- To save this word, you'll need to log in. Log In
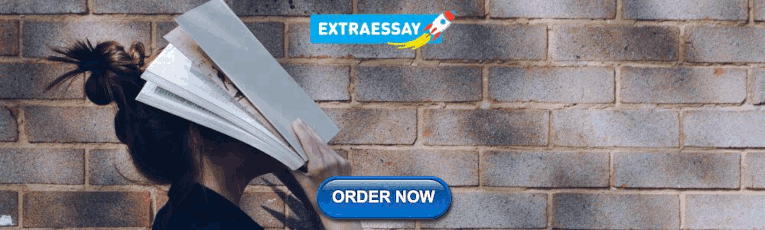
Definition of anthesis
Examples of anthesis in a sentence.
These examples are programmatically compiled from various online sources to illustrate current usage of the word 'anthesis.' Any opinions expressed in the examples do not represent those of Merriam-Webster or its editors. Send us feedback about these examples.
Word History
borrowed from New Latin, borrowed from Greek ánthēsis "blooming," from anthē-, variant stem of antheîn "to blossom, bloom" (verbal derivative of ánthos "flower") + -sis -sis — more at antho-
circa 1823, in the meaning defined above
Dictionary Entries Near anthesis
Cite this entry.
“Anthesis.” Merriam-Webster.com Dictionary , Merriam-Webster, https://www.merriam-webster.com/dictionary/anthesis. Accessed 21 Apr. 2024.
Subscribe to America's largest dictionary and get thousands more definitions and advanced search—ad free!
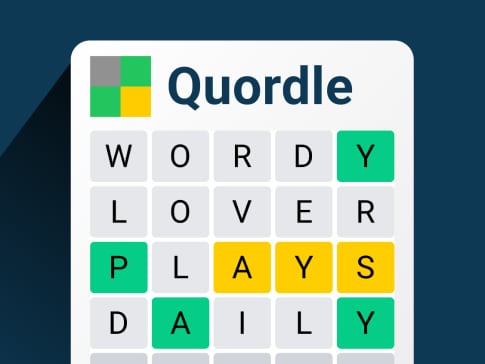
Can you solve 4 words at once?
Word of the day, noblesse oblige.
See Definitions and Examples »
Get Word of the Day daily email!
Popular in Grammar & Usage
Your vs. you're: how to use them correctly, every letter is silent, sometimes: a-z list of examples, more commonly mispronounced words, how to use em dashes (—), en dashes (–) , and hyphens (-), absent letters that are heard anyway, popular in wordplay, the words of the week - apr. 19, 10 words from taylor swift songs (merriam's version), a great big list of bread words, 10 scrabble words without any vowels, 12 more bird names that sound like insults (and sometimes are), games & quizzes.
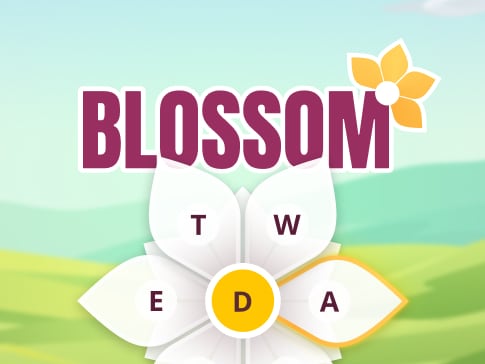
Learn (Aprender): Anthesis

Table of Contents
Introduction
Anthesis is a term commonly used in botany to describe a specific phase in the life cycle of a flower . It marks a significant period during which a flower is fully open and functional, allowing for pollination to occur.
Definition: What does Anthesis mean?
Anthesis is defined as the period during which a flower is fully open and the sexual organs are exposed , facilitating pollination. This is the time when the flower is most receptive to pollinators such as bees, birds, bats, or the wind .
Beginner Explanation
Imagine a flower like a tiny shop that needs to open its doors to let customers in. Anthesis is like the grand opening of that shop, when the flower opens up wide so that bees and other insects can come inside to visit. When they do, they help the flower by moving pollen from one flower to another, which is how new seeds are made.
Advanced Explanation
In botanical terms, anthesis encompasses the sequence of events that occur during the maturation of a flower, leading to its full expansion and the exposure of its reproductive structures, namely the stamens and pistils . This stage can vary in duration among different plant species , ranging from a few hours to several days. Anthesis is often synchronized with the diurnal or nocturnal activity patterns of the pollinators that a particular plant species relies on for successful reproduction . Environmental factors such as temperature, light , and humidity can influence the timing of anthesis, thereby affecting the flower’s reproductive success.
Historical Background
The term anthesis is derived from the Greek word anthos, meaning flower, and has been used in botanical literature to describe the flowering stage since at least the 18th century. The study of anthesis and its patterns has been important in the field of botany for understanding plant reproduction and the evolution of flowering plants.
Practical Application
For gardeners and farmers, understanding anthesis can be very important for the cultivation of both ornamental and crop plants. Knowing when a flower is at anthesis can help in planning the planting and care schedule to optimize pollination and, consequently, fruit and seed production. For instance, if a farmer knows that a particular crop flowers and reaches anthesis at dawn, they might plan to have pollinators like bees present at that time to ensure effective pollination. Similarly, in ornamental gardening , a gardener may time the planting of different species to ensure a continuous display of flowers at anthesis, creating an aesthetically pleasing garden with a succession of blooms.
Scientific Application
In scientific research, anthesis is a critical phase for studying plant reproduction, genetics, and breeding. By understanding the timing and conditions that trigger anthesis, scientists can develop strategies to enhance crop yields, create new varieties through selective breeding , and even protect endangered plant species by ensuring successful pollination and seed set in conservation programs.
Subscribe Now
Get FREE instant access to our eBook, "13 Mistakes Beginner Gardeners Make (And How To Avoid Them)". Enter your email below.
Download our FREE eBook "13 Mistakes Beginner Gardeners Make"
"13 Mistakes Beginner Gardeners Make (And How to Avoid Them)" Get it FREE - Enter your email below.
Disclaimer - Privacy Policy - Terms and Conditions
First days of anthesis: C. texana
First days of anthesis: c. carduacea, last days of anthesis: c. texana, last days of anthesis: c. carduacea.
postanthesis
- 1.1 Alternative forms
- 1.2 Etymology
- 1.3.1 Antonyms
English [ edit ]
Alternative forms [ edit ].
- post-anthesis
Etymology [ edit ]
From post- + anthesis .
Adjective [ edit ]
postanthesis ( not comparable )
- occurring after the opening of a flower
Antonyms [ edit ]
- preanthesis
- English terms prefixed with post-
- English lemmas
- English adjectives
- English uncomparable adjectives
Navigation menu

- Cambridge Dictionary +Plus
- Examples of anthesis
These examples are from corpora and from sources on the web. Any opinions in the examples do not represent the opinion of the Cambridge Dictionary editors or of Cambridge University Press or its licensors.
{{message}}
Please choose a part of speech and type your suggestion in the Definition field.
Help us improve the Cambridge Dictionary
anthesis doesn't have a definition yet. You can help!
Word of the Day
have irons in the fire
to be involved with many activities or jobs at the same time or to make certain that there are always several possibilities available

Binding, nailing, and gluing: talking about fastening things together

Learn more with +Plus
Thank you for suggesting a definition! Only you will see it until the Cambridge Dictionary team approves it, then other users will be able to see it and vote on it.
See your definition
- Recent and Recommended {{#preferredDictionaries}} {{name}} {{/preferredDictionaries}}
- Definitions Clear explanations of natural written and spoken English English Learner’s Dictionary Essential British English Essential American English
- Grammar and thesaurus Usage explanations of natural written and spoken English Grammar Thesaurus
- Pronunciation British and American pronunciations with audio English Pronunciation
- English–Chinese (Simplified) Chinese (Simplified)–English
- English–Chinese (Traditional) Chinese (Traditional)–English
- English–Dutch Dutch–English
- English–French French–English
- English–German German–English
- English–Indonesian Indonesian–English
- English–Italian Italian–English
- English–Japanese Japanese–English
- English–Norwegian Norwegian–English
- English–Polish Polish–English
- English–Portuguese Portuguese–English
- English–Spanish Spanish–English
- English–Swedish Swedish–English
- Dictionary +Plus Word Lists
There was a problem sending your report.
- Add a definition
- All translations
Words and phrases
Personal account.
- Access or purchase personal subscriptions
- Get our newsletter
- Save searches
- Set display preferences
Institutional access
Sign in with library card
Sign in with username / password
Recommend to your librarian
Institutional account management
Sign in as administrator on Oxford Academic
anthesis noun
- Hide all quotations
Earlier version
- anthesis in OED Second Edition (1989)
What does the noun anthesis mean?
There is one meaning in OED's entry for the noun anthesis . See ‘Meaning & use’ for definition, usage, and quotation evidence.
How common is the noun anthesis ?
How is the noun anthesis pronounced, british english, u.s. english, where does the noun anthesis come from.
Earliest known use
The earliest known use of the noun anthesis is in the late 1700s.
OED's earliest evidence for anthesis is from 1783, in C. Linnaeus' Syst. Veg.
anthesis is a borrowing from Latin.
Etymons: Latin anthesis .
Nearby entries
- antheridium, n. 1818–
- antheriferous, adj. 1799–
- antheriform, adj. 1802–
- antherine, n. 1689–
- antherless, adj. 1798–
- antherogenous, adj. 1847
- antheroid, adj. 1818–
- antherozoid, n. 1853–
- antherozoidal, adj. 1865–
- anther valve, n. 1839–
- anthesis, n. 1783–
- anthias, n. 1601–
- anthill, n. Old English–
- ant-hillock, n. 1656–
- ant-hilly, adj. 1796–
- anthine, n. & adj. 1601–1768
- ant-hive, n. 1817–
- antho-, comb. form
- anthobian, n. & adj. 1835–
- anthocarpous, adj. 1835–
- anthocephalous, adj. 1847
Meaning & use
The Anthesis [Latin Anthesis ] takes place, when the burnt Anthers scatter their bags of Dust upon the Stigma.
Bractea of the female flowers very much enlarged after anthesis , when the spike presents the appearance of a pine-apple; bright yellow, with red apices.
The term anthesis is sometimes used to indicate the period at which the flower-bud opens.
There were both delayed and extended antheses and most of the time the flowers were semi-open.
Histologically the ovary and style are relatively simple at anthesis .
From the time of anthesis , when the floral parts open to receive pollen, the developing grain becomes the dominant sink.
A later planting date reduced pre-anthesis moisture stress by reducing the number of days..for the crop to reach anthesis .
- efflorescence 1626– The process of producing flowers, or bursting into flower; the period of flowering.
