactive thermal evaporation of SnCl2. Mater Chem Phys 48(3):263–267. https://doi.org/10.1016/s0254-0584(96)01899-8
Buchanan JL, McKown C (1997) Off-line sheet glass coating system. J Non-Cryst Solids 218:179–184. https://doi.org/10.1016/s0022-3093(97)00292-5
Gordon R (1997) Chemical vapor deposition of coatings on glass. J Non-Cryst Solids 218:81–91. https://doi.org/10.1016/s0022-3093(97)00198-1
McCurdy RJ (1999) Successful implementation methods of atmospheric CVD on a glass manufacturing line. Thin Solid Films 351(1-2):66–72. https://doi.org/10.1016/s0040-6090(99)00199-6
Choy K (2003) Chemical vapour deposition of coatings. Prog Mater Sci 48(2):57–170. https://doi.org/10.1016/s0079-6425(01)00009-3
Yusta FJ, Hitchman ML, Shamlian SH (1997) CVD preparation and characterization of tin dioxide films for electrochemical applications. J Mater Chem 7(8):1421–1427. https://doi.org/10.1039/a608525c
Melsheimer J, Ziegler D (1993) Thin tin oxide films of low conductivity prepared by chemical vapour deposition. Thin Solid Films 109(1):71–83. https://doi.org/10.1016/0040-6090(83)90032-9
Houssa M (Ed.) (2004) High k Gate Dielectrics. https://doi.org/10.1201/9781420034141
Heil SBS, van Hemmen JL, Hodson CJ, Singh N, Klootwijk JH, Roozeboom F, … Kessels WMM (2007) Deposition of TiN and HfO[sub 2] in a commercial 200 mm remote plasma atomic layer deposition reactor. J Vacuum Sci Technol A: Vacuum Surf Films 25(5):1357. https://doi.org/10.1116/1.2753846
Ritala M, Leskelä M (2002) Atomic layer deposition. Handbook of Thin Films, 103–159. doi https://doi.org/10.1016/b978-012512908-4/50005-9
Gerritsen E, Emonet N, Caillat C, Jourdan N, Piazza M, Fraboulet D, Boeck B, Berthelot A, Smith S, Mazoyer P (2005) Evolution of materials technology for stacked-capacitors in 65 nm embedded-DRAM. Solid State Electron 49(11):1767–1775. https://doi.org/10.1016/j.sse.2005.10.024
Jakschik S, Schroeder U, Hecht T, Dollinger G, Bergmaier A, Bartha J (2004) Physical properties of ALD-Al2O3 in a DRAM-capacitor equivalent structure comparing interfaces and oxygen precursors. Mater Sci Eng B 107(3):251–254. https://doi.org/10.1016/j.mseb.2003.09.044
Suk Yang W, Kwan Kim Y, Yang S-Y, Hwak Choi J, Soo Park H, In Lee S, Yoo J-B (2000) Effect of SiO2 intermediate layer on Al2O3/SiO2/n + -poly Si interface deposited using atomic layer deposition (ALD) for deep submicron device applications. Surf Coat Technol 131(1-3):79–83. https://doi.org/10.1016/s0257-8972(00)00763-5
Suntola T (1999) Atomic layer epitaxy. Mater Sci Rep 4(5):261–312. https://doi.org/10.1016/s0920-2307(89)80006-4
Kim H (2003) Atomic layer deposition of metal and nitride thin films: Current research efforts and applications for semiconductor device processing. J Vacuum Sci Technol B: Microelectron Nanometer Struct 21(6):2231. https://doi.org/10.1116/1.1622676
Leskelä M, Ritala M (2003) Atomic layer deposition chemistry: recent developments and future challenges. Angew Chem Int Ed 42(45):5548–5554. https://doi.org/10.1002/anie.200301652
Ghosh AP, Gerenser LJ, Jarman CM, Fornalik JE (2005) Thin-film encapsulation of organic light-emitting devices. Appl Phys Lett 86(22):223503. https://doi.org/10.1063/1.1929867
Groner MD, George SM, McLean RS, Carcia PF (2006) Gas diffusion barriers on polymers using Al2O3 atomic layer deposition. Appl Phys Lett 88(5):051907. https://doi.org/10.1063/1.2168489
Agostinelli G, Delabie A, Vitanov P, Alexieva Z, Dekkers HFW, De Wolf S, Beaucarne G (2006) Very low surface recombination velocities on p-type silicon wafers passivated with a dielectric with fixed negative charge. Sol Energy Mater Sol Cells 90(18-19):3438–3443. https://doi.org/10.1016/j.solmat.2006.04.014
Reijnen L, Meester B, Goossens A, Schoonman J (2003) Atomic Layer Deposition of CuxS for Solar Energy Conversion. Chem Vap Depos 9(1):15–20. https://doi.org/10.1002/cvde.200290001
Van TT, Chang JP (2005) Controlled erbium incorporation and photoluminescence of Er-doped Y2O3. Appl Phys Lett 87(1):011907. https://doi.org/10.1063/1.1984082
King JS, Neff CW, Summers CJ, Park W, Blomquist S, Forsythe E, Morton D (2003) High-filling-fraction inverted ZnS opals fabricated by atomic layer deposition. Appl Phys Lett 83(13):2566–2568.10.1063/1 1609240
Mayer TM, Elam JW, George SM, Kotula PG, Goeke RS (2003) Atomic-layer deposition of wear-resistant coatings for microelectromechanical devices. Appl Phys Lett 82(17):2883–2885. https://doi.org/10.1063/1.1570926
Scharf T, Prasad S, Dugger M, Kotula P, Goeke R, Grubbs R (2006) Growth, structure, and tribological behavior of atomic layer-deposited tungsten disulphide solid lubricant coatings with applications to MEMS. Acta Mater 54(18):4731–4743. https://doi.org/10.1016/j.actamat.2006.06.009
Johnson RW, Hultqvist A, Bent SF (2014) A brief review of atomic layer deposition: from fundamentals to applications. Mater Today 17(5):236–246. https://doi.org/10.1016/j.mattod.2014.04.026
George SM (2010) Atomic layer deposition: an overview. Chem Rev 110(1):111–131. https://doi.org/10.1021/cr900056b
Knez M, Nielsch K, Niinistö L (2007) Synthesis and surface engineering of complex nanostructures by atomic layer deposition. Adv Mater 19(21):3425–3438. https://doi.org/10.1002/adma.200700079
Sundqvist J, Lu J, Ottosson M, Hårsta A (2006) Growth of SnO2 thin films by atomic layer deposition and chemical vapour deposition: A comparative study. Thin Solid Films 514(1-2):63–68. https://doi.org/10.1016/j.tsf.2006.02.031
Choi W-S (2009) The fabrication of tin oxide films by atomic layer deposition using tetrakis(ethylmethylamino) tin precursor. Trans Electr Electron Mater 10(6):200–202. https://doi.org/10.4313/teem.2009.10.6.200
Tiznado H, Zaera F (2006) Surface chemistry in the atomic layer deposition of tin films from TiCl4and ammonia. J Phys Chem B 110(27):13491–13498. https://doi.org/10.1021/jp062019f
Aaltonen T, Ritala M, Sajavaara T, Keinonen J, Leskelä M (2003) Atomic layer deposition of platinum thin films. Chem Mater 15(9):1924–1928. https://doi.org/10.1021/cm021333t
Pan D, Guan D, Jen T-C, Yuan C (2016) Atomic layer deposition process modeling and experimental investigation for sustainable manufacturing of nano thin films. J Manuf Sci Eng 138(10). https://doi.org/10.1115/1.4034475
Bachmann J (Ed.) (2017) Atomic layer deposition in energy conversion applications. doi https://doi.org/10.1002/9783527694822
Shaeri MR, Jen T-C, Yuan CY (2015) Reactor scale simulation of an atomic layer deposition process. Chem Eng Res Des 94:584–593. https://doi.org/10.1016/j.cherd.2014.09.019
Marquardt AE, Breitung EM, Drayman-Weisser T, Gates G, Phaneuf RJ (2015) Protecting silver cultural heritage objects with atomic layer deposited corrosion barriers. Herit Sci 3(1):37. https://doi.org/10.1186/s40494-015-0066-x
Farmer DB, Gordon RG (2005) ALD of high-κ dielectrics on suspended functionalized SWNTs. Electrochem Solid-State Lett 8(4):G89. https://doi.org/10.1149/1.1862474
Nwanna EC, Coetzee RAM, Jen T-C (2019) Investigating the purge flow rate in a reactor scale simulation of an atomic layer deposition process. Volume 2B: Advanced Manufacturing. doi https://doi.org/10.1115/imece2019-10692
Lim BS, Rahtu A, Gordon RG (2003) Atomic layer deposition of transition metals. Nat Mater 2(11):749–754. https://doi.org/10.1038/nmat1000
Aaltonen T, Ritala M, Sammelselg V, Leskelä M (2004) Atomic layer deposition of iridium thin films. J Electrochem Soc 151(8):G489. https://doi.org/10.1149/1.1761011
Wang X, Tabakman SM, Dai H (2008) Atomic layer deposition of metal oxides on pristine and functionalized graphene. J Am Chem Soc 130(26):8152–8153. https://doi.org/10.1021/ja8023059
Frank MM, Wilk GD, Starodub D, Gustafsson T, Garfunkel E, Chabal YJ, Muller DA (2005) HfO 2 and Al 2 O 3 gate dielectrics on GaAs grown by atomic layer deposition. Appl Phys Lett 86(15):152904. https://doi.org/10.1063/1.1899745
Ritala M, Kaupo K, Antti R, Räisänen PI, Markku L, Timo S, Juhani K (2000) Atomic layer deposition of oxide thin films with metal alkoxides as oxygen sources. Science 288(5464):319–321. https://doi.org/10.1126/science.288.5464.319
Kim JY, George SM (2010) Tin monosulfide thin films grown by atomic layer deposition using tin 2,4-pentanedionate and hydrogen sulfide. J Phys Chem C 114(41):17597–17603. https://doi.org/10.1021/jp9120244
Yousfi E, Weinberger B, Donsanti F, Cowache P, Lincot D (2001) Atomic layer deposition of zinc oxide and indium sulfide layers for Cu(In,Ga)Se2 thin-film solar cells. Thin Solid Films 387(1-2):29–32. https://doi.org/10.1016/s0040-6090(00)01838-1
Zaera F (2008) The surface chemistry of thin film atomic layer deposition (ALD) processes for electronic device manufacturing. J Mater Chem 18(30):3521. https://doi.org/10.1039/b803832e
Pellin MJ, Stair PC, Xiong G, Elam JW, Birrell J, Curtiss L, Wang H-H (2005) Mesoporous catalytic membranes: synthetic control of pore size and wall composition. Catal Lett 102(3-4):127–130. https://doi.org/10.1007/s10562-005-5843-9
Elam JW, Routkevitch D, Mardilovich PP, George SM (2003) Conformal coating on ultrahigh-aspect-ratio nanopores of anodic alumina by atomic layer deposition. Chem Mater 15(18):3507–3517. https://doi.org/10.1021/cm0303080
Wei Z, Hai Z, Akbari MK, Qi D, Xing K, Zhao Q, Verpoort F, Hu J, Hyde L, Zhuiykov S (2018) Atomic layer deposition-developed two-dimensional α-MoO3 windows excellent hydrogen peroxide electrochemical sensing capabilities. Sensors Actuators B Chem 262:334–344. https://doi.org/10.1016/j.snb.2018.01.243
Bakke JR, Pickrahn KL, Brennan TP, Bent SF (2011) Nanoengineering and interfacial engineering of photovoltaics by atomic layer deposition. Nanoscale 3(9):3482. https://doi.org/10.1039/c1nr10349k
Van Delft JA, Garcia-Alonso D, Kessels WMM (2012) Atomic layer deposition for photovoltaics: applications and prospects for solar cell manufacturing. Semicond Sci Technol 27(7):074002. https://doi.org/10.1088/0268-1242/27/7/074002
Hatanpää T, Ritala M, Leskelä M (2013) Precursors as enablers of ALD technology: contributions from University of Helsinki. Coord Chem Rev 257(23-24):3297–3322. https://doi.org/10.1016/j.ccr.2013.07.002
Miikkulainen V, Leskelä M, Ritala M, Puurunen RL (2013) Crystallinity of inorganic films grown by atomic layer deposition: overview and general trends. J Appl Phys 113(2):021301. https://doi.org/10.1063/1.4757907
Warner EJ, Johnson F, Campbell SA, Gladfelter WL (2015) Atomic layer deposition of tin oxide and zinc tin oxide using tetraethyltin and ozone. J Vac Sci Technol A 33(2):021517. https://doi.org/10.1116/1.4907562
Heo J, Hock AS, Gordon RG (2010) Low temperature atomic layer deposition of tin oxide. Chem Mater 22(17):4964–4973. https://doi.org/10.1021/cm1011108
Mullings MN, Hägglund C, Bent SF (2013) Tin oxide atomic layer deposition from tetrakis(dimethylamino)tin and water. J Vac Sci Technol A 31(6):061503. https://doi.org/10.1116/1.4812717
Li AD, Liu WC (2010) Optical properties of ferroelectric nanocrystal/polymer composites. In Physical Properties and Applications of Polymer Nanocomposites (pp. 108-158). Woodhead Publishing. doi https://doi.org/10.1533/9780857090249.1.108
Sōmiya S, Roy R (2000) Hydrothermal synthesis of fine oxide powders. Bull Mater Sci 23(6):453–460. https://doi.org/10.1007/bf02903883
Du X, George SM (2008) Thickness dependence of sensor response for CO gas sensing by tin oxide films grown using atomic layer deposition. Sensors Actuators B Chem 135(1):152–160. https://doi.org/10.1016/j.snb.2008.08.015
Download references
Acknowledgements
The authors hereby devote acknowledgement to the University Research Commission (URC), the Global Excellence Stature (GES) as well as the National Research Foundation (NRF) South Africa for being supportive financially.
Author information
Authors and affiliations.
Department of Mechanical Engineering Science, Faculty of Engineering and the Built Environment, University of Johannesburg, Auckland, Johannesburg, 2006, South Africa
Emeka Charles Nwanna, Patrick Ehi Imoisili & Tien-Chien Jen
You can also search for this author in PubMed Google Scholar
Corresponding author
Correspondence to Tien-Chien Jen .
Additional information
Publisher’s note.
Springer Nature remains neutral with regard to jurisdictional claims in published maps and institutional affiliations.
Rights and permissions
Reprints and permissions
About this article
Nwanna, E.C., Imoisili, P.E. & Jen, TC. Fabrication and synthesis of SnO X thin films: a review. Int J Adv Manuf Technol 111 , 2809–2831 (2020). https://doi.org/10.1007/s00170-020-06223-8
Download citation
Received : 24 August 2020
Accepted : 05 October 2020
Published : 03 November 2020
Issue Date : December 2020
DOI : https://doi.org/10.1007/s00170-020-06223-8
Share this article
Anyone you share the following link with will be able to read this content:
Sorry, a shareable link is not currently available for this article.
Provided by the Springer Nature SharedIt content-sharing initiative
- Transparent conducting oxide
- Solar cells
- Find a journal
- Publish with us
- Track your research
Open Access is an initiative that aims to make scientific research freely available to all. To date our community has made over 100 million downloads. It’s based on principles of collaboration, unobstructed discovery, and, most importantly, scientific progression. As PhD students, we found it difficult to access the research we needed, so we decided to create a new Open Access publisher that levels the playing field for scientists across the world. How? By making research easy to access, and puts the academic needs of the researchers before the business interests of publishers.
We are a community of more than 103,000 authors and editors from 3,291 institutions spanning 160 countries, including Nobel Prize winners and some of the world’s most-cited researchers. Publishing on IntechOpen allows authors to earn citations and find new collaborators, meaning more people see your work not only from your own field of study, but from other related fields too.
Brief introduction to this section that descibes Open Access especially from an IntechOpen perspective
Want to get in touch? Contact our London head office or media team here
Our team is growing all the time, so we’re always on the lookout for smart people who want to help us reshape the world of scientific publishing.
Home > Books > Thin Films - Deposition Methods and Applications
Thin Films Processed by SILAR Method
Submitted: 11 June 2022 Reviewed: 11 July 2022 Published: 22 December 2022
DOI: 10.5772/intechopen.106476
Cite this chapter
There are two ways to cite this chapter:
From the Edited Volume
Thin Films - Deposition Methods and Applications
Edited by Dongfang Yang
To purchase hard copies of this book, please contact the representative in India: CBS Publishers & Distributors Pvt. Ltd. www.cbspd.com | [email protected]
Chapter metrics overview
361 Chapter Downloads
Impact of this chapter
Total Chapter Downloads on intechopen.com

Total Chapter Views on intechopen.com
Overall attention for this chapters
SILAR is one of the simplest techniques in terms of the better flexibility of the substrate choice, capability of large-area fabrication, deposition of stable and adherent film, low processing temperature for the film fabrication as well as reproducibility. This technique is very budget friendly since it does not require any sophisticated equipment. Moreover, various fabrication parameters such as solution concentration, precursors, the number of cycles during immersion, pH, annealing, doping, and growth temperature affect the rate of fabrication as well as the structural, optical, and electrical properties of the fabricated thin films led the technique unique to study in an extensive manner. A chapter regarding different aspects of semiconductors-based optoelectronics by SILAR has yet to be published. This chapter will concern the recent progress that has recently been made in different aspects of materials processed by the SILAR. It will describe the theory, mechanism, and factors affecting SILAR deposition as well as recent advancements in the field. Finally, conclusions and perspectives concerning the use of materials in optoelectronic devices will be represented.
- supercapacitors
- photovoltaics
- water splitting
Author Information
Md abdul majed patwary *.
- Department of Chemistry, Comilla University, Cumilla, Bangladesh
*Address all correspondence to: [email protected]
1. Introduction
The thin film is generally fabricated on a simply cleaned and rigid planar substrate having no dimensional limits. There is no restriction on the surface of the coating materials, so films can be fabricated on temperature-sensitive substrates like plastics. Even oxidation or corrosion of metal backing substrates can be used to deposit films by choosing suitable precursors.
The deposition rates and film thickness can be well controlled by monitoring reactant precursors, which are generally the desired cationic and anionic salts dissolved in solvents, while the anticipated stoichiometry can be attained through changing their type, concentration, or other involved dipping parameters.
By controlling the number of deposition cycles and concentration of species, the thicknesses of the thin films can be simply tuned over a wide range such as from nm scale to μm.
SILAR fabrications are very convenient and energy efficient as the technique is mostly controlled at room or low temperature. If required, the as-deposited coating materials can be annealed post-deposition to activate grain growth, crystallization, etc.
Besides, the fabricated thin films can be reformed to show preferential crystallographic orientation as well as grain assembly due to the controllability of ionic reactions performed at the substrate solution interface.
The SILAR method supports film development only on the surface of the substrate that is immersed into the solution, hence diminishing unnecessary consumption of the used reactants. If required, precursor solutions could also be reloaded and reprocessed.
Therefore, SILAR is a vastly multipurpose and influential process for the fabrication of numerous thin film materials having huge technological attention and, hence, unlocked a wide window in optoelectronic device applications.
2. Theory and process mechanism
SILAR is widely used, simple technique to fabricate high-quality thin films [ 3 , 15 ]. During deposition, successive ionic layer adsorption and reaction of the ions take place at the solid-solution interface of the substrate. Thus, the thin film of the compound, A p B y is deposited on to the substrate surface by dint of the adsorbed cations, xA y+ and anions, qB p− due to the following heterogeneous chemical reactions:
where x, y, p, q and y + , q + , x − , p − are the number and charges of the corresponding ions A (metal ions), P (cationic precursor), Q (anionic precursor), and B (anions) respectively [ 2 , 16 ]. Sometimes, the ligands L n are a necessity to complete the reaction [ 17 , 18 , 19 , 20 ]. The solution having the first element containing the final target material can be thought as the compound A x Q y fully dissociated in the chosen solvent such as in water (Reaction 3). Usually, A x Q y is a metal salt where A y + represents cations such as Zn 2+ , Cu 2+ , Mn 2+ , Cd 2+ , Bi 3+ , and B p − represents anions such as NO 3− , Cl − , SO 4 2− .
Hence, a basic SILAR cycle comprises four different steps, correlating alternate immersion of the substrate into cationic and anionic precursor solution followed by rinsing in every immersion cycle to eliminate loosely adhered particles as shown in Figure 1 and described below:

Representation of different steps during a SILAR cycle.
2.1 Adsorption
First step of the SILAR process is the formation of the Helmholtz double layer, which is due to the initial adsorption of cationic precursor, x A y + , on the surface of the substrate. This layer is generally composed of two charged layers, the positively charged inner layer and negatively charged outer layers. The positive (+ve) layer consists of the cations, x A y + , while the negative (−ve) layer, y Q x − , is the counter ions of the cations.
2.2 Rinsing I
In the second step, excessive adsorbed ions, x A y + and y Q x − , are rinsed away from the diffusion layer toward the bulk solution and a hypothetical monolayer is formed. This results in a saturated electrical double layer showing an ideal scenario of the process.
2.3 Reaction
In the reaction stage, the anions, qB p − , from anionic precursor solution are introduced into the system. A solid substance, A p B y , is formed on the interface due to the low stability of the material. This process employs the reaction of x A y + surface species with the anionic precursor, qB p − .
2.4 Rinsing II
In the final step of a SILAR cycle, the excess and unreacted species ( y Q x − , pP q+ ) and the reaction by product from the diffusion layer are removed leaving expected films.