- blow 1748– Manner, style, or time of blossoming. Also figurative .
- anthesis 1783– The stage at which a flower is open, allowing fertilization to occur. Also: an instance of this.
- florescence 1793– The process of producing flowers or bursting into flower; the period or state of flowering. Also concrete . Flowers collectively.
Pronunciation
Plural: antheses.
- ð th ee
- ɬ rhingy ll
Some consonants can take the function of the vowel in unstressed syllables. Where necessary, a syllabic marker diacritic is used, hence <petal> /ˈpɛtl/ but <petally> /ˈpɛtl̩i/.
- a trap, bath
- ɑː start, palm, bath
- ɔː thought, force
- ᵻ (/ɪ/-/ə/)
- ᵿ (/ʊ/-/ə/)
Other symbols
- The symbol ˈ at the beginning of a syllable indicates that that syllable is pronounced with primary stress.
- The symbol ˌ at the beginning of a syllable indicates that that syllable is pronounced with secondary stress.
- Round brackets ( ) in a transcription indicate that the symbol within the brackets is optional.
View the pronunciation model here .
* /d/ also represents a 'tapped' /t/ as in <bitter>
Some consonants can take the function of the vowel in unstressed syllables. Where necessary, a syllabic marker diacritic is used, hence <petal> /ˈpɛd(ə)l/ but <petally> /ˈpɛdl̩i/.
- i fleece, happ y
- æ trap, bath
- ɑ lot, palm, cloth, thought
- ɔ cloth, thought
- ɔr north, force
- ə strut, comm a
- ər nurse, lett er
- ɛ(ə)r square
- æ̃ sal on
Simple Text Respell
Simple text respell breaks words into syllables, separated by a hyphen. The syllable which carries the primary stress is written in capital letters. This key covers both British and U.S. English Simple Text Respell.
b, d, f, h, k, l, m, n, p, r, s, t, v, w and z have their standard English values
- arr carry (British only)
- a(ng) gratin
- o lot (British only)
- orr sorry (British only)
- o(ng) salon
Inflections
anthesis typically occurs about 0.2 times per million words in modern written English.
anthesis is in frequency band 4, which contains words occurring between 0.1 and 1 times per million words in modern written English. More about OED's frequency bands
Frequency of anthesis, n. , 1810–2010
* Occurrences per million words in written English
Historical frequency series are derived from Google Books Ngrams (version 2), a data set based on the Google Books corpus of several million books printed in English between 1500 and 2010.
The overall frequency for a given word is calculated by summing frequencies for the main form of the word, any plural or inflected forms, and any major spelling variations.
For sets of homographs (distinct entries that share the same word-form, e.g. mole , n.¹, mole , n.², mole , n.³, etc.), we have estimated the frequency of each homograph entry as a fraction of the total Ngrams frequency for the word-form. This may result in inaccuracies.
Smoothing has been applied to series for lower-frequency words, using a moving-average algorithm. This reduces short-term fluctuations, which may be produced by variability in the content of the Google Books corpus.
Frequency of anthesis, n. , 2017–2023
Modern frequency series are derived from a corpus of 20 billion words, covering the period from 2017 to the present. The corpus is mainly compiled from online news sources, and covers all major varieties of World English.
Smoothing has been applied to series for lower-frequency words, using a moving-average algorithm. This reduces short-term fluctuations, which may be produced by variability in the content of the corpus.
Compounds & derived words
- synanthesis , n. 1880– Simultaneous ripening of the stamens and pistils in a flower.
Entry history for anthesis, n.
anthesis, n. was revised in March 2016.
anthesis, n. was last modified in July 2023.
oed.com is a living text, updated every three months. Modifications may include:
- further revisions to definitions, pronunciation, etymology, headwords, variant spellings, quotations, and dates;
- new senses, phrases, and quotations.
Revisions and additions of this kind were last incorporated into anthesis, n. in July 2023.
Earlier versions of this entry were published in:
OED First Edition (1885)
- Find out more
OED Second Edition (1989)
- View anthesis in OED Second Edition
Please submit your feedback for anthesis, n.
Please include your email address if you are happy to be contacted about your feedback. OUP will not use this email address for any other purpose.
Citation details
Factsheet for anthesis, n., browse entry.
- Daily Crossword
- Word Puzzle
- Word Finder
Word of the Day
- Synonym of the Day
- Word of the Year
- Language stories
- All featured
- Gender and sexuality
- All pop culture
- Grammar Coach ™
- Writing hub
- Grammar essentials
- Commonly confused
- All writing tips
- Pop culture
- Writing tips
Advertisement
[ an- thee -sis ]
- the period or act of expansion in flowers, especially the maturing of the stamens.
/ ænˈθiːsɪs /
- the time when a flower becomes sexually functional
/ ăn-thē ′ sĭs /
- The period during which a flower is fully open and functional.
- Also called efflorescence
Discover More
Word history and origins.
Origin of anthesis 1
Example Sentences
These swellings help to spread out the branches especially at the time of anthesis.
Relation of temperature to anthesis and blossom drop of the tomato together with a histological study of the pistils.
Anthesis, the period or the act of the expansion of a flower.
These glands secrete a viscid juice at the time of anthesis.
Anthesis, anthropocosmic—— Say, I'm glad you didn't call me that!
[ ak -s uh -lot-l ]
Start each day with the Word of the Day in your inbox!
By clicking "Sign Up", you are accepting Dictionary.com Terms & Conditions and Privacy Policies.
- Open access
- Published: 04 May 2023
Nitrogen uptake and remobilization from pre- and post-anthesis stages contribute towards grain yield and grain protein concentration in wheat grown in limited nitrogen conditions
- Sandeep Sharma ORCID: orcid.org/0000-0002-5795-8899 1 ,
- Tarun Kumar 1 ,
- M. John Foulkes 2 ,
- Simon Orford 3 ,
- Anju Mahendru Singh 4 ,
- Luzie U. Wingen 3 ,
- Venkatesh Karnam 5 ,
- Lekshmy S. Nair 1 ,
- Pranab Kumar Mandal 6 ,
- Simon Griffiths 3 ,
- Malcolm J. Hawkesford 7 ,
- Peter R. Shewry 7 ,
- Alison R. Bentley 8 , 9 &
- Renu Pandey ORCID: orcid.org/0000-0002-9244-8579 1
CABI Agriculture and Bioscience volume 4 , Article number: 12 ( 2023 ) Cite this article
1843 Accesses
4 Citations
2 Altmetric
Metrics details
In wheat, nitrogen (N) remobilization from vegetative tissues to developing grains largely depends on genetic and environmental factors. The evaluation of genetic potential of crops under limited resource inputs such as limited N supply would provide an opportunity to identify N-efficient lines with improved N utilisation efficiency and yield potential. We assessed the genetic variation in wheat recombinant inbred lines (RILs) for uptake, partitioning, and remobilization of N towards grain, its association with grain protein concentration (GPC) and grain yield.
We used the nested association mapping (NAM) population (195 lines) derived by crossing Paragon (P) with CIMMYT core germplasm (P × Cim), Baj (P × Baj), Watkins (P × Wat), and Wyalkatchem (P × Wya). These lines were evaluated in the field for two seasons under limited N supply. The plant sampling was done at anthesis and physiological maturity stages. Various physiological traits were recorded and total N uptake and other N related indices were calculated. The grain protein deviation (GPD) was calculated from the regression of grain yield on GPC. These lines were grouped into different clusters by hierarchical cluster analysis based on grain yield and N-remobilization efficiency (NRE).
The genetic variation in accumulation of biomass at both pre- and post-anthesis stages were correlated with grain-yield. The NRE significantly correlated with aboveground N uptake at anthesis (AGNa) and grain yield but negatively associated with AGN at post-anthesis (AGNpa) suggesting higher N uptake till anthesis favours high N remobilization during grain filling. Hierarchical cluster analysis of these RILs based on NRE and yield resulted in four clusters, efficient (31), moderately efficient (59), moderately inefficient (58), and inefficient (47). In the N-efficient lines, AGNa contributed to 77% of total N accumulated in grains, while it was 63% in N-inefficient lines. Several N-efficient lines also exhibited positive grain protein deviation (GPD), combining high grain yield and GPC. Among crosses, the P × Cim were superior and N-efficient, while P × Wya responded poorly to low N input.
Conclusions
We propose that traits favouring pre- or post-anthesis biomass accumulation and pre-anthesis N uptake may be targeted for breeding to improve grain-yield under limited N. The lines with positive GPD, a first report of genotype-dependent GPD associated with both AGNpa and AGNa in wheat, may be used as varieties or genetic resources to improve grain yield with high GPC for sustainable development under limited N conditions.
Wheat ( Triticum aestivum L.) is the most widely grown staple food crop globally, providing a significant contribution to dietary calories, protein, and micronutrients (Shiferaw et al. 2020 ). Global wheat production was 765 million tons in 2019 (FAO 2021 ), while India produced an all-time high of 102 million tons in 2019 becoming the second-largest wheat producing country in the world (Tyagi et al. 2020 ). To obtain maximum yield potential, chemical fertilizer is one of the key inputs, with nitrogen (N) being the major macronutrient. Globally, India is the second largest consumer of nitrogenous fertilizers, with the current overall N fertilizer use of about 17 million tons, projected to grow to 24 million tons by 2030. Up to ~ 70% of N fertilizer applied in wheat fields is not utilized by the crop due to low N utilization efficiency (NUE ~ 33%), therefore excessive N application not only significantly contributes to economic loss but also to environmental problems (Abrol et al. 2012 ; Tyagi et al. 2020 ). The majority of N lost from crop production is through emission of ammonia and nitrous oxide (ca. 40%), surface runoff (ca. 13%), and leaching of nitrate (Zhang et al. 2017 ; Jiang et al. 2018 ). This has resulted in several environmental concerns including soil acidification, global warming, eutrophication of surface water systems, and pollution of ground water (Sun et al. 2020 ). It is therefore, necessary to identify wheat cultivars or genetic resources possessing superior N uptake and remobilization efficiency in combination with higher grain yield and grain protein concentration (GPC). These cultivars should also be “genetically N-efficient”, allowing maintenance of superior performance even in soils with limited N availability.
In wheat, most of the plant N, in some cases more than 90% of the total N at maturity, is assimilated at the pre-anthesis stage (Cox et al. 1985 , 1986 ). However, the pre-anthesis assimilation of N varies between genotypes and is also significantly influenced by environmental conditions (Papakosta and Garianas 1991 ; Hocking and Staper 2001 ). During the pre-anthesis stage, developing leaves and roots act as major ‘sinks’ for carbon (C) and N assimilates, while after anthesis, the developing grain becomes the most active sink (Fuertes‐Mendizábal et al. 2012 ). Most of the N accumulated up to anthesis is used for synthesis of structural and regulatory proteins while the remaining N is remobilized to the grains during later growth stages (Pask et al. 2012 ). The N-remobilization efficiency (NRE) is calculated as the fraction of N in the crop or crop components at anthesis which is not present in the crop or crop components at harvest (Gaju et al. 2014 ). Although the amount of available N in the soil significantly affects the grain yield, most of the selection in plant breeding is performed under non-limiting N conditions. Whilst recent work has shown that high yielding cultivars identified under non-limiting N perform well under N limitation (Voss-Fels et al. 2019 ), this is not always the case (Nehe et al. 2018 ). Ideally, an efficient genotype should exhibit high yield under non-limiting N conditions and maintain acceptable yields under low N.
In modern wheat cultivars (developed after 1960–70 s through cross-breeding or genetic manipulation), grown under favourable growth conditions, more than 50% of aboveground biomass is allocated to the grain i.e., the harvest index (HI) is more than 0.50 (Maeoka et al. 2020 ). About 85% of carbohydrates accumulated in grains are synthesized post-anthesis (Borrell et al. 1989 ), while most of the N present in grain is taken up by the plants in the pre-anthesis stages (Bertholdsson and Stoy 1995 ). It has been reported that 50 to 95% of the N present in the grain at harvest is remobilised from the leaves, stem, and sheath (Papakosta and Garianas 1991 ), while the chaff and root contribute only 15 and 10% of the total grain N, respectively (Dalling 1985 ). The amount of N remobilized to the grain is highly dependent on the N stored at anthesis and its mobilization to the grains is determined by the supply from the source (vegetative tissue), rather than the demand of N by the sink (developing grains) (Martre et al. 2003 ). Earlier reports have shown increased NRE under N limiting (Halloran 1981 ; Gaju et al. 2014 ) or water deficit conditions during grain filling (Palta et al. 1994 ) because stress restricts post-anthesis N uptake thereby, forcing the plant to remobilize stored N to the grains. Accelerated leaf senescence during grain filling reduced grain yield but it led to a higher N remobilization to the grains (Waters et al. 2009 ). However, in the spring wheat cultivars ‘ANZA’ and ‘CAJEME-71’, a higher dose of N fertilizer during the pre-anthesis phase increased the availability of N during grain filling, thereby reducing the redundant N storage reserves and leading to decrease in NRE (Cox et al. 1986 ).
The accumulation and remobilization of N are critical processes determining grain yield and quality. Although the remobilization of N to the grains is under genetic control, it is also influenced by soil N concentration and weather conditions which particularly determine the duration of grain filling (Fuertes‐Mendizábal et al. 2012 ). Wheat cultivars with enhanced yield components (larger spikes and a greater number of kernels) show high potential for N uptake and improved NRE resulting in high grain N content (Mi et al. 2000 ). The grain ‘sink-strength’ as set by the number of kernels and the potential grain size is the principal determinant of grain yield and consequently deficiency of N in the pre-anthesis phase causes a major reduction in yields (Jeuffroy and Bouchard 1999 ). Therefore, the development of cultivars with larger spikes and more grains under N limiting conditions may contribute to increasing grain yield. Moreover, increased root growth is associated with greater aboveground biomass because deeper roots and increased root density favour N uptake. A deeper root system can also potentially increase the stay-green effect which could lead to a higher grain yield (Andersson et al. 2005 ; Nehe et al. 2020 ).
Grain quality is strongly influenced by grain protein concentration (GPC) which in turn is directly affected by the rates of N fertilizer (De Santis et al. 2020 ). Furthermore, while high GPC in wheat can be attributed in part to high N application rates, wheat cultivars with high GPC under limited N must be selected to a large extent to improve wheat grain quality (Zörb et al. 2018 ). However, in most cereals, including wheat, a negative genetic relationship exists between grain yield and GPC, thereby making it difficult to improve both traits simultaneously (Slafer and Andrade et al. 1993 ; Simmonds 1995 ; Bogard et al. 2010 ). The selection of wheat cultivars with higher grain protein deviation (GPD) (the deviation from the regression line between GPC on grain yield) (Monaghan et al. 2001 ; Mosleth et al. 2020 ) and grain yield is therefore an attractive strategy to improve grain quality at high yields.
In the present study, we used 195 recombinant inbred lines (RILs) selected from a nested association mapping (NAM) population to assess variation for remobilization of pre- and post-anthesis biomass and N uptake under limited N conditions (Fig. 1 ). We aimed to understand the physiological basis of the interaction between remobilization, yield, and GPC. Another objective was to identify superior lines for use in breeding in northern Indian conditions as a source of variation for N use efficiency. These lines with superior agronomic traits would lead towards sustaining growth and yield with higher grain protein content in wheat in soils with low N availability.
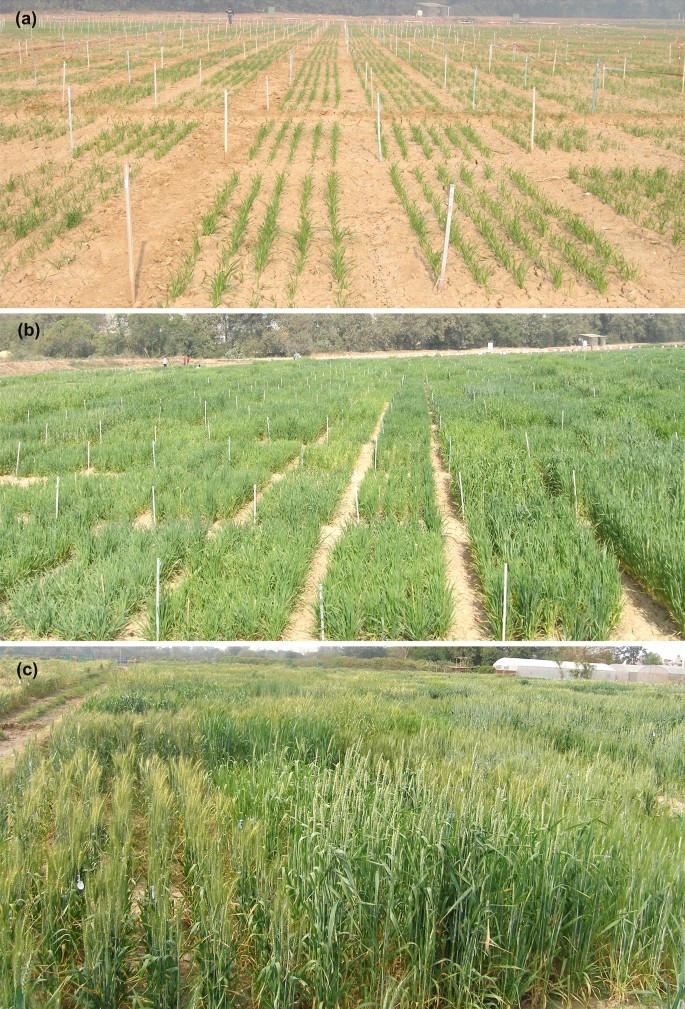
Field view of 195 wheat recombinant inbred lines at a GS23 stage (main shoot and 3 tiller), b GS33 stage (second node detectable), and c GS69 stage (flowering complete), derived from NAM population, grown with no external nitrogen application under north Indian condition. The genotypic variation in growth and canopy coverage can be clearly seen under limited N conditions
Materials and methods
Plant material and experimental site.
The material was selected from RILs derived from wheat NAM panels, all RILs sharing the common parent ‘Paragon’, a photoperiod sensitive UK spring wheat cultivar (Bentley et al. 2011 ) with broad-spectrum disease resistance (Amalova et al. 2019 ) and low Mn efficiency (Jiang 2008 ). Paragon was crossed with historical high-yielding cultivar, ‘CIMCOG’ (Cim), modern cultivars ‘Baj’ and ‘Wyalkatchem’ (Wya) and diverse landrace cultivars from the ‘Watkins’ collection (Wat). Baj is an early maturing high yielding CIMMYT spring wheat with no vernalisation requirement (Mondal et al. 2015 ). The ‘CIMCOG’ (CIMMYT CORE GERMPLASM) includes historical high-yielding durum wheat and advance hexaploid wheat cultivars developed by CIMMYT for improvement in photosynthesis and biomass (Gonzalez-Navarro et al. 2016 ). Wyalkatchem, a popular wheat line of Western Australia, possessing larger grain size with higher GPC as compared to other high yielding cultivars. It also has a high Hagberg falling number rating showing low susceptibility to pre-harvest sprouting (Zaicou-Kunesch et al. 2017 ). ‘Watkins’ represents the collections made by A. E. Watkins in 1930s from local markets of 32 countries comprising of considerable number of landrace cultivars with a large amount of genetic diversity (Wingen et al. 2014 ).
Out of total 195 lines, we selected 132 RILs from eighteen populations of the wheat landrace NAM panel (Wingen et al. 2017 ) and 63 RILs from populations with modern cultivars (Additional file 1 : Table S1). The seeds of these RILs were supplied by the John Innes Centre, Norwich under Newton-Bhabha Fund Project “Indo-UK Centre for the improvement of nitrogen use efficiency in wheat” (INEW) (Additional file 1 : Table S2). Field trials were conducted over two wheat growing seasons (2016–17 and 2017–18) in the experimental farm of ICAR-IARI, New Delhi, located at 28.08°N and 77.12°E, 228.6 m above sea level. Sowing was done in the winter season (2nd fortnight of November) and harvested during April/May. The preceding crop was rice in both years. Soil samples for nutrient analysis were taken at two depths, 0–30 and 30–60 cm, in both the years before sowing. The soil properties are presented in Additional file 1 : Table S3. The experiment was conducted in low N soil (175–198 kg N ha −1 ) with no external N application while the recommended dose of phosphorus (60 kg P 2 O 5 ha −1 ), and potash (40 kg K 2 O ha −1 ) were applied as basal dose through single super phosphate and muriate of potash, respectively. The design of the experiment was a randomized block design (RBD) with one variable (genotypes) and three replications. The net plot size was 3.5 m 2 (3.5 m × 1.0 m) with a seed rate of ~ 300 seeds m −2 . Six irrigations were applied during critical growth stages (GS) such as crown root initiation (CRI), tillering (GS25), booting (GS41), flowering (GS61), milk (GS73), and dough stages (GS85). Crops were protected from weeds, pests and diseases by applying herbicides and pesticides respectively as and when required.