A schematic presentation of a single cycle for the fabrication of Cu 2 SnS 3 film is shown in Figure 2 [ 21 ]. In the case of Cu 2 SnS 3 film fabrication, ion-by-ion type of deposition takes place through nucleation spots of the adsorbed surfaces [ 22 ]. Nucleation occurs due to the surface condensation of the ions and outcomes of, that is, an dense adherent thin film [ 23 ]. The substrate was firstly dipped into the cationic precursor containing mixed CuCl 2 and SnCl 2 solutions, where Cu 2+ and Sn 2+ species were available. Sn 2+ ion in solution is good reducing agents, and thus, Cu 2+ reduces to Cu + and Sn 2+ is oxidized to Sn 4+ in cationic solution as shown by the following reaction:

Schematic representation of Cu 2 SnS 3 thin film fabrication by SILAR technique [ 21 ].
The substrate was then rinsed off with DI H 2 O to eliminate the loosely bounded reactants. Then, it was dipped into an anionic precursor containing Na 2 S.xH 2 O solution, which gave sulfide ions (S 2− ) to react with the cations Cu + and Sn 4+ . Finally, the reaction occurred between the pre-adsorbed Cu + , Sn 4+ cations, and the S 2− anion to form a solid Cu 2 SnS 3 thin film as,
In the last step of the process, the substrate was again dipped into the DI H 2 O to remove the unwanted excessive particles to provide a uniform surface containing Cu 2 SnS 3 thin film.
3. SILAR-facilitated material deposition: A summary
The deposition of the series of chalcogenide mainly metal oxides, sulfides, selenides, and tellurides films has been always on numerous attentions in the advancement of the SILAR since its launch. Currently, SILAR has become a broadly functional technique in the deposition of a huge variety of semiconductor thin films. For the simplicity of discussion, we have summarized most of the metals still synthesized as metal compounds by SILAR in Table 1 .
List of the metals still grown by SILAR technique.
A list of materials deposited using SILAR technique with their growth conditions with the required raw materials for the growth is summarized in Table 2 . For the simplicity, the discussion is divided into four parts as of Table 1 , as specified below:
A list of materials deposited using SILAR technique with their growth conditions. (C, a: Cationic, anionic).
3.1 Metal oxides
An increasing number of oxide materials deposited by SILAR have demonstrated high chemical, thermal, and expected stability that is one of the reasons to increase the popularity of oxide synthesis by SILAR. However, the technique of oxide synthesis is somehow difficult compared to sulfides, selenides, and tellurides due to the unavailability of the anionic precursors, which is the direct source of O 2− to form oxides. For example, in case of the synthesis of most of the binary metal oxides, H 2 O, NaOH, and NH 4 OH are used as anionic precursors with a mild thermal treatment of around (70 ∼ 90) 0 C to activate the precipitation of hydroxides. On the other hand, the most common cationic precursors are mainly of metal thiosulfates, sulfates, chlorides, nitrates, etc., to provide metal ion adsorption on the substrate surface. Until today, Cu x O, ZnO, TiO 2 , and CdO are the most examined materials by SILAR. The investigation of Mn 3 O 4 , NiO, and Bi 2 O 3 is also increasing [ 31 , 32 , 33 , 34 ]. Recently, both nanostructured Fe 2 O 3 and Fe 3 O 4 have been fabricated applying sulfate and chloride salts using NaOH as the anionic precursor via SILAR [ 30 , 66 ]. But research on WO 3 [ 32 ], MgO [ 34 ], and SnO [ 67 ] fabrication is still rare. In case of ternary metal oxides, the SILAR deposition has been widely increased due to their ability to the additional modulate characteristics by controlling the composition of the materials. The synthesis of CST is discussed in the theory and mechanism section, which can be again done by two ways—a combined solution of both the deposited metal cations or, an alternating (one by one) fabrication of the two cations. A good technique to produce ternary metal oxides with excessive control on stoichiometry is to react one of the two metal ions by its own oxyanion. For example, Bi(NO 3 ) 3 and NH 4 VO 3 react to fabricate BiVO 4 [ 37 ], as ammonium vanadates are extremely soluble in water, while the anticipated metal vanadates are not. Consequently, they precipitate out of solution as the expected phase on the substrate surface. The other oxides such as Ag 3 VO 4 [ 36 ], BiVO 4 [ 37 , 68 ], Cu 2 V 2 O 7 [ 35 ], and Fe 2 V 4 O 13 [ 38 ] follow similar trends. Though bismuth oxyhalides, for instance, BiOI [ 69 , 70 ] is not a pure oxide but have been synthesized by the SILAR using cationic precursor of bismuth nitrate and anionic precursor of KI [ 71 ].
3.2 Metal sulfides
The characteristic easiness of the procedure and wide-ranging obtainability of the anionic precursors afford metal sulfides the most fabricated materials by employing SILAR technique. The easiest way of sulfide thin film deposition is to use metal salts as cationic precursors and H 2 O-soluble sodium sulfides or thiosulfates as anionic precursors. For example, NiS thin films could be fabricated using NiSO 4 (pH: 8) as cationic precursors and Na 2 S (pH: 10) as anionic precursors even at room temperature [ 47 ]. Generally, the solubility product constants of used sulfide materials in water are higher than 10 −20 , for instance, CuS: ≈10 −36 and CdS: ≈10 −27 , which is the key force of the deposition of the expected metal sulfides. Among the sulfides, CuS [ 72 ], ZnS [ 43 , 73 ], CdS [ 46 ], Ag 2 S [ 44 , 45 ], SnS [ 48 ], and PbS [ 49 ] have been broadly investigated mostly depending on the usage of chlorides and nitrates as the metal precursors and Na 2 S as anionic precursors for the S 2− source. Moreover, the investigation on NiS [ 47 ], Bi 2 S 3 [ 52 ] and MoS 2 [ 13 , 50 ], As 2 S 3 [ 13 , 51 ], MnS [ 53 ] are growing fast, while in the case of CoS [ 57 ], La 2 S 3 [ 58 ] studies are still infrequent. Moreover, core@shell-like SnS 2 @Co 3 S 4, ternary (NiCo 2 S 4 ) , and quaternary (Cu 2 BaSnS 4 ) films as well as nanocomposites of CdS and Bi 2 S 3 have been reported with their potential applications.
3.3 Metal selenides
In most of the cases, SILAR fabrication of the metal selenides has been directed through a solution of chloride, nitrate, or sulfate functioned as cationic precursor consisting of the anticipated metal, and a solution of Na 2 SeSO 3 worked as the anionic precursor consisting of the source of Se 2− to form selenides. For example, 0.2 M CdCl 2 . H 2 O (pH: 8) reacts with 0.1 M Na 2 SeSO 3 (pH: 11.3) to fabricate CdSe thin film [ 61 ]. Based on requirements, sometimes NaHSe, ethanolic NaBH 4 , or Na 2 Se can also be used as an anionic precursor to fabricate metal selenides. The SILAR deposition of Cu 3 Se 2 , Sb 2 Se 3 , Bi 2 Se 3 , and CdSe were studied and investigated mainly at room temperature avoiding thermal treatment during the sample growth, which was always maintained at (70–90) 0 C in the traditional cases.
3.4 Metal tellurides
The minimum studied materials among the chalcogen members via SILAR technique are metal tellurides because of the unavailability of the appropriate anionic precursors. Na 2 TeO 3 or ethanolic Te or TeO 2 with NaBH 4 is the mostly used anionic precursor performed as the source of Te 2− to form tellurides. For example, 0.1 M CuSO 4 . 5H 2 O (pH: 5) and 0.05 M Na 2 TeO 3 (pH: 9) react at an ambient temperature to synthesize Cu 2 Te film. Till now, CdTe, Cu 2 Te, La 2 Te 3 , Cu 7 Te 4 , and Bi 2 Te 3 thin films were fabricated and investigated via SILAR technique having potential uses in case of radiation detectors, photovoltaics, and thermo-electric devices [ 63 , 64 , 65 ]. More scientific research is expected to understand and control the characteristics of such fabricated films to build outstanding optoelectronic devices.
4. Recent advances by SILAR
The optoelectronic properties of SILAR grown thin films have been demonstrated in many more applications, for example, supercapacitors, photovoltaics, photoelectrochemical water splitting, gas sensors, and many more. The technique seems to be simpler and represents an efficient way to fabricate devices. Three potential applications such as supercapacitors, photovoltaics, and photoelectrochemical water splitting will be discussed in the following section.
4.1 Supercapacitors
The rapid progress in state-of-the-art tools has guided to a profound reliance on energy storage devices. Satellites, electric vehicles, laptops, cellphones, and sensors need some species of energy storage to function properly. The lead-acid battery was the first device, discovered around the 1800s, and most common storage energy till today. Supercapacitors, another promising energy storage device, well known as electrochemical capacitor or ultracapacitor creates a gap bridging role between conventional capacitors and batteries [ 74 ]. They can offer 1 ∼ 2 orders of higher magnitude of power density than rechargeable batteries as well as supply much more energy than traditional dielectric capacitors.
A supercapacitor works following two-charge storage mechanisms: (i) surface ion adsorption such as electric double-layer capacitance (EDLC) and (ii) redox reactions such as pseudo capacitance. Supercapacitors reveal an extraordinary set of features in comparison with batteries, for instance, high-power density, low maintenance cost, reliable cycling life, fast rates of charge or discharge, and safe operation as well as offer versatile powering solutions to many appeals ranging from portable consumer electronic appliance and electric automobiles to large-scale smart utility grids. Nevertheless, carbon-based EDLC supercapacitors show very low energy densities, which are limited through the finite electrical charge separation at the interface of electrolyte and electrode materials, as well as the approachability of surface area. Consequently, efforts to surge the energy densities of supercapacitors have involved the application of better pseudo-capacitance electrode supplies, equipped by conducting polymers and nanostructured metal oxides, bearing the low cost of high-power density as well as chemical stability, which have the significance of phase changes and faradaic reactions in it [ 74 ].
Several types of metal oxides, sulfides, and tellurides have been used in supercapacitor device fabrication so far, by utilizing the ever-fast-growing technique SILAR as summarized in Tables 3 and 4 . Initially the single metal oxides or sulfides such as CuO, NiO, NiMoO 4 , WO 3 , Bi 2 O 3 , Mn 3 O 4 , or MnS have been prepared by following SILAR technique and then tested for the supercapacitor behaviors to acquire the results of specific capacitance with their retention stability using cyclic voltammetry (CV) with the assistance of 3-electrode measurement system. Higher capacitance was attained at the lower scan rate and/or lower current density during such measurements and usually a relatively small quantity of electrochemical active material was developed atop of the working electrode. Moreover, the performance found using the 3-electrode system is higher than 2-electrode test cells, and the latter can be either a symmetric (S) or asymmetric (A) cell. Generally, in a symmetric cell both positive and negative electrodes are alike, whereas they are different active materials in an asymmetric cell.
Properties of various electrode materials deposited by SILAR for electrochemical capacitors by 3-electrode system.
Supercapacitors performance of SILAR grown films measured by 2-electrode system.
Not only binary, but also ternary or even doped metal oxides, sulfides or tellurides were synthesized via SILAR for supercapacitor device application. For example, ZnCo 2 O 4 and ZnFe 2 O 4 were synthesized via SILAR technique from binary cationic solutions in the presence of Zn and Co (or Fe) precursors and demonstrate high energy density of 9.67 and 28 Wh kg −1 as well as power density of 1451 and 7970 W kg −1 , respectively. La 2 S 3 and La 2 Te 3 with mesoporous pine-leaf structure prepared with SILAR showed 35 and 60 WhKg −1 energy density and power density of 1260 and 7220 WKg −1 , respectively. A flexible La 2 Te 3 │LiClO 4 -PVA│La 2 Te 3 supercapacitor cell was further fabricated and is represented as in Figure 3 .

Schematic diagrams of La 2 Te 3 │LiClO 4 -PVA│La 2 Te 3 supercapacitor device [ 65 ].
Hybrid supercapacitors, EDLC and pseudo-capacitance, build of charge storage mechanisms reduce the superior features of the device. On the other hand, among the other EDLC electrode materials multiwalled carbon nanotubes (MWCNTs) fascinated major interest due to their favorable features such as high surface area and mesoporous network, good mechanical strength and flexibility, excellent electrical conductivity, and chemical stability. The facile synthesis of composites of metal oxides with carbon materials was facilitated by SILAR as well. For instance, the fabrication of NiO/MWCNTs nanohybrid thin films via SILAR and the specific capacitance was as high as 1727 Fg −1 and current density 5 mAcm −2 with 91% retention ability after 2000 cycles as demonstrated in the Figure 4 [ 84 ]. Moreover, an analogous synthesis style was employed to NiCoO x /Carbon-black hybrid thin films accomplishing coatings with a high specific capacitance of 1811 Fg −1 at 0.5 mAcm −2 [ 85 ].

Assembly of highly flexible symmetric NiO/MWCNTs-NiO/MWCNTs nanohybrid device: (a) image of flexible NiO/MWCNTs thin film deposited on the stainless steel, (b) NiO/MWCNTs nanohybrid thin film electrodes with closed ends (2 × 3 cm 2 area), (c) coating of the electrode by PVA/LiClO 4 gel electrolyte, (d) flexible supercapacitor built under the ∼1 ton pressure through sandwiching the two-gel electrolyte coated electrodes, (e) two flexible supercapacitors in series can successfully light a LED [ 84 ].
Therefore, SILAR is a unique as well as multipurpose technique to fabricate thin films for supercapacitor device application with superior power and energy densities in comparison with other available and more conventional deposition techniques, justifying the quality of the SILAR growth thin films.
4.2. Solar cells
Photovoltaic (PV) is a simple device, which promotes the direct conversion of light radiation into electrical energy by following the photovoltaic effect [ 91 ]. The discovery of such a device for the conversion of sunlight radiation directly into electricity was first carried out during the late 1800s. C. Fritts first demonstrated the solid-state PV by fabricating a thin layer of Au on Se semiconductor material [ 92 ]. At American Telephone and Telegraph Bell Laboratory the modern PV was discovered by Ohl in 1946 [ 93 ] but demonstrated by Chapin, Fuller and Pearson in 1954 [ 94 ]. The cell was fabricated by single-crystal Si wafer having an efficiency of ∼5%.
At present for feasible use, extensive research is going for efficiency enhancement of solar cells, as the efficiency of solar cells is one of the very vibrant parameters to promote this technology. Over the years, the efficiency of single crystal-Si solar cells has shown a sound development. In 1950s, it was only 15% and nowadays it is improved to around 26.7% [ 95 ]. The commercial efficiency of Si solar cell is approaching in between 12% and 15%, while the theoretical Shockley-Queisser (SQ) limit energy conversion efficiency is of around 28% [ 96 ]. The PV cell and module market was mostly occupied on first-generation Si-based cells until 2004, for example, sc- and poly-Si cells, which covered about 85% of the overall international PV modules market. In the meantime, thin film cells or second-generation PV have exhibited great advantages, for instance, the ease of large area fabrication and usage of minimum materials, though their market share was much smaller in comparison with the Si-cells [ 97 ]. After 2005, the developments were spurred by the sharp increase in the country’s implementation of solar energy due to the rapid advancement of the PV production industry in China. The price of PVs is generally supplemented by the strict requirement for fabricating high-purity materials such as GaAs and Si, or the rare-earth elements such as CIGS. The element, In, is rare and can be certainly exhausted, which might affect the prospect of such PVs. Later, CdTe thin-film PVs have increased longing in the market of South-Eastern countries. But Cd has a serious environmental distress, which is due to its high toxicity [ 98 , 99 ]. For example, chronic Cd exposure breeds an extensive acute and chronic effects in humans [ 100 , 101 ]. Moreover, Cd is a rare earth element and it will also generate a higher cost within the demand in future. Further, a third generation of devices has newly developed in addition to the thin-film solar cells, based on fresh organic materials such as dye-sensitized solar cells (DSSC) [ 102 ], quantum dot solar cells (QDSC), perovskites, bulk heterojunctions, having innovative device architectures with the usage of multiple exciton generation, upconverting layers, and others. Though, organic materials-built PVs have small life spans as the nature of the materials, for example, thermal stability [ 103 ] or concerns of electrolyte-based variability [ 104 ]. Inherently, a mostly striking new field of PV devices using metal oxide (MO) semiconductors has performed [ 105 ]. Atop of the MO thin films, favorable next-generation PV cells such as exciting thin absorber cells [ 106 ], DSSC [ 107 ], and QDSC [ 46 , 108 ] are built as they are promising applicants for being stable, eco-friendly, and ultra-low-cost PV materials.
SILAR accounts itself directly into the third generation, by affording ultra-thin, compositionally fabricated by layers of several semiconductors that could be subjugated in a diversity of device architectures. Besides Si, most of the absorbers in PVs are conventionally II–VI, III–V semiconductors, or organic polymers, or small molecules, or perovskites. Still, the number of metal oxides is not adequate, which can be effectively used as absorber layers. Consequently, research into SILAR-grown light absorber layers for PV device applications has been aimed mainly on selective transition metal oxides, sulfides, and selenides. These materials have drawn incredible interest in technological and scientific research due to their unique optical, electrical, and mechanical properties in the past few decades [ 109 , 110 , 111 ]. Nevertheless, there are some examples of SILAR grown metal oxides, sulfides, and selenides applied in different types of solar cells such as thin film, DSSC, perovskite, and QDSC as summarized in Table 5 . The layers were used not only to achieve high efficiency, but also served diverse roles inside the PVs such as light absorber, selective charge transport (electrons or holes), and passivation.
SILAR growth PV cells demonstrating with the cell properties.
N.B. N3: ethanolic 0.3 mM cis-Bis (isothiocyanato) bis (2,2′-bipyridyl-4,4′-dicarboxylato ruthenium (II).
In many cases, the core light-absorbing layers, within the solar cells, fabricated via SILAR have been investigated. For example, in ITO/CdS/PbS/C heterojunction solar cell, n-type layer, CdS thin films were deposited by CBD on transparent conductive oxide (ITO) substrates, whereas PbS film by SILAR using different deposition cycles, 15, 20, 30, 40 and 60 to obtain different thicknesses, showed that 40 cycles PbS film has a greater photovoltaic conversion efficiency [ 115 ]. In another study, p -type CuO was utilized as photo-absorber in the p-CuO/n-Si heterojunction cell [ 123 ], where a vibrant role of the SILAR deposition was observed in the overall device performance, depending mainly on the concentration of the copper precursor solution. In an all-oxide solar cell, NiO/Cu 2 O or CuO/ZnO/SnO 2 , both Cu 2 O and CuO fabricated were examined as light absorber fabricated by SILAR and the hole transporting layer ( p -type NiO), buffer layer (ZnO) as well as n -type SnO 2 were deposited by sol-gel method [ 124 ]. The cell having Cu 2 O showed better performance than CuO, which is due to the reduced conductivity, mobility, and carrier concentration of CuO. However, the study showed an overall efficiency over 1%. In a different study, heterojunction solar cells have been fabricated between layers of p-type CuS and n-type Ag 2 S deposited via SILAR method and Sn 2+ and Al 3+ heterovalent dopants are introduced in Ag 2 S so that Fermi energy of the semiconductor can be modified to alter the band diagram of pn junctions. The Sn 2+ -doped Ag 2 S resulted in better solar cell parameters with an efficiency of 2.85% as compared to that based on Al 3+ -doped Ag 2 S, which consists of many defect states due to mismatch in ionic radii of the cation and the dopant ions [ 44 ].
Further, metal oxides were worked in charge transportation in between different layers in solar cells, and both electron transport layer (ETL) and hole transport layer (HTL) can enhance the performance of PVs. Since the early 1990s, TiO 2 is one of the key materials used as ETL owing to its wide popularity in DSSC [ 120 ]. In a recent study, TiO 2 nanocrystalline film was directly deposited using SILAR at 90°C for perovskite solar cell applications and used as an ETL [ 114 ]. Due to the fast charge transport, kinetics and slow charge recombination process of the TiO 2 ETL synthesized from the solutions of TiCl 4 and hot K 2 S 2 O 8 , with subsequent annealing at 450°C, advances the efficiency of the cell to around 10%. Further, a couple of studies showed the deposition of TiO 2 layers from solutions of TiCl 3 and NaOH [ 27 , 125 ] followed by annealing at 400°C, as ETLs in DSSC with the modest efficiency of just over 1%. Other SILAR-fabricated layers used as ETLs in PVs consist of ZnO and ZrO 2 as interfacial layer attached to porous TiO 2 , both demonstrated performance in DSSC [ 102 , 126 ].
In this study, Cu 2 O thin films were introduced as a HTL in a planar perovskite solar cell and successfully enhanced the efficiency of the cell to around 8.23%, as shown in Figure 5(a-c) . The Cu 2 O films were deposited via SILAR by followed the complexation reaction of copper and ammonia with H 2 O 2 [ 112 ]. The methylammonium lead triiodide (MAPbI 3 ) perovskite layer is sandwiched between a p -type Cu 2 O HTL layer and another n -type PCBM (phenyl-C61-butyric acid methyl ester) ETL layer, respectively. The Cu 2 O films demonstrated suitable band structure after annealing at 170°C and boosted device performances better than conventional sol-gel-deposited NiO and Cu-doped NiO hole transport layers, confirming the quality of the SILAR-Cu 2 O.

(a) Cell structure, (b) schematic energy level diagram; the dashed line represents the Fermi energy after contact (c) current-voltage characteristics of under dark and a white light illumination condition of Cu 2 O/MAPbI 3 /PCBM heterojunction [ 112 ].
In a report of 2009, Lee et al. showed a novel technique for preparing selenide (Se 2− ) by the SILAR process in pursuit of efficient QD-sensitized solar cells atop of mesoporous TiO 2 photoanodes. After several optimization of the QD-sensitized TiO 2 films via regenerative photoelectrochemical cells in presence of a cobalt redox couple [Co(o-phen) 3 2+/3+ ], with a final layer of CdTe, the overall efficiencies of the was recorded around 4.2% at 100 Wm −2 [ 127 ]. To find the answer to a question, “How does a SILAR CdSe film grow?” Becker et al. tuned the deposition steps to suppress interfacial charge recombination in FTO/TiO 2 /CdSe/Na 2 S: S/CoS 2 cell by showing an efficiency of 3.53% [ 117 ]. Recently, in another report, SILAR- and CBD-grown CdSe-sensitized TiO 2 solar cells were examined concentrating on the influences of two commonly used QD deposition techniques [ 119 ], and atop of pre-accumulated CdS seed layers, a successful CdSe deposition was performed. The PEC of both the cells has been recorded as 4.85%, for CBD grown CdS/CdSe cell, whereas for SILAR grown cell the value was 3.89%. One research group enhanced the PCE of CdS/CdSe/S 2− -S/RGO/Cu 2 S cell to 5.4% by employing Mn 2+ doping of CdS via SILAR method [ 118 ], whereas another group reported on a PbS: Hg QD-sensitized solar cell by Hg 2+ doping into PbS employing similar deposition technique and showed an unprecedentedly high J SC of 30 mA/cm 2 with the PEC of 5.6% [ 120 ]. More studies are ongoing with great efforts to find new alternative, clean, and environment-friendly energy resources due to the increasing demands.
4.3. Photoelectrochemical water splitting
Hydrogen energy is a key issue to cope with the present global energy crisis and environmental complication exploiting clean and inexhaustible energy [ 128 ]. Photoelectrochemical (PEC) water splitting is a promising technique to create hydrogen fuel by utilizing solar energy. Within the nonstop efforts in developing efficient photoelectrodes, the major challenge researchers presently face is to explore cost effective, nontoxic, and earth-abundant photoelectrodes with high efficiency [ 129 ]. In a recent study, Ag/Ag 2 WO 4 was fabricated on ZnO nanorods using 0.05 M AgNO 3 and 0.05 M Na 2 WO 4 as the cationic and anionic precursors, respectively, by following SILAR technique and the composite material demonstrated outstanding performances in PEC water splitting with 3 mAcm −2 at 1.23 V versus RHE in the presence of 0.1 M Na 2 SO 4 electrolyte. Based on these results, a brief possible updated mechanism of the PEC activity was demonstrated by Adam et al. for the better understanding of the technique with Figure 6 [ 130 ]. The development of PEC activity of the semiconductors was principally attributed to electrons and hole transfer at the interfaces of the photoelectrodes. The band edge potentials of the Ag/Ag 2 WO 4 and the ZnO materials showed a significant role in the efficiency of growth and separation technique of the electron (e − ) and hole (h + ) pairs. The energy of valence band (E VB ) of ZnO and Ag 2 WO 4 is calculated as +2.86 and + 3.03 eV, whereas energy of conduction band (E CB ) of them is projected as −0.34 and − 0.07 eV, respectively [ 131 ].

(a) and (b) FM-SEM images of the ZnO NRs and the ZnO/Ag/Ag 2 WO 4 heterostructure. (c) Curves of the ZnO NRs, and the ZnO/Ag/Ag 2 WO 4 photo-electrodes under light and dark conditions using linear sweep voltammetry. Schematic diagram presenting the energy band structure and probable electron-hole separation as well as transportation in ZnO/Ag/Ag 2 WO 4 heterostructure with the SPR effect [ 130 ].
In sunlight, both the semiconductors absorb light and the electrons in the VB become excited up to a higher potential of −0.34 and − 0.07 eV for the ZnO and Ag 2 WO 4 , respectively. Consequently, due to high photon energy, within the semiconductor, the effective charge transfer process proceeds. Ag 0 nanoparticles (NPs) cause active separation of h + or e − pairs upon the absorption of light owing to the surface plasmon resonance (SPR) effect. Electrons from the Ag NPs are transported to the CB of the Ag 2 WO 4 and the ZnO, while holes persist in the Ag NPs. In the meantime, to occupy the vacant holes created by the plasmonic absorption, the photogenerated electrons in the CB of ZnO will be transported to the Ag NPs [ 132 ]. The photogenerated charge carriers can be proficiently separated to enhance the PEC performance by following this mechanism. Further, the photogenerated electrons will eventually reach at the Pt electrode (counter) and contribute to H 2 generation. Also, the photogenerated holes in the VB of Ag 2 WO 4 and ZnO will contribute on O 2 production via H 2 O oxidation. Hence, these outcomes validate the modification via Ag/Ag 2 WO 4 , which is an active technique to attain a high PEC activity by means of ZnO NRs arrays.
Like the above example, many studies based on SILAR had been devoted toward exploring the potential of semiconductor thin films as photoelectrodes for water splitting as shown in Table 6 with their potential applications. In terms of low-cost, simplicity, and theoretically high solar to H 2 efficiency, PEC water splitting is much more favorable than solar photobiological, photochemical, and thermochemical generation of hydrogen [ 146 ]. The most investigated semiconductor materials include BiVO 4 , Fe 2 O 3 , CuCoO 2 , WO 3 , and TiO 2 [ 147 ]. Other semiconductor materials such as Cu 2 O [ 24 , 148 , 149 ], ZnO [ 150 , 151 ], TiO 2 [ 152 , 153 ], and CdO [ 28 ] were also produced using SILAR method but the PEC performances are quite low under visible light due to their wide bandgap.
SILAR growth films demonstrating the properties of photoelectrochemical water splitting at 1.23 V vs. RHE.
5. Factors affecting SILAR deposition
A lot of research work has been done on the deposition and optimization of the SILAR thin films for optoelectronic device applications. Solution concentration, composition of precursors, the number of SILAR cycles, pH, annealing, and doping will absolutely affect the quality and quantity of thin films, which directly influence the cell performance. The effect of different parameters used in SILAR deposition on the performance of thin films is reviewed based on the contemporary research work.
5.1 Solution concentration
Solution concentration of the used precursor is one of the key factors in governing the properties as well as the performances of SILAR grown thin films. From a general viewpoint, depositing through a more concentrated solution results with bigger grain size and higher surface roughness during deposition. Consequently, thinner, smoother, and probably pinhole-free deposition can be attained using multiple SILAR cycles with a lower concentrated precursor solution.
With the increase of molar concentration (0.03, 0.05, and 0.1 M) of the cationic solution prepared by Cd(CH 3 COO) 2 and H 2 O 2 , the surface morphology of the SILAR-deposited nanostructured CdO thin films was improved toward the crack free and homogeneous nature [ 154 ]. On the other hand, the structural change such as nanorods, nanoflowers, and nanoflakes morphologies was observed by altering only the concentration of anionic precursors NaOH (high, 0.05 M; moderate, 0.01 M; and low, 0.001 M) with fixed Zn precursor concentration (0.005 M) [ 155 ]. A comparative study of CdS films deposited by SILAR and CBD techniques revealed that the S/Cd ratio in the sample increases (0.83 to 1.04) for SILAR deposited films with the molar concentration of sulfur (1:1, 3:1, 5:1 and 7:1) in the starting solution increases, while it was almost constant (∼0.80) for CBD films [ 156 ]. During the investigation of the effect of the molar concentration of pyrrole monomer on the electrochemical behavior of highly pristine poly-pyrrole flexible electrodes, it was shown that among 0.025 M, 0.05 M, and 0.1 M pyrrole, the 0.1 M pyrrole exhibited excellent performance with specific capacitance as high as 899.14 Fg −1 at 5 mVs −1 in 0.2 M Na 2 SO 4 showing retention stability of 61.5% even after 2000 cycles [ 107 ]. The SILAR synthesis, in such case, was performed on the stainless steel strips, which were firstly immersed in pyrrole precursor, followed by 30% H 2 O 2 for 10 s each [ 107 ]. Further, studies on the use of the optimum precursor concentration of different Mn dopant (0.04 M, 0.075 M and 0.1 M) in CdS QDSSCs reveal that 0.075 M Mn-doped CdS can strongly enhance the incident photon to charge carrier efficiency (IPCE), due to the improved light harvesting, electron injection as well as charge collection efficiencies. As a result, the PCE of SILAR-grown Mn/CdS QDSC is up to 3.29%, which is much higher than that of QDSC without doping (2.01%) as well as other used concentration of Mn dopant under standard simulated AM 1.5 G, 100 mW cm −2 [ 157 ].
5.2 Effect of precursors
ZnO is one of the most investigated materials performed by SILAR technique. During fabrication, among the other properties precursor selection is one of the key requirements. In this study, the role of the precursor materials such as Zn(CH₃COO)₂, ZnSO₄, and ZnCl 2 on the properties of SILAR-deposited ZnO films were examined and the outcomes showed that the films fabricated by utilizing Zn(CH₃COO)₂ and ZnSO₄ precursors exhibited better optical properties than ZnCl 2 . Besides, the crystallite sizes of all the fabricated samples were increased upon annealing [ 158 ]. On the other hand, the effect of four different precursors of Zn(NO 3 ) 2 , Zn(CH 3 COO) 2 , ZnSO 4 , and ZnCl 2 on structural, morphological, electrical and optical properties of AZO thin films using SILAR method was examined. After varying the different precursors, the significant effects on film crystallization, surface morphology, optical nature, and electrical resistivity of the deposited films were studied, in which chloride precursor demonstrated the best performance [ 159 ].
Sfaelou and co-workers studied the effect of the nature of three cadmium precursors such as Cd(NO 3 ) 2 , CdSO 4 , and Cd(Ac) 2 on the effectiveness of CdS SILAR deposition and measures the performance of sensitized solar cells and photo fuels. The CdS reflection spectra, load, and the size of CdS nanoparticles varied a lot from one precursor to the other as shown in Figure 7(a-e) . The highest load and the largest nanoparticles were obtained in the case of Cd(Ac) 2 , and the smallest in the case of Cd(NO 3 ) 2 . And acetate-derived photoanodes provide more effective outcomes in the case of QDSSCs, while nitrate-derived precursors were more effective in the case of photo fuel cells as in Figure 7(f, g) [ 11 ]. In a similar but detailed study, Zhou and co-worker showed almost similar results showing a better performance of Cd(Ac) 2 over Cd(NO 3 ) 2 during the study of another CdS QDSCs as shown in Figure 7(h) [ 119 ]. Another recent investigation on the effect of different precursors such as Mn(CH₃CO₂)₂, MnCl 2 , and MnSO 4 on electrochemical properties of Mn 3 O 4 thin films prepared by SILAR method using 1 M Na 2 SO 4 aqueous electrolyte exhibited the specific capacitance of 222, 375, and 248 Fg −1 , respectively, at 5 mVs −1 scan rate. Hence, the MnCl 2 -derived Mn 3 O 4 electrode showed a good electrochemical with maximum energy density of 17 Whkg −1 and power density of 999 Wkg −1 at 0.5 mAcm −2 current density showing retention stability of 94% after 4500 CV cycles [ 160 ]. Besides, a study of SILAR-deposited SnO 2 films showed improvement of the crystallite with solution molarity performed by using different precursor concentration of both cations and anions [ 161 ].

TEM and HRTEM photographs of (a) pure titania and titania loaded with CdS deposited by using the three precursors: (b) Cd(NO 3 ) 2 , (c) CdSO 4 , and (d) Cd(Ac) 2 . (e) Reflection spectra using Cd(NO 3 ) 2 , CdSO 4 , Cd(Ac) 2 , and titania film without CdS. J − V curves recorded with a (f) QDSSC and (g) photo fuel cell employing 1 cm 2 active photoanode and 2.25 cm 2 active cathode electrode [ 11 ]. (h) J − V characteristics of CdS QDSCs fabricated by using acetate and nitrate precursors and measured under the illumination of one sun (AM 1.5, 100 mW/cm 2 ) [ 119 ].
5.3 Number of deposition cycles
SILAR technique involves the successive immersion of the substrate in anionic and cationic precursors following the substrate rinsing procedures in between. The deposition rate and the thickness of the required films can be simply controlled over a wide range by varying the deposition cycle and there are no boundaries on the substrate material, dimensions, or surface profile to be used, which in turn influence the properties such as crystallite size, surface morphology, and possibly light absorption. Nevertheless, overloading may consequence in delamination and fragmentation of the films owing to undesirable mechanical stress. The number of cycles optimization is therefore requisite to all SILAR system for the anticipated utilization.
Recently, lily flower-like ZnO structures were demonstrated by a group of researchers deposited by SILAR method [ 162 ]. In the study, lily flower-like morphologies were obtained when the deposition cycle number increases from 1 to 10 as shown in Figure 8(a-d) . Another group, while studying the growth of porous Fe 2 V 4 O 13 films for photoelectrochemical water oxidation, the deposition cycle had directly altered the current density as shown in Figure 8(e) . The highest photocurrent was achieved at the potential of 1.23 V vs. RHE for a Fe 2 V 4 O 13 film attained through 20 deposition cycles, which was chosen to improve the performance of the material further [ 38 ]. Further, Das and coauthors studied the influence of dipping cycle on SILAR-synthesized NiO thin film and observed that 40 cycle dipping NiO electrode provides highest specific energy of 64.38 WhKg −1 with the highest specific power 2305 WKg −1 , by retaining fast electron transfer as well as admission of electrolyte ions much easily due to porous nanostructure of fabricated electrode [ 78 ]. Moreover, other efforts including the effect of immersion cycles on structural, morphology, and optoelectronic properties such as Ag 2 S [ 163 ], CdO [ 164 ], ZnS [ 165 ] thin films were studied extensively, which make them desirable for optical coating as well as other opto-electronic applications.

(a-d) FE-SEM photographs of ZnO lily flower-like structures deposited by varying number of deposition cycles via SILAR method [ 162 ]. (e) Chopped LSVs of Fe 2 V 4 O 13 films with different number of cycles annealed at 500°C for 1 h in a buffer solution of pH 9.2 [ 38 ].
5.4 Impact of pH
By altering the pH of both cationic and anionic precursors, it is possible to tune the bandgap of thin films over a wide range for optoelectronic device applications. Preetha and co-workers investigated the effect of cationic precursor pH on optical as well as transport properties of SILAR-fabricated nanocrystalline PbS thin films. They successfully showed that the pH of the cationic precursor and in turn the size of the crystallites affect the optical and electrical properties of PbS thin films [ 166 ]. Besides, Sakthivelu and coauthors demonstrated a similar effect on ZnO thin films that the grain size of ZnO increased with the increase in pH of the precursor solution as represented in the SEM micrograms in Figure 9(a-e) . The film deposited at pH =8.5 shows aggregated and non-uniform grains, while flower-like appearance appeared at pH =9. Later, at pH =9.5, bigger grains with hexagonal nanorods structure appeared and finally, at pH =10, the size of the nanorods increased further with the well elongated nanorods sticking with each other. They also showed a decrease of bandgap from 3.29 to 3.09 eV with the increase of pH [ 167 ].

SEM micrograms of ZnO thin films prepared at (a) pH = 8.5, (b) 9.0, (c) 9.5, (d) 10.0, and (e) 10.5 [ 167 ].
Moreover, both acidic and basic mediums can be used based on the requirement to deposit films as listed on Table 7 . Farhad and co-author worked on Cu 2 O thin film under pH range from ∼2 to 8 [ 169 , 170 ], while Gençyılmaz [ 171 ] and Visalakshi et al. [ 172 ] on CuO thin film under pH range from ∼9 to 12 by showing promising electrical and optical properties. In another research, CdO was deposited using Cd(CH 3 COO) 2 as a cationic precursor and thickness as well as bandgap tuning was effectively observed in the pH range of 11.3 to 12.5, in addition to NH 3 solution [ 173 ]. However, not only a wide range of study to understand the influence of pH on the thin films fabricated by SILAR, but also more device fabrications were performed under diverse pH conditions with excellent performance, as described in the application section.
Properties of thin films deposited by varying solution pH via SILAR method [ 168 ].
OP = Optimized precursor (2 M NaOH), NOP = Non optimized precursor, SA = Sulfuric acid and AA = Acetic acid.
5.5 Annealing
SILAR is currently in demand to maintain the high quality of films with high growth rate. Despite extensive efforts, the adsorption of complex agents from precursors, drop of rinsing time, and slow production rate for almost all kinds of films has still been a main disadvantage, which restricts its usage in semiconductor industry. New deposited films may have defects, for instance, oxide vacancies and hydroxide phase since the presence of hydroxide phase is unavoidable owing to an aqueous alkaline medium for fabrication [ 174 ]. However, annealing of films can minimize such defects and eliminates the hydroxide phase along with the recrystallization. As high-temperature annealing mostly induces a rise in crystallite size, and possibly alters in morphology, which is steady with thermally induced grain growth. Still, the bandgap does not allow a steady trend with annealing due to numerous factors coming into ground outlining the absorption onset: size of particles, existence of defects, stoichiometry as well as existence of oxygen vacancies, etc. [ 175 ].
Putri and co-authors studied annealing temperature effect on the photovoltaic performance of BiOI-based materials and showed that at 300°C temperature, the role of the device which consisted of Bi 7 O 9 I 3 attained three times higher efficiency than the annealed parent BiOI at 100°C. Hence, the structural tuning due to the addition of oxygen via annealing to BiOI structure had an influence on the photoelectrochemical cell [ 69 ]. Besides, Ashith and co-worker studied the effect of post-deposition annealing on the properties of ZnO films and demonstrated that the crystallite size of the films increased significantly after annealing. The annealed films further showed very high absorption in the UV region with marginal modification in bandgap. Both the crystallite size and optical absorbance were observed to rise proportionately with the annealing temperature [ 176 ]. In a separate study of annealing and light effect on structural, optical and electrical properties of CuS, and Cu 0.6 Zn 0.4 S thin films grown by the SILAR demonstrated that the current increase with increasing light intensity and increasing rate in illuminated 500 Wcm −2 films were greater than the others that have annealed at 400°C [ 43 ]. Further studies are reported showing grain size increase after annealing or bandgap tuning are listed in the Table 8 , including Cu 2 O, ZnO, CuO, CdO, MgO, NiO etc.
A list of thin films grown via SILAR and annealed further for better film quality.
5.6 Impact of doping
In order to have a maximum number of carriers to take part in the functioning, a material with low activation energy is necessary so that electrons can easily jump from valence band to conduction band and doping is one of the best options in such structure tuning [ 181 , 182 ]. In many cases, the incorporation of cation doping is an effective way to improve the electrical conductivity [ 183 , 184 ]. By decreasing the bandgap, electron transfer between the valence and conduction bands will increase, and thus in case of energy storage device, electrode capacitance will increase [ 185 ]. For example, Y-doping in Sr.(OH) 2 improves both electronic conductivity as well as electrochemical performance of the electrode for energy storage device [ 89 ].
Again, electrochemical performance of In 3+ -doped WO 3 /[Cu 7 Te 4 /Bi 2 Te 3 ] electrodes for similar applications enhanced the capacitance to a great extent [ 90 ]. The study showed the specific capacity of undoped WO 3 was around 64 mAhg −1 whereas it was increased to 90.2 mAhg −1 for the same scan rate of 10 mVs −1 for In 3+ -doped films, with the high-power density of 1.7 kWkg −1 at the highest energy density of 18.85 Whkg −1 . Inside the WO 3 lattice, the doped In 3+ cation diffused and was connected in the insertion-removal exchange method of electrons, with further electrochemical S 2− insertion or extraction striking at the Cu 7 Te 4 /Bi 2 Te 3 and polysulfide electrolyte surfaces.
Besides, Zhu and coauthors prepared Cu-doped CdS on QDSCs and investigated the effect of Cu doping on several cells based on the doping concentration. When the doping ratio of Cu decreased successively from 1:10, all the parameters such as J sc , V oc , and PEC increased and reached at the maximum value when the ratio became 1: 500 [ 186 ]. In a separate report of Mn-doped-CdS/CdSe deposited on mesoscopic TiO 2 film as photoanode using Cu2S/graphene oxide composite electrode, in the presence of sulfide/polysulfide electrolyte provide PEC of 5.4%, higher than undoped sample [ 118 ], whereas Hg 2+ -doped PbS QDSC having unprecedentedly high photocurrent delivered PEC of 5.6% at one sun illumination over the undoped PbS QD cell [ 120 ]. Moreover, Abel and co-workers developed an improved photoelectrochemical water splitting device via SILAR-fabricated Ti-doped α-Fe 2 O 3 thin films. Ti, acting as water oxidation intermediates, enhanced interfacial hole transfer efficiency from less than 3–80% by increasing the concentration of surface-trapped holes, which is then triggered by FeOOH to amplify hole transfer efficiency to ∼100%. Both Ti doping and FeOOH overlayer resulted in photocurrents of 0.85 mAcm −2 at 1.23 V vs. RHE [ 138 ]. However, a lot of work has been done by several authors to get better film quality through doping. A list of such initiatives of the doped films with the starting materials of growth and other properties is shown in Table 9 .
A list of the doped films with some of their properties, fabricated by using SILAR techniques.