Phenology and biomass sampling
Crop growth was monitored regularly and the dates of anthesis (GS65) and physiological maturity (GS91, grain hard and difficult to divide) were recorded following the Zadoks decimal code (Zadoks et al. 1974 ). At anthesis, samples for aboveground biomass accumulation were taken from each plot by harvesting shoots from 1.0 m 2 area and recording the fresh weight. A sub-sample from the harvested shoots was weighed fresh and after drying in a hot air oven at 65 °C to a constant weight. After calculating the moisture percentage in the shoots, the dry weight of 1.0 m 2 sample was calculated. Samples were harvested from 1.0 m 2 areas from each plot at maturity (GS92) and the total biomass was recorded after sun-drying. The shoot was then separated into parts, i.e., leaf, stem (true stem with leaf-sheath), and spike which were also weighed. The total biomass accumulated after anthesis was calculated by subtracting total aboveground biomass at anthesis (AGBa) from the total aboveground biomass at harvest (AGBh). For measuring yield, shoots were cut at ground level from the centre of each plot from one m −2 sub-plot area at physiological maturity stage (GS92) and after threshing, cleaning and moisture analysis of grains, the total grain weight m −2 and 1000-grain weight (TGW) were recorded.
Nitrogen estimation and calculation of N indices
For N% analysis, the main shoots were randomly selected from three different plants at anthesis and physiological maturity stage from each plot. The anthesis stage N content was determined using the whole shoot, while at maturity, the shoot was separated into the leaf lamina, stem (true stem with leaf-sheath) and grain. The N% was analysed by Dumas method using CHNS analyser (Eurovector EA3000, Italy) after milling dry samples into fine powder using a mixer-grinder. Post-anthesis N uptake (AGNpa) was calculated as the difference between aboveground N uptake at harvest (AGNh) and at anthesis (AGNa). N remobilization efficiency (NRE) was calculated as the amount of N taken up at anthesis (AGNa) that was remobilized:
Nitrogen Nutrition Index (NNI) was calculated at anthesis as the ratio of the aboveground N% to the critical N% at anthesis, where critical N% was estimated according to the ‘critical dilution curve’ described by Justes et al. ( 1994 ). The N harvest index (NHI) was calculated as the proportion of aboveground N in the grain while N Partitioning Index (NPI) at maturity was calculated in different plant components against the total aboveground N at harvest. The grain protein concentration (GPC) was calculated by multiplying the grain N% by the factor 5.7 (Sosulski and Imafidon 1990 ). Grain protein deviation (GPD) was calculated from the regression of grain yield on GPC to determine the extent of deviation among RILs with respect to grain yield and GPC (Monaghan et al. 2001 ).
Environmental measurements
Meteorological data were collected daily for minimum and maximum temperature, humidity, and rainfall from the meteorological observatory of IARI, Pusa Campus, New Delhi situated within 250 m from the experimental field site (Additional file 1 : Fig. S1).
Statistical analysis
The data were analysed by one-way analysis of variance (ANOVA) and the significant differences between different crosses were calculated by critical difference (CD) using MS-DOS based statistical software AGRES ver. 3.01 (Agres 1994 ). To quantify the association between traits, Pearson’s correlation coefficient and linear regression were calculated using MS Excel 2016. R (ver 3.6.1) package Factoextra was used to prepare bi-plot to test association between traits. For identification of low N stress tolerant lines, all 195 lines were clustered into different groups by hierarchical cluster analysis based on grain yield and NRE. Graphs were made using GraphPad Prism version 6.00 (GraphPad Software, La Jolla, CA).
The 195 lines of the NAM population were divided into four groups based on the parents used in the crosses viz . Paragon × CIMCOG (P × Cim, n = 32), Paragon × Baj (P × Baj, n = 16), Paragon × Watkins (P × Wat, n = 132), and Paragon × Wyalkatchem (P × Wya, n = 15). Growth and physiological parameters were recorded for 2016–17 and 2017–18. Most of the traits showed no significant difference between the years except a few (Table 1 ). We used pooled means of two years to assess the performance of crosses in each group grown under low N soil.
Differential response to limited N in terms of phenology, grain yield, and grain protein concentration
The average number of days to attain GS65 in both years ranged from 97 to 123 days after sowing (DAS) while the days to reach GS91 ranged from 23 to 48 days after attaining GS65 (Additional file 1 : Table S2). Among the crossing groups, average number of days to reach GS65 was lesser in P × Cim (105 days) and P × Baj (104 days) than in P × Wat (112 days) and P × Wya (115 days). Likewise, maximum post-anthesis duration was recorded in P × Cim and P × Baj groups which was 40 and 41 days respectively while it was less than 35 days for other two groups i.e., 34 and 31 days for P × Wat and P × Wya respectively.
The grain yield ( P < 0.05) varied between lines across 2 years but the average grain yield among the four groups was statistically similar (Additional file 1 : Table S4; Table 1 ). The TGW and GPC varied significantly ( P < 0.05) between 195 lines and crossing groups. Among different groups, the pooled values of grain yield and TGW were significantly higher in P × Cim but the GPC was ( P < 0.05) lower compared to other three groups (Table 1 ). In P × Wat and P × Wya, grain yield and TGW were lower but the GPC was higher ( P < 0.05) compared to the other groups. The Pearson’s correlation obtained for 195 lines showed that the grain yield was positively correlated (r = 0.45; P < 0.001) with TGW but was negatively correlated (r = − 0.24; P < 0.001) with GPC (Fig. 2 , Additional file 1 : Table S6). Further, the P × Wat group showed a significant negative correlation between GPC and grain yield (r = − 0.17; P < 0.05); though the other three groups also showed similar association but the interaction was non-significant (Table 3 ). The AGNpa exhibited a positive correlation with GPC in P × Cim (r = 0.50; P < 0.01) and P × Wat (r = 0.40; P < 0.001) as compared to AGNa (r = 0.36; P < 0.05 and r = 0.36; P < 0.001 respectively), however, these associations were not significant in P × Baj and P × Wya (Tables 2 and 3 ).
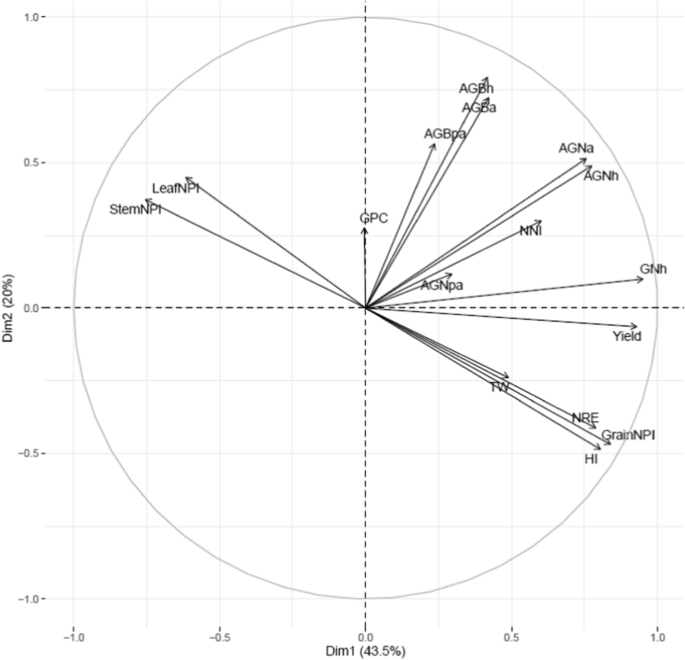
Principal component analysis of various traits using 195 RILs derived from NAM population grown in low N soil. Pooled data was used for PCA. Yield grain yield; NRE N remobilization efficiency; AGNa aboveground N uptake at anthesis; AGNpa aboveground N uptake at post-anthesis; AGNh aboveground N uptake at harvest; GNh grain N uptake at harvest; AGBa aboveground biomass at anthesis; AGBpa aboveground biomass at post-anthesis; AGBh aboveground biomass at harvest; NNI nitrogen nutrition index; HI harvest index; GPC grain protein concentration; TW test-weight; StemNPI stem N partitioning index; LeafNPI leaf N partitioning index; GrainNPI grain N partitioning index
Out of 195 lines, 94 produced higher grain yields than the overall mean value (3008 kg ha −1 ), out of which 47 lines belonged to P × Wat, 30 were from P × Cim, and 14 were from P × Baj group (Fig. 3 a–d). Among the 94 lines with higher than the average grain yield, 27 lines showed GPC higher than the overall mean GPC (14.4%). Out of these 27 lines with higher grain yield and GPC, 21 belonged to P × Wat group (P × Wat34-33, P × Wat34-44, P × Wat34-52, P × Wat34-70, P × Wat34-93, P × Wat223-86, P × Wat264-20, P × Wat264-80, P × Wat264-85, P × Wat273-10, P × Wat291-16, P × Wat291-62, P × Wat291-73, P × Wat292-5, P × Wat292-50, P × Wat292-560, P × Wat292-70, P × Wat420-39, P × Wat566-10, P × Wat566-28, and P × Wat685-2), three lines were from P × Cim (P × Cim47-328, P × Cim49-116, and P × Cim49-265), two lines were from P × Baj group (P × Baj-56 and P × Baj-62), and one line belonged to P × Wya group (P × Wya-48) (Fig. 3 a–d).
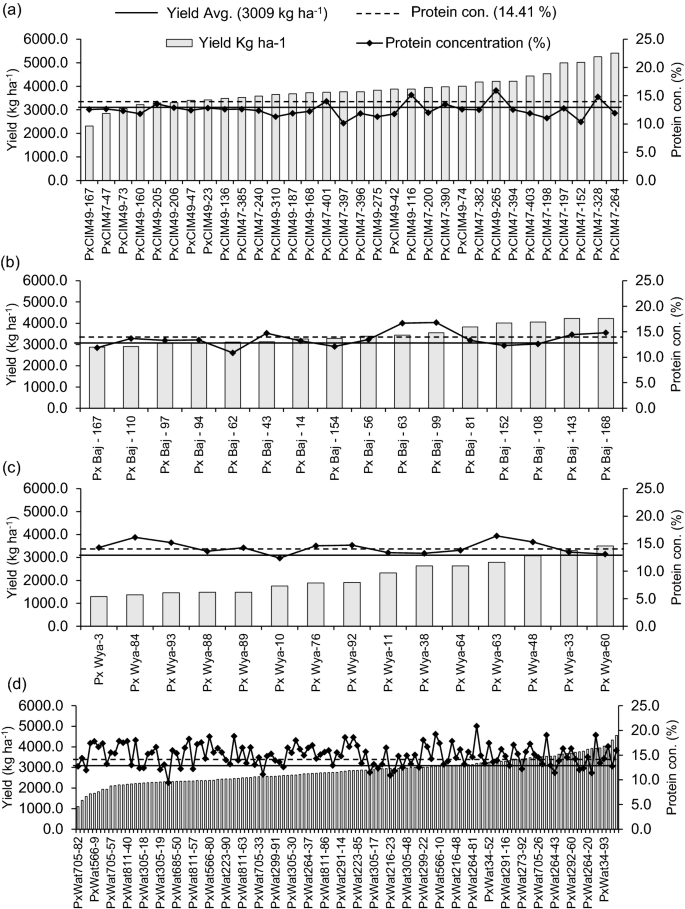
Variation in grain yield and grain protein concentration of wheat belonging to different groups of crosses grown in low N soil. a Paragon × CIMCOG (n = 32), b Paragon × Baj (n = 16), c Paragon × Wyalkatchem (n = 15), and d Paragon × Watkins (n = 132). Bar represents the pooled mean of grain yield for two seasons (2016–17 and 2017–18) and solid line shows the mean of protein concentration
Aboveground biomass accumulation during anthesis majorly contributes to grain yield
Wide variation ( P < 0.05) was observed among 195 lines for biomass accumulation at anthesis, post-anthesis, and at harvest between the two years (Additional file 1 : Table S4). The average of 2 years for aboveground biomass at anthesis (AGBa) was 7618 kg ha −1 while at post-anthesis (AGBpa) and harvest (AGBh), it was 3977 and 11,530 kg ha −1 respectively. The correlation matrix for 195 lines showed a positive association between grain yield and biomass accumulation at anthesis (r = 0.40; P < 0.001), post-anthesis (r = 0.27; P < 0.01), and at maturity (r = 0.42; P < 0.001) (Fig. 2 , Additional file 1 : Table S6).