6. Conclusion
This chapter represented detailed discussions on the methods and techniques of the fabrication process of thin films by utilizing SILAR for the optoelectronic device applications. Among the diverse fabrication techniques both physically and chemically, SILAR is the simplest to fabricate thin films having remarkable quality. It is widely fit for the fabrication of thin films of metal chalcogenides, hydroxides, peroxides, as well as complex and composite nanostructures with innovative functionalities. The role of experimental conditions on the structural, optical, and electrical properties of the thin films as well as device performances is reviewed in this chapter mainly for the advanced utilization of both the generation and storage of energy such as solar cells, photoelectrochemical water splitting, supercapacitors, and so on. The technological advancement of a fabrication technique is deeply reliant on the opportunity of controlling the experimental factors involved. In this chapter, a brief advantage of SILAR technique is highlighted, including flexibility of the film growth, thickness control, composition control, and low temperature management, along with a broad range of applications. From this point of view, a deep knowledge of the connections between processing, structure, specific characteristics, and performances is the foundation for accurate and rational engineering of such optoelectronic devices. Moreover, a comprehensive profile of recent status is required to focus on further prospects. This work will therefore deliver a strong contribution to move ahead with future research goals on SILAR technique, by utilizing low-cost deposition of high-quality thin films and associated optoelectronic devices.
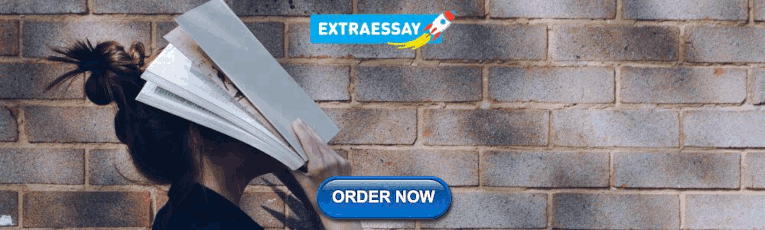
Acknowledgments
The author thanks the Department of Chemistry, Comilla University and UGC, Bangladesh, for supporting this work. Also, thanks to Professor Dr. Jamal Uddin, Center for Nanotechnology, Department of Natural Sciences, Coppin State University, Baltimore, MD, USA, for his insightful discussion and support.
Conflict of interest
The authors declare no conflict of interest.
- 1. Nicolau Y. Solution deposition of thin solid compound films by a successive ionic-layer adsorption and reaction process. Applied Surface Science. 1985; 22 :1061
- 2. Ristov M, Sinadinovski G, Grozdanov I. Chemical deposition of Cu2O thin films. Thin Solid Films. 1985; 123 :63
- 3. Pathan H, Lokhande C. Deposition of metal chalcogenide thin films by successive ionic layer adsorption and reaction (SILAR) method. Bulletin of Materials Science. 2004; 27 :85-111
- 4. Nicolau Y, Dupuy M, Brunel M. ZnS, CdS, and Zn 1-x Cd x S Thin Films Deposited by the Successive Ionic Layer Adsorption and Reaction Process. Journal of the Electrochemical Society. 1990; 137 :2915
- 5. Nicolau Y, Menard J. Solution growth of ZnS, CdS and Zn 1-X Cd X S thin films by the successive ionic-layer adsorption and reaction process; growth mechanism. Journal of Crystal Growth. 1988; 92 :128
- 6. Hossain MA, Farhad SFU, Tanvir NI, Chang JH, Rahman MA, Tanaka T, et al. Facile synthesis of Cu2O nanorods in the presence of NaCl by successive ionic layer adsorption and reaction method and its characterizations. RSC Open Science. 2022; 9 :211899
- 7. Ghos BC, Farhad SFU, Patwary MAM, Majumder S, Hossain MA, Tanvir NI, et al. Influence of the Substrate, Process Conditions and Post-annealing Temperature on the Properties of ZnO Thin Films Grown by the Successive Ionic Layer Adsorption and Reaction Method. Journal of ACS Omega. 2021; 6 (4):2665
- 8. Majumder S, Tanvir NI, Ghos BC, Patwary MAM, Rahman MA, Hossain MA, et al. Optimization of the growth conditions of Cu 2 O thin films and subsequent fabrication of Cu 2 O/ZnO heterojunction by m-SILAR method. IEEE WIECON-ECE. 2020:139
- 9. Ubale AU, Belkhedkar MR, Sakhare YS, Singh A, Gurada C, Kothari DC. Characterization of nanostructured Mn3O4 thin films grown by SILAR method at room temperature. Materials Chemistry and Physics. 2012; 136 :1067
- 10. Xiangdong G, Xiaomin L, Weidong Y, Preparation and characterization of highly oriented ZnO film by ultrasonic assisted SILAR method. Journal of Wuhan University of Technology, Materials Science Edition. 2005; 20 :23
- 11. Sfaelou S, Sygellou L, Dracopoulos V, Travlos A, Lianos P. Effect of the nature of Cadmium salts on the effectiveness of CdS SILAR deposition and Its consequences on the Performance of Sensitized Solar Cells. Journal of Physical Chemistry C. 2014; 118 :22873
- 12. Wang T, Luo Z, Li C, Gong J. Controllable fabrication of nanostructured materials for photoelectrochemical water splitting via atomic layer deposition. Chemical Society Reviews. 2014; 43 :7469
- 13. Pawar SM, Pawar BS, Kim JH, Joo O-S, Lokhande CD. Recent status of chemical bath deposited metal chalcogenide and metal oxide thin films. Current Applied Physics. 2011; 11 :117
- 14. Niesen TP, De Guire MR. Review: Deposition of Ceramic Thin Films at Low Temperatures from Aqueous Solution. Journal of Electroceramics. 2001; 6 :169
- 15. Sankapal BR, Ennaoui A, Guminskaya T, Dittrich T, Bohne W, Röhrich J, et al. Characterization of p-CuI prepared by the SILAR technique on Cu-tape/n-CuInS 2 for solar cells. Thin Solid Film. 2005; 480-481 :142-146
- 16. Oluyamo SS, Nyagba SM, Ojo SA. Optical Properties of Copper (I) Oxide Thin Films Synthesized by SILAR Technique. IOSR Journal of Applied Physics. 2014; 6 :102-110
- 17. Mageshwari K, Sathyamoorthy R. Physical properties of nanocrystalline CuO thin films prepared by the SILAR method. Materials Science in Semiconductor Processing. 2012; 16 :337-343
- 18. Mehrabian M. Optical and photovoltaic properties of ZnS nanocrystals fabricated on Al:ZnO films using the SILAR technique. Journal of Optical Technology. 2016; 83 :422-428
- 19. García C, Dávila S, Jardón G, Flores F, Bon R, Vorobiev YV. Characterization of PbS films deposited by successive ionic layer adsorption and reaction (SILAR) for CdS/PbS solar cells application. Materials Research Express. 2020; 7 :015530
- 20. Manikandan K, Dilip C, Mani P, Prince JJ. Deposition and Characterization of CdS Nano Thin Film with Complexing Agent Triethanolamine. American Journal of Engineering & Applied Science. 2015; 8 :318
- 21. Lokhande C, Shelke H, Raut V, Patil A, Lokhande A, Kim J. Facile synthesis of Cu2SnS3 thin films grown by SILAR method: effect of film thickness. Journal of Materials Science: Materials in Electronics. 2017; 28 :7912
- 22. Hodes G. Chemical Solution Deposition of Semiconductor Films. New York: Marcel Dekker; 2005
- 23. Lundin A, Kitaev G. Inorganic Materials. 1965; 1 :1900-1905
- 24. Nikam S, Suryawanshi M, Bhosale S, Gaikwad M, Shinde P, Moholkar A. Cu 2 O thin films prepared using modified successive ionic layer adsorption and reaction method and their use in photoelectrochemical solar cells. Journal of Materials Science: Materials in Electronics. 1897; 2016 :27
- 25. Das MR, Mukherjee A, Maiti P, Das S, Mitra P. Studies on multifunctional properties of SILAR synthesized CuO thin films for enhanced supercapacitor, photocatalytic and ethanol sensing applications. Journal of Electronic Materials. 2019; 48 :2718
- 26. Garcia FJ, Calderon CLL, Arbelaez DE, Del Real A, Garcia MR. Influence of substrate on structural, morphological and optical properties of ZnO films grown by SILAR method. Bulletin of Materials Science. 2014; 37 :1283
- 27. Gaikwad M, Mane A, Desai S, Moholkar A. Template-free TiO 2 photoanodes for dye-sensitized solar cell via modified chemical route. Journal of Colloid Interface, Science. 2017; 488 :269
- 28. Jambure S, Lokhande C. Photoelectrochemical solar cells with chemically grown CdO rice grains on flexible stainless-steel substrates. Materials Letters. 2013; 106 :133
- 29. Thakur AV, Lokhande BJ. Electrolytic anion affected charge storage mechanisms of Fe 3 O 4 flexible thin film electrode in KCl and KOH: a comparative study by cyclic voltammetry and galvanostatic charge-discharge. Journal of Materials Science: Materials in Electronics. 2017; 28 :11755
- 30. Dubal DP, Jagadale AD, Lokhande CD. Big as well as light weight portable, Mn3O4 based symmetric supercapacitive devices: Fabrication, performance evaluation and demonstration. Electrochimica Acta. 2012; 80 :160
- 31. Taşdemirci TCA. Influence of Annealing on Properties of SILAR Deposited Nickel Oxide Films. Vacuum. 2019; 167 :189
- 32. Shinde NM, Jagadale AD, Kumbhar VS, Rana TR, Kim J, Lokhande CD. Wet chemical synthesis of WO3 thin films for supercapacitor application. Korean Journal of Chemical Engineering. 2015; 32 :974
- 33. Raut SS, Bisen O, Sankapal BR. Synthesis of interconnected needle-like Bi2O3 using successive ionic layer adsorption and reaction towards supercapacitor application. Ionics. 1831; 2017 :23
- 34. Guney H, İskenderoğlu D. Synthesis of MgO thin films grown by SILAR technique, Synthesis of MgO thin films grown by SILAR technique. Ceramics International. 2018; 44 :7788
- 35. Guo W, Lian X, Nie Y, Hu M, Wu L, Gao H, et al. Facile growth of b-Cu2V2O7 thin films and characterization for photoelectrochemical water oxidation. Materials Letters. 2020; 258 :126842
- 36. Chemelewski WD, Mabayoje O, Mullins CB. SILAR Growth of Ag3VO4 and Characterization for Photoelectrochemical Water Oxidation. Journal of Physical Chemistry C. 2015; 119 :26803
- 37. Guo W, Tang D, Mabayoje O, Wygant BR, Xiao P, Zhang Y, et al. A Simplified Successive Ionic Layer Adsorption and Reaction (s-SILAR) Method for Growth of Porous BiVO4 Thin Films for Photoelectrochemical Water Oxidation. Journal of the Electrochemical Society. 2017; 164 :H119
- 38. Tang D, Rettie AJ, Mabayoje O, Wygant BR, Lai Y, Liu Y, et al. Facile Growth of Porous Fe 2 V4O 13 Films for Photoelectrochemical Water Oxidation. Journal of Materials Chemistry A. 2016; 4 :3034
- 39. Vadiyar MM, Kolekar SS, Deshpande NG, Chang J-Y, Kashale AA, Ghule AV. Binder-free chemical synthesis of ZnFe 2 O 4 thin films for asymmetric supercapacitor with improved performance. Ionics. 2017; 23 :741
- 40. Gong J, Wang X, Li X, Wang K. Highly sensitive visible light activated photoelectrochemical biosensing of organophosphate pesticide using biofunctional crossed bismuth oxyiodide flake arrays. Biosensors & Bioelectronics. 2012; 38 :43
- 41. Chavan HS, Hou B, Ahmed ATA, Jo Y, Cho S, Kim J, et al. Nanoflake NiMoO 4 based smart supercapacitor for intelligent power balance monitoring. Solar Energy Materials & Solar Cells. 2018; 185 :166
- 42. Babar P, Lokhande A, Shim H, Gang M, Pawar B, Pawar S, et al. SILAR deposited iron phosphate as a bifunctional electrocatalyst for efficient water splitting. Journal of Colloid and Interface Science. 2019; 534 :350
- 43. Ali Yildirm M, Ates A, Astam A. Annealing and light effect on structural, optical and electrical properties of CuS, CuZnS and ZnS thin films grown by the SILAR method. Physica E. 2009; 41 :1365-1372
- 44. Paul G, Chatterjee S, Pal AJ. Heterovalent doping and energy level tuning in Ag 2 S thin-films through solution approach: pn-Junction solar cells. Solar Energy Materials & Solar Cells. 2018; 182 :339
- 45. Pathan H, Salunkhe P, Sankapal B, Lokhande C. Photoelectrochemical investigation of Ag2S thin films deposited by SILAR method. Materials Chemistry and Physics. 2001; 72 :105
- 46. Veerathangam K, Pandian MS, Ramasamy P. Size-dependent photovoltaic performance of cadmium sulfide (CdS) quantum dots for solar cell applications. Journal of Alloys and Compounds. 2018; 735 :202
- 47. Sartale S, Lokhande C. Preparation and characterization of nickel sulphide thin films using successive ionic layer adsorption and reaction (SILAR) method. Materials Chemistry and Physics. 2001; 72 :101
- 48. Gao C, Shen H, Sun L, Huang H, Lu L, Cai H. Preparation of SnS films with zinc blende structure by successive ionic layer adsorption and reaction method. Materials Letters. 2010; 64 :2177
- 49. Abbas MA, Basit MA, Park TJ, Bang JH. Enhanced performance of PbS-sensitized solar cells via controlled successive ionic-layer adsorption and reaction. Physical Chemistry Chemical Physics. 2015; 17 :9752
- 50. Sartale S, Lokhande C. Studies on large area (∼50 cm2) MoS2 thin films deposited using successive ionic layer adsorption and reaction (SILAR) method. Materials Chemistry and Physics. 2001; 71 :94
- 51. Sartale S, Lokhande C. Preparation and characterization of As 2 S 3 thin films deposited using successive ionic layer adsorption and reaction (SILAR) method. Materials Research Bulletin. 2000; 35 :1345
- 52. Wang Y, Chen J, Jiang L, Liu F, Lai Y, Li J. Characterization of Bi 2 S 3 thin films synthesized by an improved successive ionic layer adsorption and reaction (SILAR) method. Materials Letters. 2017; 209 :479
- 53. Yıldırım MA, Yıldırım ST, Cavanmirza İ, Ateş A. Chemically synthesis and characterization of MnS thin films by SILAR method. Chemical Physics Letters. 2016; 647 :73
- 54. Deshpande M, Chauhan K, Patel KN, Rajput P, Bhoi HR, Chaki S. Study of Sb2S3 thin films deposited by SILAR method. Materials Research Express. 2018; 5 :056410
- 55. Karade SS, Dwivedi P, Majumder S, Pandit B, Sankapal BR. First report on FeS based 2 V operating flexible solid-state symmetric supercapacitor device. Sustainable Energy & Fuels. 2017; 1 :1366
- 56. Manikandan K, Mani P, Dilip CS, Valli S, Inbaraj PFH, Prince JJ. Effect of complexing agent TEA: The structural, morphological, topographical and optical properties of FexSx nano thin films deposited by SILAR technique. Applied Surface Science. 2014; 288 :76
- 57. Sartale S, Lokhande C. Deposition of cobalt sulfide thin films by successive ionic layer adsorption and reaction (SILAR) method, and their characterization. Indian Journal of Pure and Applied Physics. 2000; 38 :48
- 58. Patil S, Lokhande A, Lokhande C. Effect of aqueous electrolyte on pseudocapacitive behavior of chemically synthesized La2S3 electrode. Materials Science in Semiconductor Processing. 2016; 41 :132
- 59. Astam A, Akaltun Y, Yıldırım M. Conversion of SILAR deposited Cu 3 Se 2 thin films to Cu 2-x Se by annealing. Materials Letters. 2016; 166 :9
- 60. Zhao B, Wan Z, Luo J, Han F, Malik HA, Jia C, et al. Efficient Sb 2 Se 3 sensitized solar cells prepared through a facile SILAR process and improved performance by interface modification. Applied Surface Science. 2018; 450 :228
- 61. Chaudhari K, Gosavi NM, Deshpande N, Gosavi S. Chemical synthesis and characterization of CdSe thin films deposited by SILAR technique for optoelectronic applications. Journal of Science: Advanced Materials Devices. 2016; 1 :476
- 62. Lokhande C, Sankapal B, Sartale S, Pathan H, Giersig M, Ganesan V. A novel method for the deposition of nanocrystalline Bi 2 Se 3 , Sb 2 Se 3 and Bi 2 Se 3 –Sb 2 Se 3 thin films — SILAR. Applied Surface Science. 2001; 182 :413
- 63. Ubale A, Dhokne R, Chikhlikar P, Sangawar V, Kulkarni D. Characterization of nanocrystalline cadmium telluride thin films grown by successive ionic layer adsorption and reaction (SILAR) method. Bulletin of Materials Science. 2006; 29 :165
- 64. Pathan H, Lokhande C, Amalnerkar D, Seth T. Preparation and characterization of copper telluride thin films by modified chemical bath deposition (M-CBD) method. Applied Surface Science. 2003; 218 :291
- 65. Patil S, Lokhande A, Lee D-W, Kim J, Lokhande C. Chemical synthesis and supercapacitive properties of lanthanum telluride thin film. Journal of Colloid and Interface Science. 2017; 490 :147
- 66. Kulal PM, Dubal DP, Lokhande CD, Fulari VJ. Chemical synthesis of Fe2O3 thin films for supercapacitor application. Journal of Alloys and Compounds. 2011; 509 :2567
- 67. Yıldırım MA, Akaltun Y, Ateş A. Characteristics of SnO 2 thin films prepared by SILAR, Characteristics of SnO 2 thin films prepared by SILAR. Solid State Sciences. 2012; 14 :1282
- 68. Yassin SNH, Sim ASL, Jennings JR. Photoelectrochemical evaluation of SILAR-deposited nanoporous BiVO4 photoanodes for solar-driven water splitting. Nano Materials Science. 2020; 2 :227
- 69. Putri AA, Kato S, Kishi N, Soga T. Relevance of precursor molarity in the prepared bismuth oxyiodide films by successive ionic layer adsorption and reaction for solar cell application. Journal of Science: Advanced Materials Devices. 2019; 4 :116
- 70. Sfaelou S, Raptis D, Dracopoulos V, Lianos P. BiOI solar cells. RSC Advances. 2015; 5 :95813
- 71. Hu L, Liao Y, Xia D, Zhang Q, He H, Yang J, et al. In-situ fabrication of AgI-BiOI nanoflake arrays film photoelectrode for efficient wastewater treatment, electricity production and enhanced recovery of copper in photocatalytic fuel cell. Catalysis Today. 2020; 339 :379
- 72. Zhang Y, Chong X, Sun H, Kedir MM, Kim KJ, Ohodnicki PR, et al. Silver nanoplate aggregation based multifunctional black metal absorbers for localization, photothermic harnessing enhancement and omnidirectional light antireflection. Journal of Materials Chemistry C. 2018; 6 :989
- 73. Mehrabian M, Esteki Z. Degradation of methylene blue by photocatalysis of copper assisted ZnS nanoparticle thin films. Optik. 2017; 130 :1168
- 74. Zhao J, Burke AF. Electrochemical Capacitors: Performance Metrics and Evaluation by Testing and Analysis. Advanced Energy Materials. 2020; 11 :2002192
- 75. Dubal D, Dhawale D, Salunkhe R, Lokhande C. A novel chemical synthesis of Mn3O4 thin film and its stepwise conversion into birnessite MnO2 during super capacitive studies. Journal of Electroanalytical Chemistry. 2010; 647 :60
- 76. Nwankwo M, Nwanya A, Agbogu A, Ekwealor A, Ejikeme PM, Bucher R, et al. Electrochemical Supercapacitive Properties of SILAR-Deposited Mn 3 O 4 Electrodes. Vacuum. 2018; 158 :206
- 77. Kumbhar VS, Lee YR, Ra CS, Tuma D, Min B-K, Shim JJ. Modified chemical synthesis of MnS nanoclusters on nickel foam for high performance all-solid-state asymmetric supercapacitors. RSC Advances. 2017; 7 :16348
- 78. Das MR, Roy A, Mpelane S, Mukherjee A, Mitra P, Das S. Influence of dipping cycle on SILAR synthesized NiO thin film for improved electrochemical performance. Electrochimica Acta. 2018; 273 :105
- 79. Shinde S, Ghodake G, Fulari V, Kim D-Y. High electrochemical performance of nanoflakes like CuO electrode by successive ionic layer adsorption and reaction (SILAR) method. Journal of Industrial and Engineering Chemistry. 2017; 52 :12
- 80. Shinde SK, Yadav HM, Ramesh S, Bathula C, Maile N, Ghodake GS, et al. High -Performance Symmetric Supercapacitor; Nanoflower -Like NiCo 2 O 4 //NiCo 2 O 4 Thin Films Synthesized by simple and highly stable chemical method. Journal of Molecular Liquids. 2020; 299 :112119
- 81. Chavan HS, Hou B, Ahmed ATA, Kim J, Jo Y, Cho S, et al. Ultrathin Ni-Mo oxide nanoflakes for high-performance supercapacitor electrodes. Journal of Alloys and Compounds. 2018; 767 :782
- 82. Pusawale SN, Deshmukh PR, Jadhav PS, Lokhande CD. Electrochemical properties of chemically synthesized SnO 2 -RuO 2 mixed films. Materials for Renewable and Sustainable Energy. 2018; 8 :1
- 83. Kumar N, Mishra D, Kim SY, Jin SH. Two dimensional, bi-layered SnS 2 @Co 3 S 4 heterostructure formation via SILAR method: Toward high performance supercapacitors with superior electrodes. Materials Letters. 2020; 262 :127173
- 84. Gund GS, Dubal DP, Shinde SS, Lokhande CD. Architectured Morphologies of Chemically Prepared NiO/MWCNTs Nanohybrid Thin Films for High Performance Supercapacitors. ACS Applied Materials & Interfaces. 2014; 6 :3176
- 85. Kumar N, Sahoo P, Panda H. Tuning the electro-chemical properties by substituting selectively transition metals on carbon in Ni/Co oxide-carbon composite electrode for supercapacitor device. New Journal of Chemistry. 2017; 41 :3562
- 86. Pandit B, Pande SA, Sankapal BR. Facile SILAR Processed Bi 2 S 3 :PbS Solid Solution on MWCNTs for High-performance Electrochemical Supercapacitor. Chinese Journal of Chemistry. 2019; 37 :1279
- 87. Raut SS, Sankapal BR. Porous zinc cobaltite (ZnCo2O4) film by successive ionic layer adsorption and reaction towards solid-state symmetric supercapacitive device. Journal of Colloid and Interface Science. 2017; 487 :201
- 88. Raut SS, Sankapal BR. First report on synthesis of ZnFe 2 O 4 thin film using successive ionic layer adsorption and reaction: Approach towards solid-state symmetric supercapacitor device. Electrochimica Acta. 2016; 198 :203
- 89. Kavyashree S, Parveen SK, Sharma SN. Solid-state symmetric supercapacitor based on Y doped Sr(OH) 2 using SILAR method. Pandey, Energy. 2020; 197 :117163
- 90. Buathet S, Simalaotao K, Reunchan P, Vailikhit V, Teesetsopon P, Raknual D, et al. Tubtimtae, Electrochemical performance of Bi 2 Te 3 heterostructure thin film and Cu 7 Te 4 nanocrystals on undoped and In 3+ -doped WO 3 films for energy storage applications. Electrochimica Acta. 2020; 341 :136049
- 91. Becquerel E. On Electron Effects under the Influence of Solar Radiation. Comptes. 1839; 9 (144):561
- 92. Fritts CE. On a New Form of Selenium Cell, and some Electrical Discoveries made by its use. American Journal of Science. 1883; 26 :465
- 93. Ohl R. U. S. Patent 2. 1946; 402 :662
- 94. Chapin D, Fuller C, Pearson G. A New silicon p-n Junction Photocell for Converting Solar Radiation into Electrical Power. Journal of Applied Physics. 1954; 25 (5):676
- 95. National Renewable Energy Laboratory, NREL, USA. Available from: https://www.nrel.gov/pv/interactive-cell-efficiency.html
- 96. Shockley W, Queisser HJ. Detailed Balance Limit of Efficiency of p-n Junction Solar Cells. Journal of Applied Physics. 1961; 32 (3):510
- 97. Maycock PD. PV review: World Solar PV market continues explosive growth. Refocus. 2005; 6 :18
- 98. Inaba T, Kobayashi E, Suwazono Y, Uetani M, Oishi M, Nakagawa H, et al. Estimation of Cumulative Cadmium Intake Causing Itai-itai Disease. Toxicology Letters. 2005; 159 :192-201
- 99. Baba H, Tsuneyama K, Yazaki M, Nagata K, Minamisaka T, Tsuda T, et al. The liver in itai-itai disease (chronic cadmium poisoning): pathological features and metallothionein expression. Modern Pathology. 2013; 26 :1228
- 100. Pan J, Plant JA, Voulvoulis N, Oates CJ, Ihlenfeld C. Ihlenfeld, Cadmium levels in Europe: implications for human health. Environmental Geochemistry and Health. 2010; 32 :1-12
- 101. Bertin G, Averbeck D. Cadmium: cellular effects, modifications of biomolecules, modulation of DNA repair and genotoxic consequences (a review). Biochimie. 2006; 88 :1549-1559
- 102. Gaikwad M, Suryawanshi M, Maldar P, Dongale T, Moholkar A. Nanostructured zinc oxide photoelectrodes by green routes M-SILAR and electrodeposition for dye sensitized solar cell. Optical Materials. 2018; 78 :325
- 103. Divitini G, Cacovich S, Matteocci F, Cinà L, Di Carlo A, Ducati C. In situ observation of heat-induced degradation of perovskite solar cells. Nature Energy. 2016; 1 :15012
- 104. Pathak SK, Abate A, Leijtens T, Hollman DJ, Teuscher J, Pazos L, et al. Towards Long-Term Photostability of Solid-State Dye Sensitized Solar Cells. Advanced Energy Materials. 2014; 4 (8):1301667
- 105. Rühle S, Anderson AY, Barad HN, Kupfer B, Bouhadana Y, Hodesh ER, et al. All-Oxide Photovoltaics. Journal of Physical Chemistry Letters. 2012; 3 :3755
- 106. Dittrich T, Belaidi A, Ennaoui A. Ennaoui, Concepts of inorganic solid-state nanostructured solar cells. Solar Energy Materials & Solar Cells. 2011; 95 :1527
- 107. Thakur AV, Lokhande BJ. Effect of the molar concentration of pyrrole monomer on the rate of polymerization, growth and hence the electrochemical behavior of highly pristine PPy flexible electrodes. Heliyon. 2019; 5 :e02909
- 108. Badawi A, Al-Baradi AM, Atta AA, Algarni SA, Almalki ASA, Alharthi SS. Graphene/TiO 2 nanocomposite electrodes sensitized with tin sulfide quantum dots for energy issues. Physica E. 2020; 121 :114121
- 109. Wang QH, Kalantar-zadeh K, Coleman KAJN, Strano MS. Electronics and optoelectronics of two-dimensional transition metal dichalcogenides. Nature Nanotechnology. 2012; 7 :699-712
- 110. Manzeli S, Ovchinnikov D, Pasquier D, Yazyev OV, Kis A. 2D transition metal dichalcogenides. Nature Reviews Materials. 2017; 2 (8):17033
- 111. Tack LW, Azam MA, Seman RNAR. Structural and Electronic Properties of Transition-Metal Oxides Attached to a Single-Walled CNT as a Lithium-Ion Battery Electrode: A First-Principles Study. The Journal of Physical Chemistry A. 2017; 121 (13):2636
- 112. Chatterjee S, Pal AJ. Introducing Cu2O Thin-Films as a Hole-Transport Layer in Efficient Planar Perovskite Solar Cell Structures. Journal of Physical Chemistry C. 2016; 120 :1428
- 113. Gaikwad M, Suryawanshi M, Nikam S, Bhosale C, Kim J, Moholkar A. Influence of Zn concentration and dye adsorption time on the photovoltaic performance of M- SILAR deposited ZnO-based dye sensitized solar cells. Journal of Photochemistry and Photobiology, A: Chemistry. 2016; 329 :246
- 114. Shaikh SF, Ghule BG, Nakate UT, Shinde PV, Ekar SU, O'Dwyer C, et al. Low-Temperature Ionic Layer Adsorption and Reaction Grown Anatase TiO2 Nanocrystalline Films for Efficient Perovskite Solar Cell and Gas Sensor Applications. Scientific Reports. 2018; 8 :11016
- 115. Sankapal B, Lokhande CD. Photoelectrochemical characterization of Bi2Se3 thin films deposited by SILAR technique. Materials Chemistry and Physics. 2002; 73 :151
- 116. Luo J, Wang YX, Sun J, Yang ZS, Zhang QF. MnS passivation layer for highly efficient ZnO-based quantum dotsensitized solar cells. Solar Energy Materials & Solar Cells. 2018; 187 :199
- 117. Becker MA, Radich JG, Bunker BA, Kamat PV. How Does a SILAR CdSe Film Grow? Tuning the Deposition Steps to Suppress Interfacial Charge Recombination in Solar Cells. Journal of Physical Chemistry Letters. 2014; 5 :1575
- 118. Santra PK, Kamat PV. Mn-Doped Quantum Dot Sensitized Solar Cells: A Strategy to Boost Efficiency over 5%. Journal of the American Chemical Society. 2012; 134 :2508
- 119. Zhou R, Zhang Q, Tian J, Myers D, Yin M, Cao G. Influence of Cationic Precursors on CdS Quantum-Dot-Sensitized Solar Cell Prepared by Successive Ionic Layer Adsorption and Reaction. Journal of Physical Chemistry C. 2013; 117 :26948
- 120. Lee JW, Son D-Y, Ahn TK, Shin H-W, Kim IY, Hwang S-J, et al. Quantum-Dot-Sensitized Solar Cell with Unprecedentedly High Photocurrent. Scientific Reports. 2013; 3 :1050
- 121. Tian J, Shen T, Liu X, Fei C, Lv L, Cao G. Enhanced Performance of PbS quantum-dot-sensitized Solar Cells via Optimizing Precursor Solution and Electrolytes. Scientific Reports. 2016; 6 :1
- 122. Kumar DK, Loskot J, Křiž J, Bennett N, Upadhyaya HM, Sadhu V, et al. Synthesis of SnSe quantum dots by successive ionic layer adsorption and reaction (SILAR) method for efficient solar cells applications. Solar Energy. 2020; 199 :570
- 123. Visalakshi S, Kannan R, Valanarasu S, Kim H-S, Kathalingam A, Chandramohan R. Effect of bath concentration on the growth and photovoltaic response of SILAR-deposited CuO thin films. Applied Physics A: Materials Science & Processing. 2015; 120 :1105
- 124. Chatterjee S, Saha SK, Pal AJ. Formation of all-oxide solar cells in atmospheric condition based on Cu 2 O thin films grown through SILAR technique. Solar Energy Materials & Solar Cells. 2016; 147 :17
- 125. Jambure SB, Gund GS, Dubal DP, Shinde SS, Lokhande CD. Cost effective facile synthesis of TiO2 nanograins for flexible DSSC application using rose bengal dye. Electronic Materials Letters. 2014; 10 :943
- 126. Waghmare M, Beedri N, Baviskar P, Pathan H, Ubale A. Effect of ZrO 2 barrier layers on the photovoltaic parameters of rose bengal dye sensitized TiO 2 solar cell. Journal of Materials Science: Materials in Electronics. 2019; 30 :6015
- 127. Lee H, Wang M, Chen P, Gamelin DR, Zakeeruddin SM, Gratzel M, et al. Nazeeruddin, Efficient CdSe Quantum Dot-Sensitized Solar Cells Prepared by an Improved Successive Ionic Layer Adsorption and Reaction Process. Nano Letters. 2009; 9 :4221
- 128. She H, Sun Y, Li S, Huang J, Wang L, Zhu G, et al. Synthesis of non-noble metal nickel doped sulfide solid solution for improved photocatalytic performance. Applied Catalysis, B: Environmental. 2019; 245 :439-447
- 129. Zhou S, Yue P, Huang J, Wang L, She H, Wang Q. High-performance photoelectrochemical water splitting of BiVO4@Co-MIm prepared by a facile in-situ deposition method. Journal of Chemical Engineering. 2019; 371 :885-892
- 130. Adam RE, Pirhashemi M, Elhag S, Liu X, Habibi-Yangjeh A, Willander M, et al. Nur, ZnO/Ag/Ag2WO4 photo-electrodes with plasmonic behavior for enhanced photoelectrochemical water oxidation. RSC Advances. 2019; 9 :8271
- 131. Pirhashemi M, Habibi-Yangjeh A. Ultrasonic-assisted preparation of plasmonic ZnO/Ag/Ag2WO4 nanocomposites with high visible-light photocatalytic performance for degradation of organic pollutants. Journal of Colloid and Interface Science. 2017; 491 :216-229
- 132. Ghobadifard M, Mohebbi S. Novel nanomagnetic Ag/β-Ag 2 WO 4 /CoFe 2 O 4 as a highly efficient photocatalyst under visible light irradiation. New Journal of Chemistry. 2018; 42 :9530-9542
- 133. Pan L, Zhao L, Liu Z. High-efficient TiO 2 NRs/BiOI NSs heterojunction photoanodes for photoelectrochemical water splitting,. Materials and Technologies. 2017; 32 :823
- 134. Yassin BH, Halidi NAH, Sim SL, Liu YR, Jennings JR. Electron Diffusion Length and Charge Separation Efficiency in Nanostructured Ternary Metal Vanadate Photoelectrodes. Materials Science Forum. 2018; 941 :2121
- 135. Xiong Y, Yang L, He H, Wan J, Xiao P, Guo W. Enhanced charge separation and transfer by Bi2MoO6@Bi2Mo2O9 compound using SILAR for photoelectrochemical water oxidation. Electrochimica Acta. 2018; 264 :26
- 136. Stoll T, Zafeiropoulos G, Dogan I, Genuit H, Lavrijsen R, Koopmans B, et al. Tsampas, Visible-light-promoted gas-phase water splitting using porous WO 3 /BiVO 4 photoanodes. Electrochemistry Communications. 2017; 82 :47
- 137. Abel AJ, Garcia-Torregrosa I, Patel AM, Opasanont B, Baxter JB. SILAR-Deposited Hematite Films for Photoelectrochemical Water Splitting: Effects of Sn, Ti, Thickness, and Nano structuring. Journal of Physical Chemistry C. 2015; 119 :4454
- 138. Abel AJ, Patel AM, Smolin SY, Opasanont B, Baxter JB. Enhanced Photoelectrochemical Water Splitting via SILAR-Deposited Ti-Doped Hematite Thin Films with an FeOOH Overlayer. Journal of Materials Chemistry A. 2016; 4 :6495
- 139. Zhong X, He H, Du J, Ren Q, Huang J, Tang Y, et al. Boosting solar water oxidation activity and stability of BiVO 4 photoanode through the Co-catalytic effect of CuCoO 2 . Electrochimica Acta. 2019; 304 :301
- 140. Majumder S, Quang ND, Hien TT, Chinh ND, Hung NM, Yang H, et al. Effect of SILAR-anchored ZnFe2O4 on the BiVO4 nanostructure: An attempt towards enhancing photoelectrochemical water splitting. Applied Surface Science. 2021:149033
- 141. Zhou M, Guo Z, Song Q, Li X, Liu Z. Improved photoelectrochemical response of CuWO 4 /BiOI p-n heterojunction embedded with plasmonic Ag nanoparticles. Chemical Engineering Journal. 2019; 370 :218
- 142. Xia L, Bai J, Li J, Zeng Q, Li L, Zhou B. High-performance BiVO4 photoanodes cocatalyzed with an ultrathin -Fe 2 O 3 layer for photoelectrochemical application. Applied Catalysis, B: Environmental. 2017; 204 :127
- 143. Zhou W, Jiang T, Zhao Y, Xu C, Pei C, Xue H. Ultrathin TiO2/BiVO4 nanosheet heterojunction arrays modified with NiFe-LDH nanoparticles for enhanced photoelectrochemical oxidation of water. Journal of Colloid and Interface Science. 2019; 549 :42
- 144. Yan L, Zhao W, Liu Z. 1D ZnO/BiVO 4 heterojunction photoanodes for efficient photoelectrochemical water splitting, Dalton Transactions. 2016; 45 :11346
- 145. Gao L, Long X, Wei S, Wang C, Wang T, Li F, et al. Facile growth of AgVO 3 nanoparticles on Mo-doped BiVO4 film for enhanced photoelectrochemical water oxidation. Chemical Engineering Journal. 2019; 378 :122193
- 146. Sharma P, Jang JW, Lee JS. Key Strategies to Advance the Photoelectrochemical Water Splitting Performance of α-Fe 2 O 3 Photoanode. ChemCatChem. 2019; 11 :157
- 147. Jiang C, Moniz SJA, Wang A, Zhang T, Tang J. Photoelectrochemical devices for solar water splitting - materials and challenges. Chemical Society Reviews. 2017; 46 :4645
- 148. Baig F, Khattak YH, Soucase BM, Beg S, Ullah S. Effect of anionic bath temperature on morphology and photo electrochemical properties of Cu2O deposited by SILAR,. Materials Science in Semiconductor Processing. 2018; 88 :35
- 149. Wang Q, Sun C, Liu Z, Tan X, Zheng S, Zhang H, et al. Ultrasound-assisted successive ionic layer adsorption and reaction synthesis of Cu 2 O cubes sensitized TiO2 nanotube arrays for the enhanced photoelectrochemical performance. Materials Research Bulletin. 2019; 111 :277
- 150. Desai MA, Sharma V, Prasad M, Jadkar S, Saratale GD, Sartale SD. Seed-layer-free deposition of well-oriented ZnO nanorods thin films by SILAR and their photoelectrochemical studies. International Journal of Hydrogen Energy. 2020; 45 :5783
- 151. Jimenez-Gonzalez A, Suarez-Parra R. Effect of heat treatment on the properties of ZnO thin films prepared by successive ion layer adsorption and reaction (SILAR). Journal of Crystal Growth. 1996; 167 :649
- 152. Patil UM, Gurav KV, Joo O-S, Lokhande CD. Synthesis of photosensitive nanograined TiO2 thin films by SILAR method. Journal of Alloys and Compounds. 2009; 478 :711
- 153. Pathan HM, Min S-K, Desai JD, Jung K-D, Joo O-S. Preparation and characterization of titanium dioxide thin films by SILAR method. Materials Chemistry and Physics. 2006; 97 :5
- 154. Shameem A, Devendran P, Siva V, Raja M, Asath Bahadur S, Manikandan A. Asath Bahadur, A. Manikandan, Preparation and Characterization Studies of Nanostructured CdO Thin Films by SILAR Method for Photocatalytic Applications. Journal of Inorganic and Organometallic Polymers. 2017; 27 :692
- 155. Desai MA, Sartale SD. Facile Soft Solution Route to Engineer Hierarchical Morphologies of ZnO Nanostructures. Crystal Growth & Design. 2015; 15 :4813
- 156. Senthamilselvi V, Saravanakumar K, Jabena Begum N, Anandhi R, Ravichandran AT, Sakthivel B, et al. Photovoltaic properties of nanocrystalline CdS films deposited by SILAR and CBD techniques-a comparative study. Journal of Materials Science: Materials in Electronics. 2012; 23 :302
- 157. Shen T, Tian J, Lv L, Fei C, Wang Y, Pullerits T, et al. Investigation of the role of Mn dopant in CdS quantum dot sensitized solar cell, Electrochimica Acta. Electrochimica Acta. 2016; 191 :62-69
- 158. Ravichandran K, Rajkumar P, Sakthivel B, Swaminathan K, Chinnappa L. Role of precursor material and annealing ambience on the physical properties of SILAR deposited ZnO films. Ceramics International. 2014; 40 :12375
- 159. Kumar KDA, Valanarasu S, Ganesh V, Shkir M, Kathalingam A, Alfaify S. Effect of Precursors on Key Opto-electrical Properties of Successive Ion Layer Adsorption and Reaction-Prepared Al:ZnO Thin Films. Journal of Electronic Materials. 2018; 47 :2
- 160. Shaikh AA, Waikar MR, Sonkawade RG. Effect of different precursors on electrochemical properties of manganese oxide thin films prepared by SILAR method, Synthetic Metals. Synthetic Metals. 2019; 247 :1-9
- 161. Kumar P, Gowrish KR. The effect of precursor concentration and post-deposition annealing on the optical and micro-structural properties of SILAR deposited SnO 2 films. Materials Research Express. 2020; 7 :016428
- 162. Hernández RG, Cruz MRA, Aguilar NP, Aguilar MER, López MQ, Guerra EM, Tostado FSA. A. Méndez-Vilas Ed. 431
- 163. Kakade BN, Nikam CP, Gosavi SR. Effect of immersion cycles on structural, morphology and optoelectronic properties of nanocrystalline Ag2S thin films deposited by SILAR technique. IOSR Applied Physics. 2014; 6 (6):06-12
- 164. Nwanya AC, Cosmas Chigbo SC, Ezugwu RU, Osuji M, Malik FI, Ezema A. Transformation of cadmium hydroxide to cadmium oxide thin films synthesized by SILAR deposition process: Role of varying deposition cycles. Arab Universities Basic and Applied Sciences. 2016; 20 :49
- 165. Ashith VK, Gowrish RK. Structural and Optical Properties of ZnS Thin Films by SILAR Technique obtained by acetate Precursor. IOP Conference Series: Materials Science and Engineering. 2018; 360 :012058
- 166. Preetha KC, Murali KV, Ragina AJ, Deepa K, Remadevi TL. Effect of cationic precursor pH on optical and transport properties of SILAR deposited nano crystalline PbS thin films. Current Applied Physics. 2012; 12 :53
- 167. Sakthivelu A, Valanarasu S, Prince JJ. Effect of pH on SILAR deposited ZnO thin films. International Journal of Chemical Sciences. 2009; 7 (4):2463
- 168. Patwary MAM, Hossain MA, Ghos BC, Chakrabarty J, Haque SR, Rupa SA, et al. Copper oxide nanostructured thin films processed by SILAR for optoelectronic applications. RSC Advances. 2022; 12 :32853
- 169. Farhad SFU, Hossain MA, Tanvir NI, Akter R, Patwary MAM, Shahjahan M, et al. Structural, optical, electrical and photoelectrochemical properties of cuprous oxide thin films by modified SILAR method. Materials Science in Semiconductor Processing. 2019; 95 :68
- 170. Farhad SFU, Majumder S, Hossain MA, Tanvir NI, Akter R, Patwary MAM. Patwary, Effect of Solution pH and post-annealing temperature on the properties of the Optical Bandgap of the Copper Oxide Thin Films Grown by modified SILAR Method. MRS Advances. 2019; 4 (16):937
- 171. Gençyılmaz O, Taşköprü T. Effect of pH on the synthesis of CuO films by SILAR method. Journal of Alloys and Compounds. 2016; 695 :1205-1212
- 172. Visalakshi S, Kannan R, Valanarasu S, Kathalingam A, Rajashabala S. Studies on optical and electrical properties of SILAR-deposited CuO thin films. Materials Research Innovations. 2016
- 173. Cavusoglu H. Structural, morphological and optical studies of nanostructured cadmium oxide films: the role of pH. Journal of Materials Science: Materials in Electronics. 2018; 29 :12777
- 174. Lokhande CD, Patil PS, Tributsch H, Ennaoui A. Ennaoui, ZnSe thin films by chemical bath deposition method. Solar Energy Materials & Solar Cells. 1998; 55 :379
- 175. Patwary MAM, Saito K, Guo Q, Tanaka T. Tanaka, Influence of oxygen flow rate and substrate positions on properties of Cu-oxide thin films fabricated by radio frequency magnetron sputtering using pure Cu target. Thin Solid Films. 2019; 675 :59-65
- 176. Ashith VK, Rao GK, Moger SN, Smitha R. Effect of post-deposition annealing on the properties of ZnO films obtained by high temperature, micro-controller-based SILAR deposition. Ceramics International. 2018; 44 (15):10669-10676
- 177. Serin N, Serin T, Horzum S, Celik Y. Annealing effects on the properties of copper oxide thin films prepared by chemical deposition, Semicond. Semiconductor Science and Technology. 2005; 20 :398-401
- 178. Tasdemirci TC. Copper Oxide Thin Films Synthesized by SILAR: Role of Varying Annealing Temperature. Electronic Materials Letters . 2020; 16 :239