Among the four groups, the maximum pooled biomass at anthesis, was recorded in P × Baj (7756 kg ha −1 ), while at post-anthesis, the highest biomass was accumulated in P × Cim (4096 kg ha −1 ). Out of the four groups, the lowest biomass was accumulated in P × Wya (6979 kg ha −1 ) at anthesis and in P × Baj (3793 kg ha −1 ) during post-anthesis (Fig. 4 ). In P × Cim, pre-anthesis biomass contributed about 70% of the total biomass accumulated at maturity while it was 65% in P × Wya. The groups P × Cim and P × Wat showed significant positive correlation of grain yield with AGBa (P × Cim r = 0.54; P < 0.01, P × Wat r = 0.51; P < 0.001) and with AGBh (P × Cim r = 0.53; P < 0.01, P × Wat r = 0.48; P < 0.001) (Tables 2 and 3 ). Furthermore, P × Cim (r = 0.57; P < 0.001) and P × Wat (r = 0.38; P < 0.001) also showed a significant positive correlation of AGBa with grain nitrogen uptake at harvest (GNh), but this association was non-significant in P × Baj and P × Wya. However, no significant relationship between AGBpa and grain yield was observed for any group except P × Wat (r = 0.25; P < 0.01) (Tables 2 and 3 ). Out of 195 lines, 54 accumulated aboveground biomass greater than the averages at both anthesis (7618 kg ha −1 ) and post-anthesis (3912 kg ha −1 ) stages. Among the 54 lines, nine belonged to P × Cim group, five lines were from P × Baj, 39 lines from P × Wat, and one line was from the P × Wya group (Additional file 1 : Table S2).
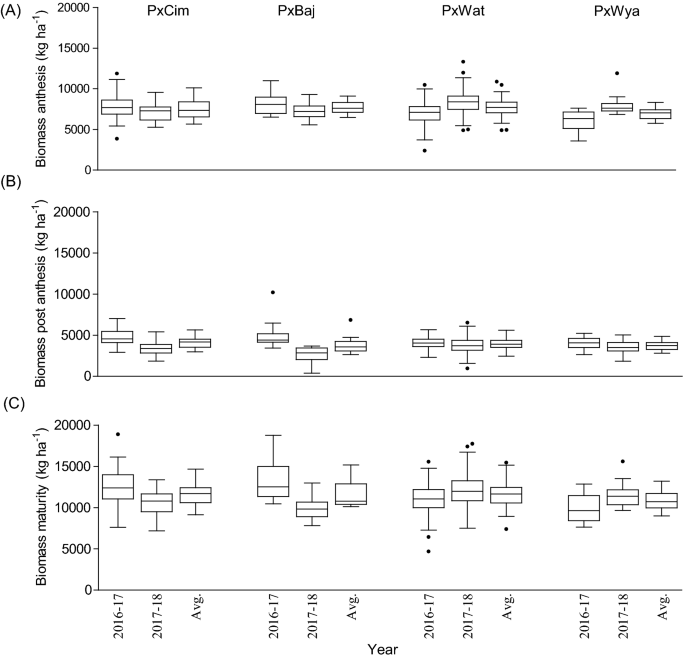
Box and whisker plot showing variation among different crosses, Paragon × CIMCOG (n = 32), Paragon × Baj (n = 16), Paragon × Watkins (n = 132), and Paragon × Wyalkatchem (n = 15), with respect to aboveground biomass accumulation at low N conditions evaluated in the field for two seasons. a biomass accumulated at anthesis, b biomass accumulated at post-anthesis, and c biomass accumulated at harvest. Black line (–) inside boxes indicates mean value and solid circles denote ‘outliers’ according to Tukey’s test
Total grain N uptake and yield are primarily dependent on aboveground N uptake at anthesis
Significant variation ( P < 0.01) was observed between lines for the aboveground N uptake at anthesis (AGNa), post-anthesis (AGNpa), and at harvest (AGNh) (Additional file 1 : Table S4). The AGNa contributed about 71% of total N mobilized towards grain while the contribution of post-anthesis N uptake was only 29%. Correlation analysis of datasets for 195 lines showed a positive relationship of grain yield with AGNa (r = 0.63; P < 0.001) and AGNpa (r = 0.23; P < 0.01). However, grain yield was negatively correlated with leaf N (r = − 0.18; P < 0.05) and stem N at harvest (r = − 0.15; P < 0.05) (Fig. 2 , Additional file 1 : Table S6).
Among the four groups, no significant difference was observed for N uptake (Table 1 ). Overall, AGNa was highest in P × Cim (115 kg ha −1 ) that contributed about 80% towards the total N accumulated in grains. The highest post-anthesis N uptake was recorded in P × Wat (24 kg ha −1 ), which contributed 31% towards the total N uptake in grains. The uptake of pre- and post-anthesis N was lowest in P × Wya as compared to other groups. Both pre- or post-anthesis N uptake showed positive corelations with grain yield (Fig. 5 ). The linear regression between AGNa and grain yield was significant in all groups (P × Cim R 2 = 0.45; P < 0.001, P × Wat R 2 = 0.32; P < 0.001, and P × Wya R 2 = 0.40; P < 0.05) except P × Baj. Similarly, the relationship between AGNpa and yield was significant in all groups (P × Cim R 2 = 0.14; P < 0.05, P × Wat R 2 = 0.16; P < 0.001, and P × Wya R 2 = 0.42; P < 0.01) except P × Baj (Fig. 6 ).
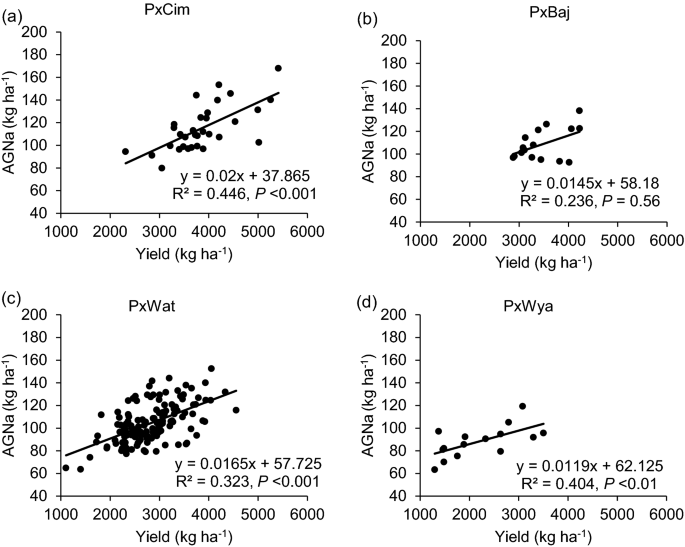
Linear regression between aboveground N uptake at anthesis (AGNa) and grain yield of groups of wheat belonging to different crosses grown in low N field. a Paragon × CIMCOG (n = 32), b Paragon × Baj (n = 16), c Paragon × Watkins (n = 132), and d Paragon × Wyalkatchem (n = 15). Values used are mean of 2 years, 2016–17 and 2017–18
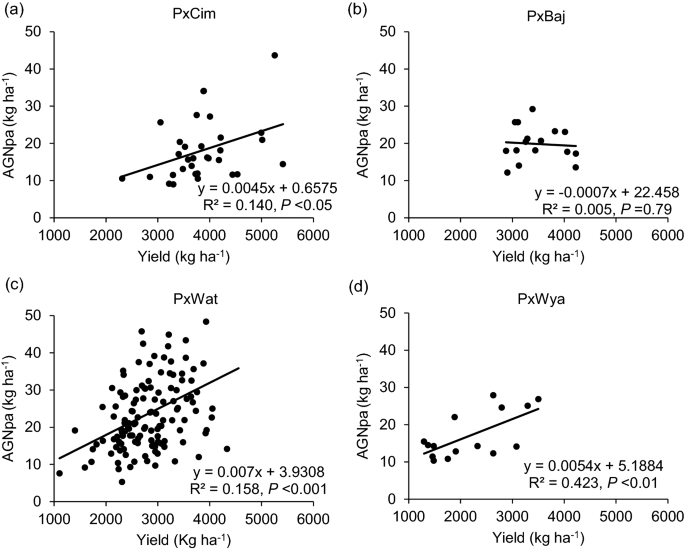
Linear regression between above ground N uptake at post-anthesis (AGNpa) and grain yield of groups of wheat belonging to different crosses grown in low N field. a Paragon × CIMCOG (n = 32), b Paragon × Baj (n = 16), c Paragon × Watkins (n = 132), and d Paragon × Wyalkatchem (n = 15) populations grown in low N field. Values used are mean of 2 years, 2016–17 and 2017–18
Relationship between N partitioning indices with grain yield and grain N content
We used NNI at anthesis as an indicator of N stress experienced by wheat plants grown in soil with limited N availability. This varied significantly ( P < 0.01) among 195 RILs (Additional file 1 : Table S4) but was not significant between the years or groups (Table 1 ). The pooled values of NNI over years for the whole population showed significant positive correlations with grain yield (r = 0.44; P < 0.001) and grain N partitioning index (grain NPI) (r = 0.30; P < 0.001) but negatively correlated with leaf N partitioning index (leaf NPI) (r = − 0.19; P < 0.01) and stem partitioning index (stem NPI) (r = − 0.29; P < 0.001) (Additional file 1 : Table S6). Furthermore, the low yielding lines retained more N in the leaf and stem at harvest which was demonstrated by strong negative correlations between grain yield, stem NPI (r = − 0.68; P < 0.001) and leaf NPI (r = − 0.61; P < 0.001 (Fig. 2 , Additional file 1 : Table S6).