- 179. Gokul B, Matheswaran P, Sathyamoorthy R. Sathyamoorthy, Influence of Annealing on Physical Properties of CdO Thin Films Prepared by SILAR Method. Journal of Materials Science and Technology. 2013; 29 (1):17-21
- 180. Iskenderoglu D, Guney H. Effect of Annealing on the Structural, Morphological and Optical Properties of MgO Nanowall Structures Grown by SILAR Method. Electronic Materials. 2019; 48 :5850-5856
- 181. Patwary MAM, Saito K, Guo Q, Tanaka T, Yu KM, Walukiewicz W. Walukiewicz, Nitrogen Doping Effect in Cu4O3 Thin Films Fabricated by Radio Frequency Magnetron Sputtering. Physica Status Solidi B. 2020; 257 (2):1900363
- 182. Patwary MAM, Ohishi M, Saito K, Guo Q, Yu KM, Tanaka T. Effect of Nitrogen Doping on Structural, Electrical, and Optical Properties of CuO Thin Films Synthesized by Radio Frequency Magnetron Sputtering for Photovoltaic Application. ECS Journal of Solid State Science and Technology. 2021; 10 :065019
- 183. Ashcroft NW, Mermin ND. Solid State Physics Holt. New York: Rinehart and Winston; 1976
- 184. Hossain MA, Patwary MAM, Rahman MM, Ohtsu Y. Properties of AZO thin films prepared by stationary and rotating RF magnetized plasma sputtering source. AIP Advances. 2022; 12 :015224
- 185. Saha S, Jana M, Khanra P, Samanta P, Koo H, Murmu NC, et al. Band gap modified boron doped NiO/Fe3O4 nanostructure as the positive electrode for high energy asymmetric supercapacitors. RSC Advances. 2016; 6 :1380
- 186. Zhu X, Zou X, Zhou H. Effects of Different Doping Ratio of Cu Doped CdS on QDSCs Performance. Journal of Nanomaterials. 2015; 498950 :4
- 187. Belkhedkar MR, Ubale AU, Sakhare YS, Zubair N, Musaddique M. Musaddique, Characterization and antibacterial activity of nanocrystalline Mn doped Fe 2 O 3 thin films grown by successive ionic layer adsorption and reaction method. Association of Arab Universities Basic & Applied Science. 2016; 21 :38
- 188. Bayram O, Guney H, Ertargin ME, Igman E, Simsek O. Effect of doping concentration on the structural and optical properties of nanostructured Cu-doped Mn3O4 films obtained by SILAR technique. Applied Physics A. 2018; 124 :606
- 189. F. Bayansal, T. Tas¸ Ko Pru, Bu Nyamin S, Ahin, Haci Ali C Etinkara. Effect of Cobalt Doping on Nanostructured CuO Thin Films. Metallurgical & Materials Transactions A, 45A, (2014) 3671
- 190. Yuksel M, Pennings JR, Bayansal F, Yeow JTW. Effect of B-doping on the morphological, structural and optical properties of SILAR deposited CuO films. Physica B: Condensed Matter. 2020; 599 :412578
- 191. Dhanabalan K, Ravichandran AT, Ravichandran K, Valanarasu S, Mantha S. Effect of Co doped material on the structural, optical and magnetic properties of Cu 2 O thin films by SILAR technique. Materials Science: Materials in Electronics. 2017; 28 :4431
- 192. Satheeskumar S, Vadivel S, Dhanabalan K, Vasuhi A, Ravichandran AT, Ravichandran K. Enhancing the structural, optical and magnetic properties of Cu 2 O films deposited using a SILAR technique through Fe-doping. Journal of Materials Science: Materials Electronics. 2018; 29 :9354
- 193. Iskenderoglu D, Güney H, Güldüren ME. Chromium - An effective dopant for engineering the structural and the optical properties of CdO nanostructures grown by SILAR method. Optical Materials. 2021; 115 :111067
- 194. Sahin B, Bayansal F, Yüksel M. Influence of manganese concentration and annealing temperatures on the physical properties of CdO films grown by the SILAR method. Philosophical Magazine. 2014; 94 (9):956-963
- 195. Yüksel M, Şahin B, Bayansal F. Nano structured CdO films grown by the SILAR method: Influence of silver-doping on the morphological, structural and optical properties. Ceramics International. 2016; 42 (5):6010-6014
- 196. Ravichandran K, Banu NN, Baneto M, Selvi VS. Realizing near stoichiometric and highly transparent CdS: Mo thin films by a low-cost improved SILAR technique. Physica Status Solidi A: Applications and Materials Science. 2016; 213 (2):436-442
- 197. Ravichandran K, Nisha Banu N, Senthamil Selvi V, Rajkumar PV. Realization of near stoichiometric Sn-doped CdS films through an improved SILAR Technique. Materials Research Innovations. 2015:1-6
- 198. Gülen Y. Characteristics of Ba-Doped PbS Thin Films Prepared by the SILAR Method. Acta Physica Polonica A. 2014; 126 (03):763
- 199. Sundhar A. Development of ZnS thin film with Co, Cu and Ag doping using SILAR Method. Materials Today: Proceedings. 2022; 48 (2):377-381
- 200. Rajkumar PV, Ravichandran K, Baneto M, Ravidhas C, Sakthivel B, Dineshbabu N. Enhancement of optical and electrical properties of SILAR deposited ZnO thin films through fluorine doping and vacuum annealing for photovoltaic applications. Materials Science in Semiconductor Processing. 2015; 35 :189
- 201. K. Radhi Devi, G. Selvan, M. Karunakaran, K. Kasirajan, L. Bruno Chandrasekar, Mohd Shkir, S. AlFaify, SILAR-coated Mg-doped ZnO thin films for ammonia vapor sensing applications. Journal of Materials Science Materials in Electronics, 2020
© 2022 The Author(s). Licensee IntechOpen. This chapter is distributed under the terms of the Creative Commons Attribution 3.0 License , which permits unrestricted use, distribution, and reproduction in any medium, provided the original work is properly cited.
Continue reading from the same book
Published: 29 March 2023
By Mandla Msimanga
167 downloads
By Katherine Lochhead, Eric Johlin and Dongfang Yang
247 downloads
By Karampuri Yadagiri and Tao Wu
84 downloads
- Departments
- Supervisors
- Thesis Type
HfO2 thin films were prepared using reactive RF magnetron sputtering of a pure hafnium target in argon and oxygen ambient onto heavily doped p++ silicon (100) substrates. ZnO semiconducting thin film channel was deposited using sputtering of a pure metallic zinc target in oxygen ambient over the already deposited dielectric layer and the metallization for contact electrodes was done using thermal evaporation system. The thin film transistors (TFTs) were also fabricated with copper and aluminum as gate material other than heavily doped silicon. Besides TFT, metal-insulator-metal (MIM) and metal-oxide-semiconductor (MOS) structures were also made using HfO2 as oxide dielectric layer. The dielectric layer thickness, along with various growth parameters were studied and optimized using the various electrical characterization results from MOS, MIM and FET devices, to know the limit of oxide thickness that can provide sufficient charge polarization and electric field for channel conduction at lower gate voltages while giving low leakage current for the successful operation of the field-effect transistor (FET) device.
Repository Staff Only: item control page
Thank you for visiting nature.com. You are using a browser version with limited support for CSS. To obtain the best experience, we recommend you use a more up to date browser (or turn off compatibility mode in Internet Explorer). In the meantime, to ensure continued support, we are displaying the site without styles and JavaScript.
- View all journals
- My Account Login
- Explore content
- About the journal
- Publish with us
- Sign up for alerts
- Open access
- Published: 05 June 2023
Monolithically-stacked thin-film solid-state batteries
- Moritz H. Futscher ORCID: orcid.org/0000-0001-8451-5009 1 na1 ,
- Luc Brinkman 1 na1 ,
- André Müller ORCID: orcid.org/0000-0003-2275-8034 1 ,
- Joel Casella ORCID: orcid.org/0000-0002-2098-2983 1 ,
- Abdessalem Aribia 1 &
- Yaroslav E. Romanyuk ORCID: orcid.org/0000-0002-0529-228X 1
Communications Chemistry volume 6 , Article number: 110 ( 2023 ) Cite this article
4620 Accesses
3 Citations
57 Altmetric
Metrics details
The power capability of Li-ion batteries has become increasingly limiting for the electrification of transport on land and in the air. The specific power of Li-ion batteries is restricted to a few thousand W kg −1 due to the required cathode thickness of a few tens of micrometers. We present a design of monolithically-stacked thin-film cells that has the potential to increase the power ten-fold. We demonstrate an experimental proof-of-concept consisting of two monolithically stacked thin-film cells. Each cell consists of a silicon anode, a solid-oxide electrolyte, and a lithium cobalt oxide cathode. The battery can be cycled for more than 300 cycles between 6 and 8 V. Using a thermo-electric model, we predict that stacked thin-film batteries can achieve specific energies >250 Wh kg −1 at C-rates above 60, resulting in a specific power of tens of kW kg −1 needed for high-end applications such as drones, robots, and electric vertical take-off and landing aircrafts.
Similar content being viewed by others
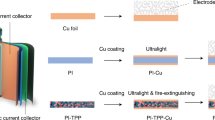
Ultralight and fire-extinguishing current collectors for high-energy and high-safety lithium-ion batteries
Yusheng Ye, Lien-Yang Chou, … Yi Cui
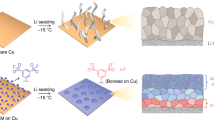
Low-temperature and high-rate-charging lithium metal batteries enabled by an electrochemically active monolayer-regulated interface
Yue Gao, Tomas Rojas, … Donghai Wang
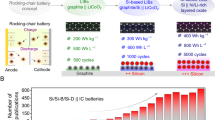
Production of high-energy Li-ion batteries comprising silicon-containing anodes and insertion-type cathodes
Gebrekidan Gebresilassie Eshetu, Heng Zhang, … Egbert Figgemeier
Introduction
Improving the performance of electrochemical energy storage devices is critical for the electrification of transport on a large scale. The specific energy of Li-ion batteries has improved greatly in recent decades, with record values >500 Wh kg −1 —close to predicted specific energy limits 1 , 2 , 3 , 4 . These batteries employ organic liquid electrolytes and composite cathodes with active electrode particles of ten micrometers in diameter. While such composite cathodes can provide high energies, the long diffusion path of Li ions within the composite cathode limits their rate capability, and thus their specific power 5 with record values up to 2.6 kW kg −1 6 . In addition, capacity fading and Li dendrite growth at high rates is an ongoing challenge and poses a significant limitation and safety risk when combined with flammable organic liquid electrolytes 7 , 8 , 9 .
Solid-state batteries (SSBs) have attracted great interest for their ability to increase safety at high charging/discharging rates 10 . However, the specific energy and power of SSBs lag behind that of conventional Li-ion batteries because current solid separators are significantly thicker than the separators used in Li-ion batteries with organic liquid electrolytes 11 , 12 .
Vacuum-based methods enable the fabrication of thin-film SSBs with electrode thicknesses on the order of micrometers in combination with (sub)micrometers-thick separators and current collectors 13 , 14 . In contrast to wet-chemical methods, solid-state thin films prepared by vacuum-based methods such as sputtering are dense and homogeneous and offer precise control of film thickness and composition 15 . Due to the short diffusion paths, thin-film SSBs offer more than ten times higher charging/discharging rates than composite cathodes 16 , 17 , enabling high powers yet with limited energy due to low areal capacities.
This work presents how a monolithic stack of thin-film cells can enable SSBs with both high energy and power. We demonstrate a prototype of a monolithically (bipolar) stacked thin-film battery with two cells electrically connected in series. Moreover, we predict the specific energy and power of monolithic stacked thin-film batteries using a thermo-electric model. We show that monolithically stacked batteries can potentially achieve specific energies >250 Wh kg −1 at charge/discharge times of less than 1 min, resulting in high specific powers of tens of kW kg −1 . These proposed batteries thus close the gap between supercapacitors and Li-ion batteries and facilitate the electrification of high-end applications such as drones, robots, and electric vertical take-off and landing aircraft (eVTOLs).
Stacked thin-film batteries
All-solid-state thin-film battery cells consist of a vacuum-processed cathode, solid electrolyte, and Li-metal anode, as illustrated in Fig. 1a . The most commonly used solid electrolyte in thin-film cells is Lipon, enabling Li-metal anodes and high-voltage cathodes due to its wide electrochemical stability window from 0 to 5 V vs. Li/Li + 18 . Thin-film cells using Lipon were shown to cycle for more than 10,000 cycles with a remarkably low degradation (<10%) 19 —a performance that has not been matched by any other SBB to date. In addition, thin-film cells offer fast charging (<1 min), are non-flammable, have excellent temperature stability suitable for temperature ranges between −40 and 150 °C, and have a meager self-discharge rate of <1% per year 13 . However, thin-film cells have a low areal capacity and are therefore limited to applications with low energy requirements, such as smart cards, medical devices, and small sensors for internet-of-things applications 20 .
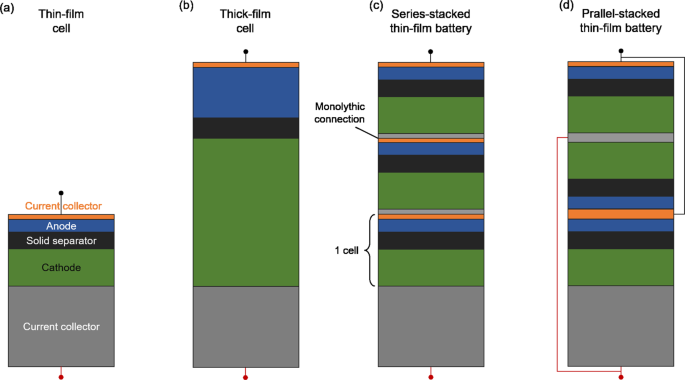
a Thin-film cell consisting of a cathode current collector, cathode, solid electrolyte, anode, and anode current collector. b A cell with a thick cathode and anode that are separated by a thin-film solid electrolyte. c Series-stacked thin-film battery, whereby several thin-film cells are monolithically stacked and electrically connected in series. d Parallel-stacked thin-film battery, whereby several thin-film cells are monolithically stacked and electrically connected in parallel.
To improve the energy of vacuum-deposited batteries, one can increase the cathode (and anode) thickness to a few tens of μm (Fig. 1b ). However, as the cathode’s thickness increases, the cell’s current and resistance increase, reducing the power due to energy lost as heat during cycling 21 , 22 . For cathode thicknesses greater than a few tens of μm, additives in the cathode are required to ensure sufficient ionic and electronic conductivity, decreasing the cathode’s active-to-passive mass ratio.
To increase both the energy and power of vacuum-processed batteries, one can stack several cells on top of each other on a single substrate to form a battery. Monolithic stacking enables the fabrication of stacked thin-film batteries, separated only by thin vacuum-deposited current collectors. The individual cells can be electrically connected in series or parallel (Fig. 1c, d ). When the cells are connected in series, the voltages of the stacked cells add up, while the capacity is limited by the cell with the lowest capacity. Such series-connected cells are often referred to as bipolar batteries. When the cells are connected in parallel, the capacities of the cells add up while the voltage is limited by the cell with the lowest voltage. Depending on the application, a high voltage (connected in series) or a high capacity (connected in parallel) design may be advantageous.
Table 1 compares the characteristics of the different vacuum-deposited device configurations. Cells with thick cathodes are suitable for high-energy cells but have a limited rate capability. In contrast, stacked thin-film batteries exhibit low heat losses, resulting in a good rate capability that enables increased power with reduced thermal management requirements. Although stacked thin-film batteries connected in series and parallel have different voltages and capacities, the usable energy and power are the same. Nevertheless, the different connection schemes have implications for the design and operation of the cells. For example, a high battery voltage helps to reduce cable power losses. The series connection further allows for a simpler cell design by eliminating the need for external connections such as tabs and wires. Differences also exist when a cell develops a shunt, e.g., by forming a dendrite. In the case of the parallel-stacked thin-film battery, a shunt in one cell can significantly reduce the effective output voltage. This is in contrast to a series-stacked thin-film battery, where a shunt in one of the cells would reduce the cumulative voltage of the battery. The failure of a cell within the battery stack is thus easier to identify in the series-stacked battery compared to the parallel-stacked battery. In addition, the current flow through the short-circuited cell will be higher in the case of a parallel-connected battery pack, which can locally heat the cell and potentially lead to accelerated degradation of the battery.
We note that stacking cells is a concept also used in conventional SSBs to simplify cell design by reducing external connections and cooling system requirements 23 , 24 , 25 . While bipolar stacked SSBs promise a 30% increase in specific energy and power 26 , their rate capability would still be limited by the long diffusion paths within the electrodes. Stacked thin-film batteries have further been discussed in patents 27 , 28 and previously attempted to be commercialized by a company called Sakti3 29 . However, to our knowledge, there has been no reported demonstration of a working stacked thin-film battery within both patents and academic literature, except for a study published in 2003 which demonstrated only one cycle at a C-rate of C/3 30 . Our work demonstrates thin-film batteries over many cycles with effective C-rates up to 60.
Experimental proof of concept
To demonstrate an experimental proof-of-concept of a monolithically-stacked device, we fabricated a (bipolar) stacked thin-film battery consisting of two cells electrically connected in series. Each cell consists of an Al cathode current collector, an amorphous LiCoO 2 (LCO) cathode, a Lipon solid electrolyte, a Si anode, and a Cu anode current collector, as illustrated in Fig. 2a . Amorphous LCO was chosen as the model cathode for this proof-of-concept because it requires no high-temperature post-crystallization steps that could otherwise damage the anode and electrolyte. Since monolithic stacking requires a smooth interface between two adjacent cells, we use a Si anode instead of a Li-metal anode, which is often inhomogeneous when deposited via vacuum-based methods. The inhomogeneous deposition of Li metal can lead to contact between the cathode of cell 2 and the anode of cell 1, resulting in battery failure. In contrast, the Si anode is deposited homogeneously, allowing a smooth interface between the two adjacent cells, as seen in the SEM cross-section in Fig. 2b . Figure 2c shows a top view of four series-stacked thin-film batteries. See Supplementary Note 1 , Fig. S 1 , and Fig. S 2 for individual electrode performance data, while Supplementary Fig. S 3 presents XRD measurements.
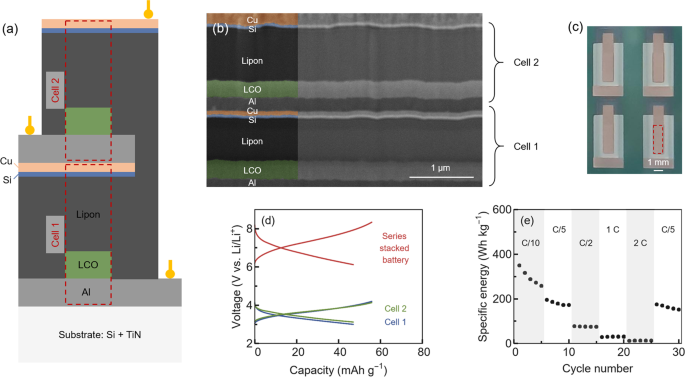
a Schematic illustration of the fabricated series-stacked thin-film battery. The two cells are marked by red dashed boxes. b FIB-SEM cross-section of a monolithically stacked thin-film battery connected in series. The two cells are separated only by thin current collectors, with the cathode current collector of cell 2 directly deposited on the anode current collector of cell 1. c Picture of four series-stacked thin-film batteries. The battery area (1 × 3) mm is marked by a red dashed box. d Charge–discharge curves of a series-stacked thin-film battery measured at C /10 (1 μA cm −2 ). The voltage of the series-stacked battery is the combined voltage of the two individual cells, which were cycled simultaneously. e The discharge energy of the series-stacked thin-film battery was measured at C -rates ranging from C /10 (1 μA cm −2 ) to 2 C (20 μA cm −2 ).