NNI was also significantly correlated with grain yield in crossing groups such as P × Wat (r = 0.35; P < 0.001), P × Wya (r = 0.57; P < 0.05), while P × Cim (r = 0.31; P = 0.35) showed a positive but non-significant correlation (Tables 2 and 3 ). However, the linear regression of NNI with N-partitioning index between leaf, stem and grain did not show any significant relationship except in the group P × Wya for stem NPI (R 2 = 0.52; P < 0.05) (Fig. 7 ). Interestingly, all groups except P × Baj showed significant positive correlations of grain-NPI with grain yield while stem and leaf-NPI were negatively correlated with grain yield and grain N content (Tables 2 and 3 ).
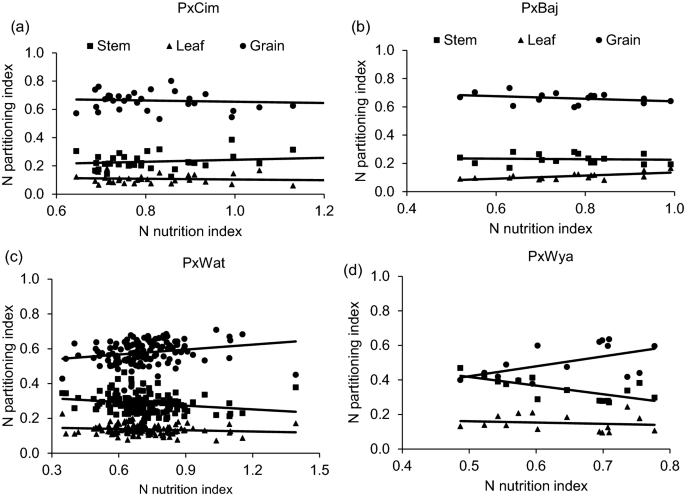
Linear regression of N nutrition index (NNI) at anthesis versus N partitioning index at harvest of different groups in wheat grown in low N field. a Paragon × CIMCOG (n = 32), leaf y = − 0.0258x + 0.1292, R 2 = 0.0119; stem y = 0.0698x + 0.173, R 2 = 0.0297; grain y = − 0.044x + 0.698, R 2 = 0.0089, b Paragon × Baj (n = 16), leaf y = 0.109x + 0.0264, R 2 = 0.400; stem y = − 0.0184x + 0.244, R 2 = 0.005; grain y = -0.0907x + 0.729, R 2 = 0.104, c Paragon × Watkins (n = 132), leaf y = -0.025x + 0.153, R 2 = 0.0186; stem y = -0.0705x + 0.337, R 2 = 0.0412; grain y = 0.095x + 0.510, R 2 = 0.0496, and d Paragon × Wyalkatchem (n = 15), leaf y = − 0.0734x + 0.197, R 2 = 0.0198; stem y = − 0.4994x + 0.667, R 2 = 0.517; grain y = 0.272x + 0.1354, R 2 = 0.290. Values used are mean of 2 years, 2016–17 and 2017–18
Association of nitrogen remobilization efficiency with biomass accumulation, grain protein concentration, and yield attributes
The N-remobilization efficiency (NRE) varied significantly ( P < 0.01) among 195 RILs in both years with pooled mean of 50% (Additional file 1 : Table S4). NRE showed significant ( P < 0.001) positive correlations with AGNa, AGNh, GNh, grain yield, and TGW. However, NRE was highly negatively correlated ( P < 0.001) with stem-NPI, leaf-NPI and GPC but exhibited a positive correlation with grain-NPI and harvest index (Fig. 2 ; Additional file 1 : Table S6).
NRE varied significantly ( P < 0.05) among the groups and between the years (Table 1 ). The mean NRE was significantly higher in P × Cim and P × Baj and lowest in P × Wya. The linear regression showed a significant positive relationship between NRE and grain yield in all groups, P × Cim (R 2 = 0.35; P < 0.001), P × Wat (R 2 = 0.30; P < 0.001), and P × Wya (R 2 = 0.87; P < 0.001) except P × Baj (R 2 = 0.05; P = 0.39) (Fig. 8 ). The Pearson’s correlation coefficient of NRE with other traits also showed a positive correlation with AGNa in P × Wat (r = 0.33; P < 0.001) and P × Wya (r = 0.64; P < 0.01) while it was non-significant in other two groups (Tables 2 and 3 ). However, no significant association was found between NRE and AGNpa in different groups except in P × Wat (r = -0.19; P < 0.05). Further, there was a positive correlation between NRE and grain-NPI in P × Cim (r = 0.94; P < 0.001), P × Wat (r = 0.22; P < 0.01), and P × Wya (r = 0.97; P < 0.001). In all groups, NRE was negatively correlated ( P < 0.001) with leaf and stem-NPI.
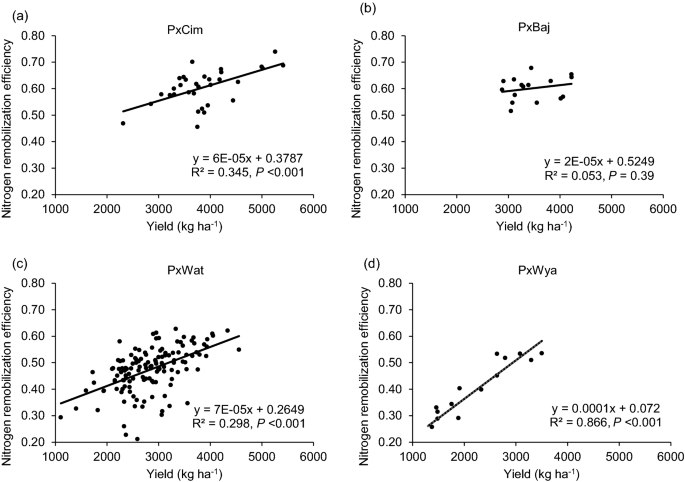
Linear regression between N remobilization efficiency at harvest and grain yields of groups of wheat belonging to different crosses grown in low N field. a Paragon × CIMCOG (n = 32), b Paragon × Baj (n = 16), c Paragon × Watkins (n = 132), and d Paragon × Wyalkatchem (n = 15). Values used are mean of 2 years, 2016–17 and 2017–18
Pre- and post-anthesis N uptake contributes to grain protein deviation
The GPD varied significantly ( P < 0.01) among 195 RILs in both years ranging between − 2.47 to 3.14 in 2016–17 and − 2.48 to 2.92 in 2017–18 (Additional file 1 : Table S4). Out of 195 RILs, 20 lines showed reverse trend while all others showed similar positive GPD in both seasons. Lines exhibiting positive GPD accumulated more protein in grains than lines with negative GPD (Additional file 1 : Fig. S2). Pearson’s correlation coefficient showed a significant positive correlation of GPD with AGNa (r = 0.36; P < 0.001) and AGNpa (r = 0.58; P < 0.001) amongst 195 RILs (Additional file 1 : Table S6). Moreover, GPD was significantly correlated with AGNa in all four groups (Tables 2 and 3 ). Interestingly, significant positive correlations between GPD and post-anthesis N uptake were also observed in P × Cim (r = 0.50; P < 0.001) and P × Wat (r = 0.48; P < 0.001).
Identification of low N stress tolerant wheat lines based on NRE, grain yield and protein concentration
The 195 lines were grouped by Ward’s clustering method using pooled mean of yield and NRE to identify lines that performed good under low N condition. Four distinct clusters were identified, namely, efficient (average grain yield 4161 kg ha −1 and NRE 0.62), moderately efficient (average grain yield 3357 kg ha −1 and NRE 0.56), moderately inefficient (average grain yield 2675 kg ha −1 and NRE 0.49), and inefficient (average grain yield 2219 kg ha −1 and NRE 0.37) (Fig. 9 a). Of the 195 lines, 31 were included in the efficient cluster, 59 in the moderately efficient, 58 in moderately inefficient, and 47 were classified as inefficient. Furthermore, within the efficient cluster, 16 lines were from P × Cim, 10 lines were from P × Wat, and 5 lines were from P × Baj while in the inefficient cluster, 38 lines were from P × Wat and 9 lines belonged to P × Wya. The lines present in the efficient cluster exhibited significantly higher biomass accumulation, N uptake, yield traits and NRE than the inefficient cluster (Additional file 1 : Table S5). Most of the lines in the efficient cluster possessed shorter pre-anthesis duration and longer post-anthesis duration as compared to inefficient or moderately inefficient lines (Additional file 1 : Table S4). Further, in the efficient lines, AGNa contributed more than 75% towards total N accumulated in the grains while in the inefficient lines, it was ~ 63%; the remaining part of N in grains was remobilized from AGNpa.
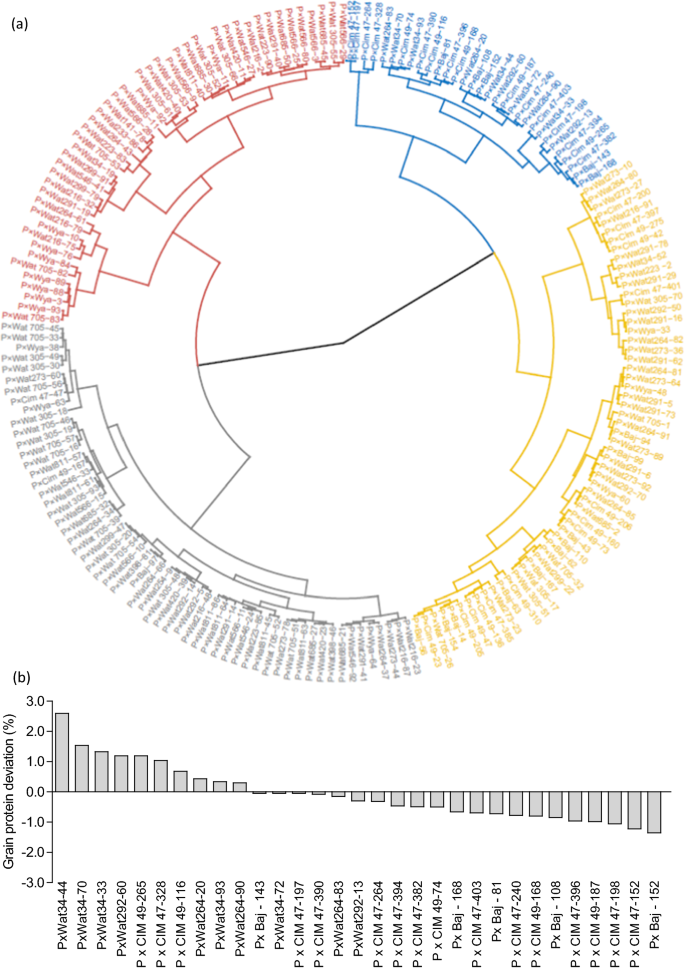
a Circular dendrogram depicting clustering of 195 wheat RILs derived from NAM population into four distinct clusters based on two years pooled mean of grain yield and nitrogen remobilization efficiency (NRE). Cluster 1 (blue color) represents 31 efficient lines, cluster 2 (yellow color) represents 59 moderately efficient lines, cluster 3 (black color) represents 58 moderately inefficient lines, and cluster 4 (red color) represents 47 inefficient lines. b The lines of efficient cluster (31 numbers) were ranked according to grain protein deviation (GPD) determined from the regression of pooled mean of grain yield on pooled mean of grain protein concentration (GPC)
To identify the lines combining high grain yield, GPC, and NRE, we ranked the 31 lines of efficient cluster for GPD. Ten of these lines exhibited positive GPD in both the years (Fig. 9 b): three from the P × Cim group (P × Cim47-328, P × Cim49-116, and P × Cim49-265) and seven from the P × Wat group (P × Wat34-33, P × Wat34-44, P × Wat34-70, P × Wat34-93, P × Wat264-20, P × Wat264-90, P × Wat292-60).
Biomass accumulation is the ultimate outcome of environmental CO 2 -fixation into organic matter through photosynthesis whereas grain yield is the fraction of the accumulated biomass which is translocated from vegetative tissues to the grain during post-anthesis growth stages (Paul et al. 2016 ). It is well established that N deficiency decreases the photosynthetic rate resulting in reduced biomass accumulation which ultimately affects grain yield. However, N-efficient genotypes can produce better yields under N-limiting condition. In the present study, we did not find any significant correlation between grain yield and pre- or post-anthesis durations. However, results indicated that the N efficient lines took lesser number of days to reach anthesis stage (GS65) as compared to the inefficient lines indicating that the efficient lines received sufficient time for grain development before the sudden rise in temperature from mid-April (Additional file 1 : Fig. S1). Reports have confirmed that post-anthesis, if the plants are exposed to heat stress even if it is at 10 days after anthesis, there will be more negative impact on yield components, indicating that well-fertilized wheat may be more sensitive to heat stress (Elia et al. 2018 ; Dubey et al. 2020 ). Studies shown that the post-anthesis heat stress can cause considerable grain abortion which dramatically reduces grain filling rate and in turn, reduces grain weight (Mondal et al. 2015 ; Slafer et al. 2015 ). These reports are in agreement with our results as N-inefficient lines showed lower TGW than the N-efficient lines (Additional file 1 : Table S5). This implies that in the near future, when the terminal heat stress events become more frequent, the impact of such N-efficient lines would be more useful for designing future wheat varieties.
Our results also revealed a positive correlation between grain yield and biomass accumulation at anthesis (AGBa) in the P × Cim and P × Wat groups (Tables 2 and 3 ). These results are in agreement with earlier studies which indicated that higher grain yield is a consequence of higher biomass accumulation at anthesis rather than post-anthesis stage (Shearman et al. 2005 ; Malik et al. 2016 ). It was shown that more that 75% of total biomass accumulated during the pre-anthesis stage in wheat was remobilized to the grains under drought stress (Yang et al. 2001 ). This is because improving the harvest index has been the most widely used strategy to increase grain yield of modern wheat genotypes (Acreche et al. 2008 ; Meng et al. 2013 ; Mahjourimajd et al. 2016 ). However, this strategy needs to maintain optimum leaf area for maximum interception of sun-light, storage of photo-assimilates, and N for their re-translocation during grain filling (Donmez et al. 2001 ). By contrast, grain yield in P × Baj and P × Wat groups showed positive correlations with both AGBa and AGBpa (Tables 2 and 3 ), which is in agreement with earlier reports on wheat grown in China (Pan et al. 1999 ). It has been argued that increasing dry matter at critical growth stages is an indispensable requirement for enhancing grain yield (Ye et al. 2011 ). After anthesis, the shoot, particularly stems (culm), that acted as a ‘sink’ before anthesis, becomes a ‘source’, because after anthesis the developing grains becomes the major sink and the photosynthates are mobilized directly to the developing grains from the stem (source). However, our results indicated that the relationships between grain yield with pre- or post-anthesis biomass accumulation are also affected by genetic factors, and that the increases in grain yield due to improvement of pre-anthesis biomass may not occur in all genotypes.
The developing cereal grain is the most active sink for N assimilates and the uptake of N during vegetative growth is therefore essential for determining grain yield and quality. In the present study, AGNa and AGNpa were found to be significantly positively correlated with grain yield and GPD (Tables 2 and 3 ). Earlier studies have shown that the AGNa not only contributed the greatest part of total N uptake at maturity but also exhibited a significant positive correlation with grain yield and grain N (Cox et al. 1986 ; Papakosta and Garianas 1991 ; Hocking and Staper 2001 ; Malik et al. 2016 ). Furthermore, a similar association between AGNpa and grain yield under low N conditions was reported in winter wheat but this was non-significant under high N condition (Barraclough et al. 2014 ). AGNa was reported to be strongly correlated with flag-leaf senescence and can therefore be considered as an important trait for improving grain yield under low N supply (Nehe et al. 2018 ). Consequently, traits favouring AGNa could be a possible target to breed for improved grain yield under low N conditions. We observed a wide variation in N partitioning at harvest among the groups with a negative relationship between grain yield with leaf- and stem-NPI (Tables 2 and 3 ; Additional file 1 : Table S6). Gregory et al. ( 1979 ) reported that wheat stem (with sheath) contained more N at maturity followed by the leaf. However, a detailed study of N partitioning in leaf, stem, and sheath showed that most of the N was retained in the leaf blades followed by the stem and leaf sheath (Barraclough et al. 2014 ). We found a significant positive association of GPD with pre- and post-anthesis N uptake, whereas earlier studies related GPD to post-anthesis N uptake only (Monaghan et al. 2001 ; Bogard et al. 2010 ). Post-anthesis N uptake does not induce any plastic growth responses in plants and may affect grain yield and GPC (Bogard et al. 2010 ). Studies have also shown that wheat cultivars developed in the UK possessed higher GPD and stability than those bred in other European countries and grown under UK conditions, with strong genetic variation and significant effects of N level (Mosleth et al. 2020 ).
We found a strong positive association between AGNa and NRE but a negative correlation between AGNpa and NRE (Additional file 1 : Table S6). Usually under optimum N, plants remobilize pre-anthesis accumulated N towards grain development but under low N, this relationship does not hold true. This result is in agreement with Nehe et al. ( 2020 ) who found a similar relationship in 28 Indian wheat cultivars grown under low N condition. This suggests that higher N uptake at anthesis favours higher post-anthesis N remobilization indicating that in this study, NRE was probably a source-driven trait. However, it may be possible that higher N uptake at anthesis is associated with higher grains m −2 and NRE; and the association between NRE and yield is an indirect effect of an association with anthesis N uptake which needs further study. We found a positive association between NRE and grain yield in all four crossing groups (Tables 2 and 3 ). This is in agreement with Nehe et al. ( 2018 ) who also reported a strong positive association between grain yield and NRE under both low and high N conditions in Indian wheat cultivars. They observed that the higher flag-leaf NRE was associated with higher N uptake at anthesis and slower flag leaf senescence, thereby delaying senescence and improving grain yield. On the contrary, Gaju et al. ( 2016 ) reported that higher NRE was related to faster flag leaf senescence. However, the slower senescence or stay-green phenotype has been linked to increased grain yield in different cereals under terminal abiotic (heat and drought) stresses (reviewed by Gregersen et al. 2013 ; Christopher et al. 2016 ).
Improved yield and grain quality are the two important goals in wheat production, the former being a major determinant of crop productivity while the latter determines end-use value and processing quality. We found a strong negative correlation between grain yield and GPC for all 195 lines (Additional file 1 : Table S6) but this association was weaker among the four groups, and not significant in the P × Cim group. A similar strong negative correlation between grain protein concentration and grain yield was reported in wheat (Simmonds 1995 ; Bogard et al. 2010 ; Nehe et al. 2020) which makes it difficult to improve both traits simultaneously (Slafer and Andrade 1993 ). Contrary to this, other studies suggested that the GPC can be increased without compromising grain yield either by an accelerated senescence rate to increase the N remobilization (Uauy et al. 2006a , 2006b ) or by maintaining a higher NRE by increasing N uptake during anthesis (Slafer and Andrade 1993 ). In our study, we identified ten superior lines combining higher grain yield with positive GPD in the P × Cim and P × Wat groups (Fig. 9 b). These N efficient lines belonging to NAM population derived from crosses of diverse wheat landraces/cultivars performed well under north Indian condition with limited N availability and thus, could be introduced as varieties or provide valuable genetic resource for improving grain yield as well as protein concentration for sustainable wheat production.
From our study, we concluded that the genetic variation in accumulation of biomass at both pre- and post-anthesis stages was correlated with grain-yield. The N-remobilization efficiency (NRE) was positively correlated with above-ground N uptake at anthesis (AGNa) and grain yield but negatively associated with AGN at post-anthesis (AGNpa) suggesting that higher N uptake till anthesis would favour higher N remobilization during grain filling. The AGNa and AGNpa also showed a positive association with grain protein deviation (GPD). We propose that traits favouring pre- or post-anthesis biomass accumulation and pre-anthesis N uptake may be targeted for breeding to improve grain-yield under limited N. Among the groups, AGNa, AGN at harvest (AGNa), NRE, grain yield, and 1000 grain weight were highest in the P × Cim and lowest in P × Wya group with limited N input in northern India. From the cluster analysis, we found 16 lines of P × Cim, 10 from P × Wat, and 5 from P × Baj to be N-efficient. These efficient lines were also ranked based on GPD and found several lines of P × Cim and P × Wat groups exhibiting positive GPD combined with higher grain yield and GPC. The GPC is a major determinant of wheat grain quality, but due to a strong negative correlation between GPC and grain yield, it is difficult to improve both traits simultaneously. Therefore, the identified RILs could be introduced as varieties or as genetic resource for improving grain yield with high GPC under limited N condition.