The voltage versus capacity curves in Fig. 2d show the charge and discharge behavior of the series-stacked thin-film battery. The voltage of the whole battery corresponds to the sum of the voltages of the two cells, as expected for an electrical series connection of the cells. The average charge and discharge voltages are 7.39 and 6.74 V, respectively. Prior to testing, the cells were precycled individually (see Supplementary Note 2 and Fig. S 4 ). During charging, current flowed through the entire cell stack, increasing the voltage of both cells. The voltages of cells 1 and 2 were measured independently, and the cycling process was controlled to keep both cells between 3.0 and 4.2 V. Cell 1 had a slightly lower capacity than cell 2. Therefore, cell 1 was the first to reach 4.2 V, while cell 2 remained at a lower state of charge and did not reach 4.2 V. This is more evident at higher C -rates (see Supplementary Fig. S 5 ). Figure 2e shows the discharge energy of the series-stacked thin-film battery as a function of the C -rate, from C /10 to 2 C . The prolonged cycling of the series-stacked thin-film battery for over 300 cycles is shown in Supplementary Fig. S 6 in the SI. While the battery’s specific energy degrades due to side reactions that reduce the capacity, the battery could operate up to C -rates of 2 and for hundreds of cycles without failure. We expect the amorphous LCO to be the limiting factor in the observed cycle life (see also Supplementary Note 1 ). Supplementary Fig. S 7 further shows the discharge capacity of three individual batteries along with their coulombic efficiencies.
The potential of stacked thin-film batteries
To further predict the performance potential of stacked thin-film batteries, we used a lumped steady-state thermo-electric model. The model calculates stacked thin-film batteries’ specific energy and rate capability, considering the operating limits imposed by voltage efficiency, critical current density, and thermal constraints. The model’s concept and basic working mechanism are illustrated in Fig. 3a and described in detail in Supplementary Method 1. The model assumes ten monolithically stacked cells on an Al substrate and lumps the electrochemical behavior of the individual cells into a constant voltage source in series with resistances that represent the ionic and electronic conductivity of the different material layers. The thermal behavior is modeled by Joule heating and 1D steady-state heat conduction (see Supplementary Fig. 8 ). We assumed anode-free cells, where the Li-metal anode is formed from the Li present in the cathode during the charging of the cell. Such an anode-free thin-film cell has already been achieved using Lipon as the solid electrolyte with critical current densities of up to 5 mA cm −2 31 , which can be further increased up to 8 mA cm −2 with thin carbon interlayers that are only a few tens of nanometers thick 32 .
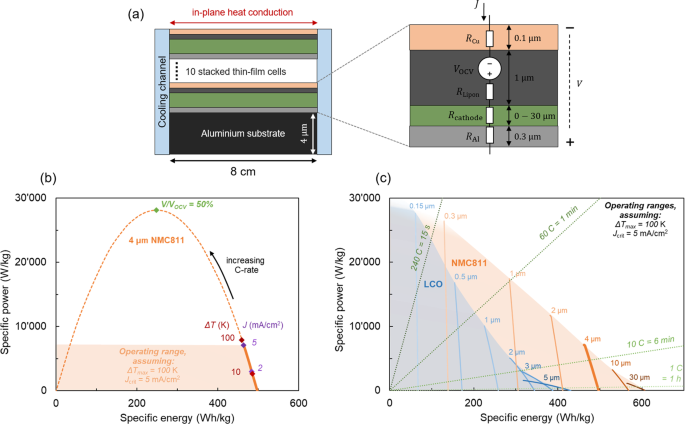
a Schematic illustration of the lumped steady-state thermo-electric model based on a series-stacked thin-film battery with the in-plane heat transfer to cooling channels on the side. Each anode-free cell consists of a 300 nm thick Al cathode current collector, a cathode with variable thickness, a 1 μm thick Lipon solid electrolyte, and a 100 nm thick Cu anode current collector. The substrate consists of a 4 μm thick Al foil. b Ragone plot of a simulated stacked thin-film battery, each cell having a 4 μm thick NMC811 cathode. The limiting factors imposed by voltage efficiency ( V/V OCV ), critical current density ( J ), and thermal constraints (Δ T ) are indicated. c The potential of stacked thin-film batteries is calculated for two different cathode materials—LCO and NMC811—for different cathode thicknesses, which are indicated at the respective lines. The green dashed lines indicate different charging and discharging rates. Note that the specific energies and powers given here are on a stack level, including substrates but not including cell casing.
Figure 3b shows the specific power of the battery as a function of its specific energy in a Ragone plot for the case of a 4 μm thick LiNi 0.8 Co 0.1 Mn 0.1 O 2 (NMC811) cathode per cell. Starting from high energy and low power, the power increases with increasing C -rate, while the energy decreases due to resistive losses. When the resistive losses lead to a voltage loss of 50%, the power reaches its maximum and decreases at higher C -rates (see Supplementary Fig. S 9 ). This decrease in power at high C -rates is universal for different battery material systems. Still, it is not usually shown in Ragone plots, as this is not a range of interest for battery operation. The simulated battery consists of ten stacked cells. Further, increasing the number of cells above ten has diminishing returns toward increasing the specific energy (see Supplementary Fig. S 10 ).
In addition to voltage efficiency ( V ), the critical current density ( J ), and the maximum allowable temperature difference between the center of the cell and the cooling channel (Δ T ) are two other factors that limit the power of the cell. For the case of 4 μm NMC811 as cathode, these limiting factors are shown in Fig. 3b for a temperature difference of 10 and 100 K and critical current densities of 2 and 5 mA cm −2 . The limiting factor is different for different cathode thicknesses and materials, as shown in Supplementary Fig. S 11 .
Figure 3c shows the simulated potential of stacked thin-film batteries for two different cathode materials, LCO and NMC811, with variable thicknesses. For the operating range, we assume a limiting temperature difference of 100 K and a limiting critical current density of 5 mA cm −2 . For NMC811 cathodes with a thickness larger than 4 μm, specific energies above 500 Wh kg −1 are calculated, which coincides with the predicted specific energy limit for SSBs using intercalation cathodes 3 . To obtain high specific power, thin cathodes are required to decrease the resistance losses during fast discharging. Using thin cathodes with a thickness below 1 μm, stacked thin-film batteries can ultimately achieve high specific powers >10 kW kg −1 at C -rates greater than 60.
Benchmarking the performance
To relate the experimental proof-of-concept results and the simulated potential of stacked thin-film batteries to published data on SSBs, we compare our results with literature data for all-SSBs in Fig. 4 11 . Note that our experimental values and literature data include only the anode, solid electrolyte, and cathode and exclude the current collectors and cell casing. The volumetric and gravimetric energy densities, including the current collectors, for three different cells are provided in Supplementary Fig. S 12 . In accordance with Randau et al. 11 , the target performance range of an SSB is specified with an energy of more than 250 Wh kg −1 and a cycle rate of more than 1 C, shown as a green-shaded area in Fig. 4 . Shown in yellow is the performance of a liquid electrolyte Li-ion battery (LG 18650 HG2L 3000 mAh) with one of the highest reported specific power values for commercially available batteries (including a current collector and cell housing) 33 . While we find that the performance of our series-stacked battery is on par with published results on SSBs, the performances of all the experimental data on SSBs are significantly lower than conventional Li-ion batteries.
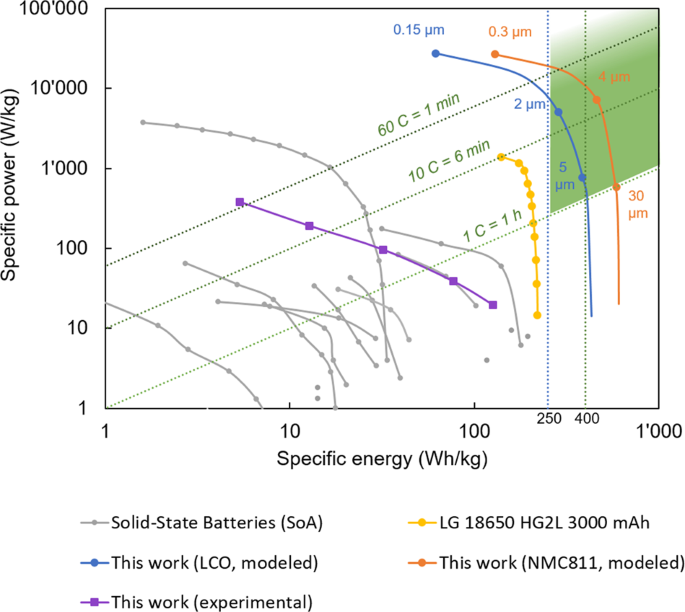
Ragone plot showing the performance of our series-stacked thin-film battery (purple), compared to published results of state-of-the-art (SoA) SSBs measured between 20 and 30 °C (gray) 11 . Our experimental results represent the mean of the values shown in Fig. 2e . Note that both data sets exclude the current collectors and cell casing. Further note that the C rates shown in Fig. 2e refer to initial capacity values under low discharge rates, while the C-rates in Fig. 4 correspond to effective discharge times. The yellow line shows the performance of a commercial lithium-ion battery for power tools, including current collectors and cell casing 33 . Blue and orange lines show the simulated potential of stacked thin-film batteries, including current collectors and substrates for LCO and NMC811 as cathode, respectively. The green shaded area shows the target performance for SSBs.
The calculated performance of stacked thin-film batteries using LCO and NMC811 cathode is shown in Fig. 4 as a solid blue and orange line, respectively (including current collectors and substrates but without cell casing). It is important to note that all-SSBs, such as the proposed stacked thin-film battery, have significantly lower packaging requirements than conventional Li-ion batteries because of the absence of liquid components. We find that stacked thin-film batteries have the potential to reach specific energies >250 Wh kg −1 at a C-rate of 10 C for both NMC811 and LCO and even >250 Wh kg −1 at a C -rate of 60 C for thin NMC811. In comparison, conventional SSBs with an NMC811 composite cathode thickness of more than 30 μm fail to achieve C rates above 10 C 22 —a distinct difference between stacked thin-film batteries and conventional SSBs.
Conclusions
We present a high-power and high-energy SSB design based on monolithically-stacked thin-film cells fabricated by scalable vacuum deposition. The individual cells can be electrically connected either in series or in parallel. Although the achievable energy and power of series- and parallel-stacked thin-film batteries are the same, the series-stacked thin-film batteries have some advantages over the parallel-stacked thin-film batteries due to lower currents and simplified cell design. We have experimentally demonstrated a proof-of-concept of a (bipolar) series-stacked thin-film battery. The performance of the fabricated stacked thin-film battery is on par with published results on SSB. We have further predicted the performance potential of stacked thin-film batteries using a thermo-electric model. Our model demonstrates that stacked thin-film batteries can reach specific energies >250 Wh kg −1 at C-rates greater than 60.
The unique differentiator of thin-film batteries over conventional SSBs is that the diffusion paths are short, which enables high C -rates and, thus, high power. While thick (composite) cathodes are well suited for applications requiring high energies, such as batteries for electric vehicles, stacked thin-film batteries with thin cathodes in the order of a few μm are well suited for applications requiring both high power and high energies, such as batteries for drones, robots, or eVTOLs that have stringent requirements for battery performance 34 .
There is undoubtedly a long way to go before the full potential of stacked thin-film batteries is realized. Several innovations are required: (i) Depending on whether high energy or high power is desired, the cathode thickness must be increased to up to tens of μm; (ii) the cathode must be crystallized to maximize capacity but without degradation of the other layers. This may be achieved by rapid thermal annealing 35 , photonic annealing methods 36 , or the use of cathodes such as vanadates with low annealing temperatures 37 ; (iii) the number of stacked cells must ideally be increased up to 10; (iv) the development of stacked thin-film cells in an anode-free design, e.g., by thin seed layers of gold or carbon 32 , 38 ; (v) the fabrication of stacked thin-film batteries on thin substrates, such as thin metal foils 39 , 40 ; (vi) the use of a bipolar current collector to avoid eventual formation of galvanic corrosion between the two metals during cycling.
Open questions remain as to how cycling-related volume changes affect such stacked thin-film batteries, especially when the number of cells increases, and how the vacuum-based deposition methods can be scaled to make stacked thin-film batteries economically viable. The fabrication costs for multi-cell thin-film batteries are expected to be higher than for conventional batteries, as vacuum coating is more expensive in terms of material volume than a slurry coating or printing. Alternative physical vapor deposition processes such as arc deposition and plasma- or thermal spraying can be explored to increase volumetric deposition rates reducing fabrication costs.
Fabrication
Si wafers with a 110 orientation and a thickness of 525 μm from University wafers were cleaned by ultra-sonication for 15 min, subsequently in detergent in deionized water, deionized water, acetone, and isopropanol. Subsequently, TiN with a thickness of 50 nm was deposited as an adhesion layer using a CT200 magnetron sputtering cluster (Alliance Concept) at a temperature of 450 °C by DC magnetron sputtering of a 25 cm target of Ti at a gas flow of 120 sccm Ar and 10 sccm N 2, a power of 3.1 W cm −2 , and a working pressure of 3 mTorr. All following depositions were performed either by a Nexdep evaporator (Angstrom Engineering Inc.) for thermal evaporation or with an Orion sputtering system (AJA International Inc.) for DC and RF sputtering. First, the cathode current collector Al with a thickness of 300 nm was deposited by thermal evaporation at a rate of 1 Å s −1 . Next, the LCO cathode with a thickness of 300 nm was deposited at room temperature through a shadow mask (3 × 1 mm) by RF magnetron sputtering of a 2” targets of Li 2 CO 2 at a gas flow of 24 sccm Ar and 1 sccm O 2 , a power of 15.3 W cm −2 , and a working pressure of 3 mTorr. Assuming a density of 4.79 g cm −3 , this corresponds to an areal mass loading of 0.1437 mg cm −2 per cell. The solid electrolyte Lipon with a thickness of 800 nm was deposited at room temperature by RF magnetron sputtering using sputtering of 2” targets of Li 3 PO 4 at a gas flow of 50 sccm N 2 , a power of 5.1 W cm −2 , and a working pressure of 3 mTorr. The Si anode with a thickness of 50 nm was deposited through a shadow mask (5.5 × 3 mm) at room temperature by DC magnetron sputtering using sputtering of 2” targets of Si at a gas flow of 24 sccm Ar, a power of 3.0 W cm −2 , and a working pressure of 4 mTorr. To finish the first cell, the anode current collector Cu with a thickness of 100 nm was deposited through a shadow mask (5.5 × 3 mm) by thermal evaporation at a rate of 1 Å s −1 . Subsequently, to deposit the second cell on top of the first cell, Al, LCO, Lipon, Si, and Cu were deposited as described above through different shadow masks (Al: 5.5 × 3 mm, LCO: 3 × 1 mm, Lipon: 6 × 2 mm, Si and Cu: 5 × 1 mm).
Characterization
Cross-section SEM images were obtained with a Helios NanoLab 600 DualBeam system. Transfer to the FIB-SEM was performed in air with an ambient time of less than 30 seconds. The cross-section was milled with an ion beam current of 0.77 nA, followed by a cleaning cut with an ion beam current of 80 pA at 30 kV. Electrochemical characterization was performed in an Ar-filled glovebox at room temperature without applied pressure using a Squidstat potentiostat (Admiral Instruments). The reported capacities correspond to electrode-level capacities. The theoretical capacity for amorphous LCO used to calculate the C -rate was assumed to be 70 mAh g −1 .
The performance potential of stacked thin-film SSBs was modeled using a lumped steady-state thermo-electric model, the details of which are described in Supplementary Method 1 .
Data availability
The data that support the findings of this study are available from the corresponding author upon reasonable request.
Han, B. et al. 500 Wh kg−1 class Li metal battery enabled by a self‐organized core–shell composite anode. Adv. Mater. 32 , 2004793 (2020).
Article CAS Google Scholar
Qiao, Y., Deng, H., He, P. & Zhou, H. A 500 Wh/kg lithium-metal cell based on anionic redox. Joule 4 , 1445–1458 (2020).
Whittingham, M. S. Ultimate limits to intercalation reactions for lithium batteries. Chem. Rev. 114 , 11414–11443 (2014).
Article CAS PubMed Google Scholar
Liu, J. et al. Pathways for practical high-energy long-cycling lithium metal batteries. Nat. Energy 4 , 180–186 (2019).
Lain, M. J. & Kendrick, E. Understanding the limitations of lithium ion batteries at high rates. J. Power Sources 493 , 229690 (2021).
Lain, B. & Kendrick Design strategies for high power vs. high energy lithium ion cells. Batteries 5 , 64 (2019).
Aurbach, D. A short review of failure mechanisms of lithium metal and lithiated graphite anodes in liquid electrolyte solutions. Solid State Ion. 148 , 405–416 (2002).
Lin, D., Liu, Y. & Cui, Y. Reviving the lithium metal anode for high-energy batteries. Nat. Nanotechnol. 12 , 194–206 (2017).
Wood, K. N. et al. Dendrites and pits: untangling the complex behavior of lithium metal anodes through operando video microscopy. ACS Cent. Sci. 2 , 790–801 (2016).
Article CAS PubMed PubMed Central Google Scholar
Choi, J. W. & Aurbach, D. Promise and reality of post-lithium-ion batteries with high energy densities. Nat. Rev. Mater. 1 , 16013 (2016).
Randau, S. et al. Benchmarking the performance of all-solid-state lithium batteries. Nat. Energy 5 , 259–270 (2020).
Kravchyk, K. V., Okur, F. & Kovalenko, M. V. Break-even analysis of all-solid-state batteries with Li-garnet solid electrolytes. ACS Energy Lett. 6 , 2202–2207 (2021).
Dudney, J. N. Thin film micro-batteries. Electrochem. Soc. Interf. 17 , 44–48 (2008).
Lobe, S., Bauer, A., Uhlenbruck, S. & Fattakhova‐Rohlfing, D. Physical vapor deposition in solid‐state battery development: from materials to devices. Adv. Sci. 8 , 2002044 (2021).
Balaish, M. et al. Processing thin but robust electrolytes for solid-state batteries. Nat. Energy 6 , 227–239 (2021).
Aribia, A. et al. In situ lithiated ALD niobium oxide for improved long term cycling of layered oxide cathodes: a thin-film model study. J. Electrochem. Soc. 168 , 040513 (2021).
Fehse, M. et al. Ultrafast dischargeable LiMn2O4 thin-film electrodes with pseudocapacitive properties for microbatteries. ACS Appl. Mater. Interfaces 9 , 5295–5301 (2017).
Bates, J., Dudney, N. J., Neudecker, B., Ueda, A. & Evans, C. D. Thin-film lithium and lithium-ion batteries. Solid State Ion. 135 , 33–45 (2000).
Li, J., Ma, C., Chi, M., Liang, C. & Dudney, N. J. Solid electrolyte: the key for high-voltage lithium batteries. Adv. Energy Mater. 5 , 1401408 (2015).
Article Google Scholar
Kyeremateng, N. A. & Hahn, R. Attainable energy density of microbatteries. ACS Energy Lett. 3 , 1172–1175 (2018).
Ioanniti, M. M., Hu, F. & Tenhaeff, W. E. Energy-dense Li metal anodes enabled by thin film electrolytes. J. Vac. Sci. Technol. A 38 , 060801 (2020).
Bielefeld, A., Weber, D. A. & Janek, J. Modeling effective ionic conductivity and binder influence in composite cathodes for all-solid-state batteries. ACS Appl. Mater. Interfaces 12 , 12821–12833 (2020).
Schnell, J. et al. All-solid-state lithium-ion and lithium metal batteries—paving the way to large-scale production. J. Power Sources 382 , 160–175 (2018).
Jung, K., Shin, H., Park, M. & Lee, J. Solid‐state lithium batteries: bipolar design, fabrication, and electrochemistry. ChemElectroChem 6 , 3842–3859 (2019).
Kim, J.-S. et al. Large area multi-stacked lithium-ion batteries for flexible and rollable applications. J. Mater. Chem. A 2 , 10862–10868 (2014).
Liu, T., Yuan, Y., Tao, X., Lin, Z. & Lu, J. Bipolar electrodes for next‐generation rechargeable batteries. Adv. Sci. 7 , 2001207 (2020).
Reynolds, G. J. & Mamazza, R. Lithium ion battery and method for manufacturing of such battery. US20120270114A1 (2010).
Wang, C.-W. et al. Monolithically integrated thin-film solid state lithium battery device having multiple layers of lithium electrochemical cells. US20120058380A1 (2011).
LeVine S. Sakti3’s quest for a better battery: hype, funding, promises, and then a surprise sale. https://qz.com/524268/sakti3s-quest-for-a-better-battery-hype-funding-promises-and-then-a-surprise-sale (2015).
Baba, M. et al. Multi-layered Li-ion rechargeable batteries for a high-voltage and high-current solid-state power source. J. Power Sources 119–121 , 914–917 (2003).
Neudecker, B. J., Dudney, N. J. & Bates, J. B. “Lithium-free” thin-film battery with in situ plated Li anode. J. Electrochem. Soc. 147 , 517 (2000).
Futscher, M. H. et al. Influence of amorphous carbon interlayers on nucleation and early growth of lithium metal at the current collector-solid electrolyte interface. J. Mater. Chem. A 10 , 15535–15542 (2022).
Battery review: LG 18650 HG2L 3000mAh. https://lygte-info.dk/review/batteries2012/LG 18650 HG2L 3000mAh (Cyan) UK.html.
Yang, X.-G., Liu, T., Ge, S., Rountree, E. & Wang, C.-Y. Challenges and key requirements of batteries for electric vertical takeoff and landing aircraft. Joule 5 , 1644–1659 (2021).
Song, S.-W. et al. High rate-induced structural changes in thin-film lithium batteries on flexible substrate. J. Power Sources 195 , 8275–8279 (2010).
Chen, X. et al. Photonic methods for rapid crystallization of LiMn2O4 cathodes for solid-state thin-film batteries. J. Power Sources 495 , 229424 (2021).
Ferrari, V. C., Kim, N. S., Lee, S. B., Rubloff, G. W. & Stewart, D. M. Co-sputtering of lithium vanadium oxide thin films with variable lithium content to enable advanced solid-state batteries. J. Mater. Chem. A 10 , 12518–12531 (2022).
Yan, K. et al. Selective deposition and stable encapsulation of lithium through heterogeneous seeded growth. Nat. Energy 1 , 16010 (2016).
Chen, X., Sastre, J., Aribia, A., Gilshtein, E. & Romanyuk, Y. E. Flash lamp annealing enables thin-film solid-state batteries on aluminum foil. ACS Appl. Energy Mater. 4 , 5408–5414 (2021).
Madinabeitia, I. et al. Monolithic all-solid-state high-voltage Li-metal thin-film rechargeable battery. ACS Appl. Energy Mater. 5 , 12120–12131 (2022).
Download references
Acknowledgements
The authors thank Ayodhya N. Tiwari and Maksym V. Kovalenko for carefully reading and commenting on the manuscript. The work is supported by the Strategic Focus Area (SFA) Advanced Manufacturing of the ETH Domain (project “SOL4BAT”) and the Swiss National Science Foundation (grant number 200021_172764). M.H.F. is supported by a Rubicon Fellowship from the Netherlands Organization for Scientific Research (NWO).
Author information
These authors contributed equally: Moritz H. Futscher, Luc Brinkman.
Authors and Affiliations
Laboratory for Thin Films and Photovoltaics, Empa—Swiss Federal Laboratories for Materials Science and Technology, Überlandstrasse 129, 8600, Dübendorf, Switzerland
Moritz H. Futscher, Luc Brinkman, André Müller, Joel Casella, Abdessalem Aribia & Yaroslav E. Romanyuk
You can also search for this author in PubMed Google Scholar
Contributions
M.H.F. conceived the experimental and theoretical work, designed and carried out experimental work, and prepared the paper; L.B. designed and carried out experimental and theoretical work and prepared the paper. A.M. carried out experimental work and performed FIB-SEM measurements. J.C. carried out experimental work and performed XRD measurements. A.A. assisted in thin-film fabrication and helped design experimental and theoretical work. Y.E.R. conceived and supervised the project. All authors reviewed and commented on the paper.
Corresponding authors
Correspondence to Moritz H. Futscher or Yaroslav E. Romanyuk .
Ethics declarations
Competing interests.
M.H.F., A.A., and Y.E.R. are founders of BTRY AG, a company commercializing solid-state batteries. The remaining authors declare no competing interests.
Peer review
Peer review information.
Communications Chemistry thanks Andrew S. Westover and the other anonymous reviewers for their contribution to the peer review of this work. A peer review file is available.
Additional information
Publisher’s note Springer Nature remains neutral with regard to jurisdictional claims in published maps and institutional affiliations.
Supplementary information
Peer review file, supplementary information, rights and permissions.
Open Access This article is licensed under a Creative Commons Attribution 4.0 International License, which permits use, sharing, adaptation, distribution and reproduction in any medium or format, as long as you give appropriate credit to the original author(s) and the source, provide a link to the Creative Commons license, and indicate if changes were made. The images or other third party material in this article are included in the article’s Creative Commons license, unless indicated otherwise in a credit line to the material. If material is not included in the article’s Creative Commons license and your intended use is not permitted by statutory regulation or exceeds the permitted use, you will need to obtain permission directly from the copyright holder. To view a copy of this license, visit http://creativecommons.org/licenses/by/4.0/ .
Reprints and permissions
About this article
Cite this article.
Futscher, M.H., Brinkman, L., Müller, A. et al. Monolithically-stacked thin-film solid-state batteries. Commun Chem 6 , 110 (2023). https://doi.org/10.1038/s42004-023-00901-w
Download citation
Received : 11 January 2023
Accepted : 11 May 2023
Published : 05 June 2023
DOI : https://doi.org/10.1038/s42004-023-00901-w
Share this article
Anyone you share the following link with will be able to read this content:
Sorry, a shareable link is not currently available for this article.
Provided by the Springer Nature SharedIt content-sharing initiative
By submitting a comment you agree to abide by our Terms and Community Guidelines . If you find something abusive or that does not comply with our terms or guidelines please flag it as inappropriate.
Quick links
- Explore articles by subject
- Guide to authors
- Editorial policies
Sign up for the Nature Briefing newsletter — what matters in science, free to your inbox daily.


Dalton Transactions
Strong thickness dependence in thin film photocatalytic heterojunctions: the zno–bi 2 o 3 case study †.

* Corresponding authors
a Instituto de Investigaciones en Materiales, Universidad Nacional Autónoma de México, Circuito Exterior S/N, Cd. Universitaria, Coyoacán, Mexico City, Mexico E-mail: [email protected]
Semiconductor heterojunctions are an effective way to achieve efficient photocatalysts, as they can provide an adequate redox potential with visible light excitation. Several works have reported synergistic effects with nanoparticle semiconductor materials. The question is still open for thin film heterojunctions formed by stacked layers, as photocatalysis is considered a surface phenomenon. To investigate if the internal layer really affects or modifies the photocatalytic properties of the external material, we analyze the thin film heterojunction with ZnO and Bi 2 O 3 semiconductors deposited by spray pyrolysis in two configurations: substrate/ZnO/Bi 2 O 3 and substrate/Bi 2 O 3 /ZnO. Microstructural analysis was performed to verify the formation of the physical junction of the materials and discard new ternary phases. The photocatalytic activity was analyzed as a function of the thickness of the layers under blue light irradiation. We determined the conduction and valence bands positions, the carrier concentrations, mobilities, Fermi levels, etc. that allowed us to distinguish two reaction mechanisms depending on the configuration. There is a strong compromise between the order and thickness of the layers with the photocatalytic activity. The internal electric field produced in the interface defines the route of the photogenerated charges, and therefore the photocatalytic response. Thus, well-designed thin film heterojunctions can indeed improve the photocatalytic activity of the surface layer.
Supplementary files
- Supplementary information PDF (142K)
Article information
Download citation, permissions.

Strong thickness dependence in thin film photocatalytic heterojunctions: the ZnO–Bi 2 O 3 case study
A. Bernal-Díaz, A. Hernández-Gordillo, J. C. Alonso, S. E. Rodil and M. Bizarro, Dalton Trans. , 2024, Advance Article , DOI: 10.1039/D4DT00697F
To request permission to reproduce material from this article, please go to the Copyright Clearance Center request page .
If you are an author contributing to an RSC publication, you do not need to request permission provided correct acknowledgement is given.
If you are the author of this article, you do not need to request permission to reproduce figures and diagrams provided correct acknowledgement is given. If you want to reproduce the whole article in a third-party publication (excluding your thesis/dissertation for which permission is not required) please go to the Copyright Clearance Center request page .
Read more about how to correctly acknowledge RSC content .
Social activity
Search articles by author.
This article has not yet been cited.
Advertisements
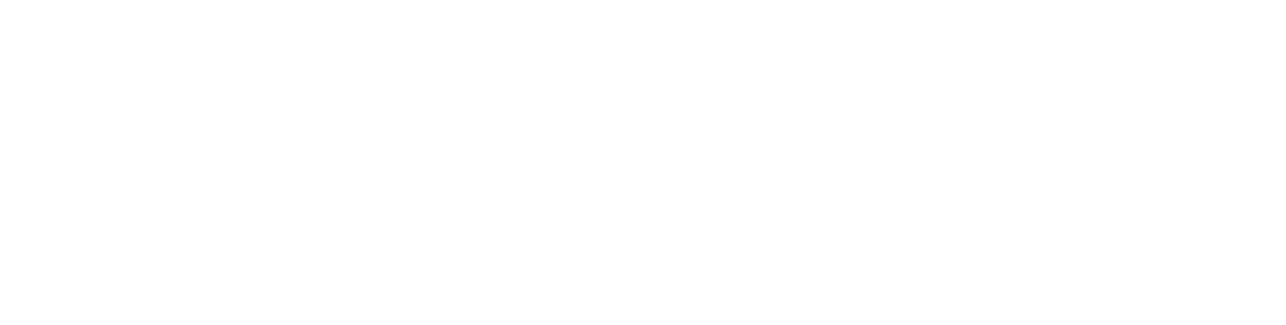
Search form
- Academic Advising & Support
- Academic Planning & Resources
- Environmental Changemakers Certificate
- Academic Opportunities & Research
- Financial Support
- Online Forms & Resources
- Calendar & Deadlines
- Career Resources
- Clubs and Organizations
- Frequently Asked Questions
- Advisors & Coordinators
- Graduate Studies Committee
- Curricular Practical Training
- New Graduate Students
- Ph.D. Milestones
- Policies & Procedures
- Teaching Assistant FAQs
- Career Development Resources
- Engineering Student Study/Meeting Space
- Free Software
- Message From Chair
- Facts & Figures
- Undergraduate
- Faculty & Staff
- Academic Employment
- Dept Events
- Discovery News
- Student Testimonials
- Give to BME
- Seminar Series
- E-Newsletter
- Message from Chair
- CEE Affiliates
- Give to CEE
- International Center Form
- MSE Business & Forms
- MSE 298 Seminars
- MSE Diversity & Inclusion
- Support MSE@UCI
- MAE Seminars
- Corporate Affiliates
- Interdisciplinary Graduate Programs
- All faculty & staff
- Dean's Office
- Development and External Relations
- Student Affairs
- Engineering Research Management
- UC Irvine Directory
- Proposal Text and Resources
- Early Career Opportunities
- Purchasing Requests
- Reimbursements
- Purchasing & Reimbursement Mission Statement
- Business Meetings/ Entertainment Guidelines
- Service Agreements
- Travel Guidelines
- Travel Tips
- UC Policies & Procedures
- Dean's Executive Office
- Chief Administrative Officers
- Personnel Unit
- Finance Unit
- Purchasing Unit
- Computing Unit
- Facilities Unit
- Curriculum, Analytical Studies, & Accreditation (CASA)
- Communications Office
- Development and External Relations Office
- Outreach Unit
- Office of Information Technology
- Faculty Websites
- Computer Labs & Laptops
- Engineering Facilities Request Form
- Safety Procedures
- Campus Evacuation Zones
- Environmental Health & Safety
- UCI Police Department
- Helpful Links
- At Your Service
- Zot! Portal
- FAQs for Engineering Instructors
- Spring Awards
- Process Improvement
- Alumni Spotlight
- Hall of Fame
- #ANTEATERENGINEER
- Ways to Give
- UCI Engineering Alumni Society
- UC Irvine Alumni Association
- Dean's Message
- Strategic Plan
- Facts and Figures
- Henry Samueli
- School Leadership
- Engineering Leadership Council
- Accreditation
- Orange County
- Got Questions?
- Enrollment and Degrees Awarded
- How to Apply
- Prospective Students
- Newly Admitted
- Majors and Minors Offered
- Programs and Concentrations
- Accelerated Status Program
- International Fellowships
- Meet Us on the Road
- Anteater Voices
- Ph.D. and Master's Inquiry Form
- Message from the Associate Dean
- UCI Engineering-LANL Graduate Fellowships
- Research Thrusts
- Research by Department
- Research Centers, Institutes and Facilities
- Undergraduate Research
- Interdisciplinary Science and Engineering Building (ISEB)
- Contracts & Grants / ERM
- Research and Proposal Development
- Annual Membership Levels
- Connect with Students
- Prototyping Services
- Sponsored Research
- External Relations Office
- Community College
- International
- IDEA - Inclusion, Diversity, Equity, Access
- Stacey Nicholas Office of Access and Inclusion
- Inclusion in Engineering Education
- Samueli Shoutouts
- Media Watch
- Dean's Report
- Social Media
- Style Guide
MRSEC/MSE Special Seminar: Designing New Electronic Phases Intransition-Metal Oxide Thin Films
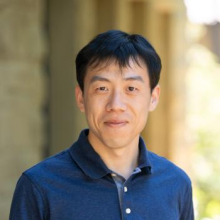
Abstract: The discovery of new quantum matters stays at the forefront of materials science and condensed matter physics, which offers the most promising way to solve the global energy and information issues. Thanks to the rapid development of epitaxial techniques, the synthesis of quantum materials can be precisely controlled at the atomic scale, opening new avenues for tailoring the device geometry and manipulating the functional properties. Although many external stimuli have been implemented to explore the exotic states in atomically thin films, the lack of microscopic understanding of the electronic structure at such extreme length scale has prevented us from fully unleashing the potential of epitaxial technology to engineer novel quantum materials. In this talk, I will show how to establish the connection between the macroscopic property and the microscopic structure in transition-metal oxides. By leveraging the power of thin film synthesis and advanced spectroscopy measurements, I explored the differentiated roles of Lifshitz transition on the thermodynamic and superconducting properties of La2-xSrxCuO4, as well as the underlying mechanism of metal-to-insulator transition in perovskite nickelate NdNiO3. This property-structure relationship will provide guiding rules for designing new electronic phases in transition-metal oxide films.
Bio: Zhong was born and raised in China. He completed his undergraduate degree at Nanjing University in 2012, and received his doctorate degree in physics from Tsinghua University in 2019. In his Ph.D. study, he combined molecular beam epitaxy and scanning tunneling microscopy techniques to investigate the exotic properties of topological materials and unconventional superconductors. Now he is a postdoctoral fellow at Stanford University. His current research uses synchrotron-based spectroscopic tools to elucidate the electronic structure of strongly correlated materials, particularly high-temperature cuprate superconductors and functional oxides.
Related Content
Upcoming events.
- 8 Apr MRSEC/MSE Special Seminar: Designing New Electronic Phases Intransition-Metal Oxide Thin Films
- 9 Apr MRSEC/MSE Special Seminar: Low-Dimensional Quantum Materials Design Through Atomically Precise Film Synthesis
- 11 Apr MSE 298 Seminar: Adaptive Materials Through Bioinspired Design and Additive Manufacturing
- 12 Apr EECS Seminar: Physical Layer Security for Dual-function Radar-Communication Systems
- 18 Apr MSE 298 Seminar: Catalyst Design For Clean Energy Technologies
News & Events
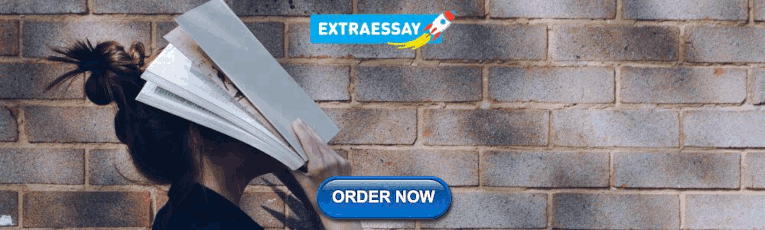
IMAGES
VIDEO
COMMENTS
Abstract. Since its discov ery in early time s, thin films rapidly found industrial applica-. tions such as in decorati ve an d optics purposes. W ith the evolution of thin film. technology ...
In this thesis, we present our works on engineering topology and correlation in two representative kagome metals, FeSn and Ni3In, stabilized in epitaxial thin film form. We characterize via transport, thermodynamic, and spectroscopic probes the emergent quantum phenomena arising from the key band structure singularities and further exploit ...
Deposition means a thin film coating process, which is achieved by modifying the . four states of matter i.e. Solid, Liquid, ... Thesis (M.S.)--Southwest Texas State University, 1995. Includes ...
This Licenciate Thesis is a part of my Ph.D. studies in the Thin Film Physics Group at the Department of Physics, Chemistry, and Biology (IFM) at Linköping University. The aim of my research is to synthesize and characterize MAX phases with novel properties. The work has been performed in cooperation with RWTH Aachen University (Germany),
The development of stress in thin films and multi-layers, monitored by in situ curvature measurements during vapor deposition in ultra-high vacuum, is then analyzed. 2. SURFACE AND INTERFACE STRESS 2.1. Theory The work, dW, required to create a new area dA of surface or interface is [7, 8] dW ‹g dA -1ƒ where g is the surface or ...
Abstract. Thin film technology is a major area of scientific research in the modern world because of its fascinating surface properties and wide range of applications from microelectronics to optics, space science to aircraft, and superconductivity to photovoltaic and solar cells. The performance of the thin films depends on the atomic ...
film and nanostructure growth. Importantly, for the thin film growth, chemical bath deposition (CBD) with various post-deposition annealing temperatures used to was grow porous thin films with different composition and degree of crystallinity; while sputtering with substrate heating used to deposit thin filmwass with excellent quality and
Thin film deposition is a technique for coating surfaces with very thin layers of material that range in thickness from a few nano meters to 100 µm, or just a few atoms. It can also be used to build up layers on top of already-deposited coatings. Today's semiconductor, photovoltaic, CD, disc drive, and optical device industries are all based ...
A thin film is a layer or multi-layer (a stack of thin films) of material ranging in thickness from nanometer to several micrometers, (Fig. 6). ... Thesis. Jul 2022; Christy Fadel; In this work ...
This chapter introduces the motivation behind the work presented in this thesis and the organization of the thesis chapters. Thin-film transistors (TFTs) for large-area electronics and in particular the stability requirement will be briefly reviewed. The stability issue is a common challenge that all new TFT technologies are facing.
In order to appreciate thin film device applications, it is essential to understand what thin films are, what makes them so attractive for applications, and how they are prepared and characterized. ... Ph.D. Thesis, Indian Institute of Technology, Delhi (1978). Google Scholar N. C. Sharma, R. C. Kainthla, D. K. Pandya, and K. L. Chopra, Thin ...
Numerous studies on the production of tin oxide thin films have been conducted, inclusive of Marikkannan et al.'s [] synthesis of thin films of metastable orthorhombic tin oxide on substrates of glass at ambient temperature through the utilization of the sol-gel approach for use in optoelectronic applications.They reported that the XRD patterns affirmed the entire films to be polycrystalline ...
This thesis demonstrates the comprehensive methods required for developing electrical recording devices and for analyzing the acquired neural data. It presents the design, fabrication, and in vivo implantation of flexible thin-film electronic ... thin-film device to the omnetics connector attached to the signal acquisition recording system ...
promise is thin-film superlattices, where each n-type and p-type film is composed of hundreds of thin alternating layers of two materials. In this work, we investigate n-type films composed of alternating silicon (Si) and silicon-carbide (SiC), and p-type films composed of alternating layers of two types of boron-carbide, B 4 C and B 9 C.
Since its discovery in early times, thin films rapidly found industrial applications such as in decorative and optics purposes. With the evolution of thin film technology, supported by the development of vacuum technology and electric power facilities, the range of applications has increased at a level that nowadays almost every industrial sector make use of them to provide specific physical ...
1. Introduction. The successive ionic layer adsorption and reaction (SILAR) technique was first introduced in 1985 by Nicolau for the deposition of ZnS and CdS [], and Ristov et al. for the deposition of Cu 2 O thin films [].Nicolau applied the adsorption technique of film preparation, relating two sources—a metal source of aqueous solutions of CdSO 4 or ZnSO 4, and a sulfide source of Na 2 ...
Abstract. Thin-film lithium niobate integrated photonics is a compelling platform for highly integrated electro-optic systems. The platform features compact, high-bandwidth phase modulators which can operate at CMOS compatible voltages, together with low optical loss, enabling photonic systems with large numbers of cascaded modulating elements.
Hu, Yaowen. 2023. Coupled-resonators on thin-film lithium niobate: Photonic multi-level system with electro-optic transition. Doctoral dissertation, Harvard University Graduate School of Arts and Sciences. ... In this thesis, we will discuss our implementation of photonic coupler-resonator . iv devices on thin -film lithium niobate. We ...
Seung-Yub Lee, 2003 Thesis title: "Synthesis and Characterization of Organically Modified Silicates Thin Film for Low Dielectric Constant Materials." Now at Caltech. Xi Zhang, 2004 Thesis title: "Electroless Ni metallization of macro-porous silicon for the application to cross-talk isolation in mixed signal integrated circuits." Now a Ph.
HfO2 thin films were prepared using reactive RF magnetron sputtering of a pure hafnium target in argon and oxygen ambient onto heavily doped p++ silicon (100) substrates. ZnO semiconducting thin film channel was deposited using sputtering of a pure metallic zinc target in oxygen ambient over the already deposited dielectric layer and the metallization for contact electrodes was done using ...
Here, the authors predict that stacked thin-film batteries with 0.15-2 µm thin cathodes can achieve a tenfold increase in specific power to over 10 kW kg−1 and demonstrate the design concept in ...
Thus, well-designed thin film heterojunctions can indeed improve the photocatalytic activity of the surface layer. About. Cited by. Related. Download ... If you want to reproduce the whole article in a third-party publication (excluding your thesis/dissertation for which permission is not required) please go to the ...
Abstract. The power output of thin film thermoelectric generators (TEG) is not sufficient for large scale applications and is mainly used for low power applications. A thin-film TEG for high-power ...
By leveraging the power of thin film synthesis and advanced spectroscopy measurements, I explored the differentiated roles of Lifshitz transition on the thermodynamic and superconducting properties of La2-xSrxCuO4, as well as the underlying mechanism of metal-to-insulator transition in perovskite nickelate NdNiO3.
Semantic Scholar extracted view of "Ultra high frequency thin film saw devices" by Juan Rodriguez Madrid. ... DOI: 10.20868/upm.thesis.15765; Corpus ID: 252827276; Ultra high frequency thin film saw devices @inproceedings{RodriguezMadridUltraHF, title={Ultra high frequency thin film saw devices}, author={Juan Rodriguez Madrid}, url={https://api ...