Availability of data and materials
The study has data published as Additional file 1
Abbreviations
Aboveground biomass at anthesis
Aboveground biomass at harvest
Aboveground biomass post anthesis
Above ground N uptake at anthesis
Above ground N uptake at harvest
Above ground N uptake at post-anthesis
Grain N uptake at harvest
- Grain protein concentration
- Grain protein deviation
Nested association mapping
- Nitrogen remobilization efficiency
Recombinant inbred lines
Wyalkatchem
Abrol YP, Pandey R, Raghuram N, Ahmad A. Nitrogen cycle sustainability and sustainable technologies for nitrogen fertilizer and energy management. J Indian Inst Sci. 2012;92:17–36.
CAS Google Scholar
Acreche MM, Briceño-Félix G, Sánchez JAM, Slafer GA. Physiological bases of genetic gains in Mediterranean bread wheat yield in Spain. Eur J Agron. 2008;28:162–70. https://doi.org/10.1016/j.eja.2007.07.001 .
Article Google Scholar
Agres. Statistical software version 3.01. California: Pascal International Software Solutions; 1994.
Google Scholar
Amalova AY, Yermekbayev KA, Griffiths S, Abugalieva SI, Turuspekov YK. Phenotypic variation of common wheat mapping population Pamyati Azieva x Paragon in south-east of Kazakhstan. Int J Bio Chem. 2019;12:11–7. https://doi.org/10.26577/ijbch-2019-i1-3 .
Article CAS Google Scholar
Andersson A, Johansson E, Oscarson P. Nitrogen redistribution from the roots in post-anthesis plants of spring wheat. Plant Soil. 2005;269:321–32. https://doi.org/10.1007/s11104-004-0693-6 .
Barraclough PB, Lopez-Bellido R, Hawkesford MJ. Genotypic variation in the uptake, partitioning and remobilisation of nitrogen during grain-filling in wheat. Field Crops Res. 2014;156:242–8. https://doi.org/10.1016/j.fcr.2013.10.004 .
Article PubMed PubMed Central Google Scholar
Bentley AR, Turner AS, Gosman N, Leigh FJ, Maccaferri M, Dreisigacker S. Frequency of photoperiod-insensitive Ppd-A1a alleles in tetraploid, hexaploid and synthetic hexaploid wheat germplasm. Plant Breed. 2011;130:10–5. https://doi.org/10.1111/j.1439-0523.2010.01802.x .
Bertholdsson NO, Stoy V. Yields of dry matter and nitrogen in highly diverging genotypes of winter wheat in relation to N-uptake and N-utilization. J Agron Crop Sci. 1995;175:285–95. https://doi.org/10.1111/j.1439-037X.1995.tb00223.x .
Bogard M, Allard V, Brancourt-Hulmel M, Heumez E, Machet JM, Jeuffroy MH, Le Gouis J. Deviation from the grain protein concentration–grain yield negative relationship is highly correlated to post-anthesis N uptake in winter wheat. J Exp Bot. 2010;61:4303–12. https://doi.org/10.1093/jxb/erq238 .
Article CAS PubMed Google Scholar
Borrell AK, Incoll LD, Simpson RJ, Dalling MJ. Partitioning of dry matter and the deposition and use of stem reserves in a semi-dwarf wheat crop. Ann Bot. 1989;63:527–39. https://doi.org/10.1093/oxfordjournals.aob.a087778 .
Christopher JT, Christopher MJ, Borrell AK, Fletcher S, Chenu K. Stay-green traits to improve wheat adaptation in well-watered and water-limited environments. J Exp Bot. 2016;67:5159–72. https://doi.org/10.1093/jxb/erw276 .
Article CAS PubMed PubMed Central Google Scholar
Cox MC, Qualset CO, Rains DW. Genetic variation for nitrogen assimilation and translocation in wheat. II. Nitrogen assimilation in relation to grain yield and protein 1. Crop Sci. 1985;25:435–40.
Cox MC, Qualset CO, Rains DW. Genetic variation for nitrogen assimilation and translocation in wheat. III. Nitrogen translocation in relation to grain yield and protein. Crop Sci. 1986;26:737–40.
Dalling MJ. The physiological basis of nitrogen redistribution during grain filling in cereals. In: Harper JE, Schrader LE, Howell RW, editors. Exploration of physiological and genetic variability to enhance crop productivity. Rockville: American society of plant physiologists; 1985. p. 55–71.
De Santis MA, Giuliani MM, Flagella Z, Reyneri A, Blandino M. Impact of nitrogen fertilisation strategies on the protein content, gluten composition and rheological properties of wheat for biscuit production. Field Crops Res. 2020;254:107829. https://doi.org/10.1016/j.fcr.2020.107829 .
Donmez E, Sears RG, Shroyer JP, Paulsen GM. Genetic gain in yield attributes of winter wheat in the great plains. Crop Sci. 2001;41:1412–9. https://doi.org/10.2135/cropsci2001.4151412x .
Dubey R, Pathak H, Chakrabarti B, Singh S, Gupta DK, Harit RC. Impact of terminal heat stress on wheat yield in India and options for adaptation. Agric Syst. 2020;181:102826. https://doi.org/10.1016/j.agsy.2020.102826 .
Elia M, Slafer GA, Savin R. Yield and grain weight responses to post-anthesis increases in maximum temperature under field grown wheat as modified by nitrogen supply. Field Crops Res. 2018;221:228–37. https://doi.org/10.1016/j.fcr.2018.02.030 .
FAO (2021). World Food and Agriculture - Statistical Yearbook 2021. Rome. pp. 157. https://doi.org/10.4060/cb4477en .
Fuertes-Mendizábal T, González-Murua C, González-Moro MB, Estavillo JM. Late nitrogen fertilization affects nitrogen remobilization in wheat. J Plant Nutr Soil Sci. 2012;175:115–24. https://doi.org/10.1002/jpln.201000299 .
Gaju O, Allard V, Martre P, Le Gouis J, Moreau D, Bogard M, Foulkes MJ. Nitrogen partitioning and remobilization in relation to leaf senescence, grain yield and grain nitrogen concentration in wheat cultivars. Field Crops Res. 2014;155:213–23. https://doi.org/10.1016/j.fcr.2013.09.003 .
Gaju O, DeSilva J, Carvalho P, Hawkesford MJ, Griffiths S, Greenland A, Foulkes MJ. Leaf photosynthesis and associations with grain yield, biomass and nitrogen-use efficiency in landraces, synthetic-derived lines and cultivars in wheat. Field Crops Res. 2016;193:1–15. https://doi.org/10.1016/j.fcr.2016.04.018 .
Gonzalez-Navarro OE, Griffiths S, Molero G, Reynolds MP, Slafer GA. Variation in developmental patterns among elite wheat lines and relationships with yield, yield components and spike fertility. Field Crops Res. 2016;196:294–304. https://doi.org/10.1016/j.fcr.2016.07.019 .
Gregersen PL, Culetic A, Boschian L, Krupinska K. Plant senescence and crop productivity. Plant Mol Biol. 2013;82:603–22. https://doi.org/10.1007/s11103-013-0013-8 .
Gregory PJ, Crawford DV, McGowan M. Nutrient relations of winter wheat. Accumulation and distribution of Na, K, Ca, Mg, P, S and N. J Agric Sci. 1979;93:485–94. https://doi.org/10.1017/S0021859600038181 .
Halloran GM. Cultivar differences in nitrogen translocation in wheat. Aust J Agri Res. 1981;32:535–44.
Hocking PJ, Staper M. Effects of sowing time and nitrogen fertiliser on canola and wheat, and nitrogen fertiliser on Indian mustard. I. Dry matter production, grain yield, and yield components. Aust J Agri Res. 2001;52:623–34. https://doi.org/10.1071/AR00113 .
Jeuffroy MH, Bouchard C. Intensity and duration of nitrogen deficiency on wheat grain number. Crop Sci. 1999;39:1385–93. https://doi.org/10.2135/cropsci1999.3951385x .
Jiang WZ. Comparison of responses to Mn deficiency between the UK wheat genotypes Maris Butler, Paragon and the Australian wheat genotype C8MM. J Integr Plant Biol. 2008;50:457–65. https://doi.org/10.1111/j.1744-7909.2007.00631.x .
Jiang Z, McDonald BC, Worden H, Worden JR, Miyazaki K, Qu Z, Boersma KF. Unexpected slowdown of US pollutant emission reduction in the past decade. PNAS. 2018;115:5099–104. https://doi.org/10.1073/pnas.1801191115 .
Justes E, Mary B, Meynard JM, Machet JM, Thelier-Huché L. Determination of a critical nitrogen dilution curve for winter wheat crops. Ann Bot. 1994;74:397–407. https://doi.org/10.1006/anbo.1994.1133 .
Maeoka RE, Sadras VO, Ciampitti IA, Diaz DR, Fritz AK, Lollato RP. Changes in the phenotype of winter wheat varieties released between 1920 and 2016 in response to in-furrow fertilizer: Biomass allocation, yield, and grain protein concentration. Front Plant Sci. 2020;10:1786. https://doi.org/10.3389/fpls.2019.01786 .
Mahjourimajd S, Kuchel H, Langridge P. Evaluation of Australian wheat genotypes for response to variable nitrogen application. Plant Soil. 2016;399:247–55. https://doi.org/10.1007/s11104-015-2694-z .
Malik AI, Veres S, Rengel Z. Differential nitrogen-use efficiency in wheat parents of doubled-haploid mapping populations. Plant Soil. 2016;408:311–25. https://doi.org/10.1007/s11104-016-2943-9 .
Martre P, Porter JR, Jamieson PD, Triboï E. Modeling grain nitrogen accumulation and protein composition to understand the sink/source regulations of nitrogen remobilization for wheat. Plant Physiol. 2003;133:1959–67. https://doi.org/10.1104/pp.103.030585 .
Meng F, Liu H, Wang K, Liu L, Wang S, Zhao Y, Li Y. Development-associated microRNAs in grains of wheat ( Triticum aestivum L.). BMC Plant Biol. 2013;13:140. https://doi.org/10.1186/1471-2229-13-140 .
Mi G, Tang L, Zhang F, Zhang J. Is nitrogen uptake after anthesis in wheat regulated by sink size? Field Crops Res. 2000;68:183–90. https://doi.org/10.1016/S0378-4290(00)00119-2 .
Monaghan JM, Snape JW, Chojecki AJS, Kettlewell PS. The use of grain protein deviation for identifying wheat cultivars with high grain protein concentration and yield. Euphytica. 2001;122:309–17. https://doi.org/10.1023/A:1012961703208 .
Mondal S, Joshi AK, Huerta-Espino J, Singh RP. Early maturity in wheat for adaptation to high temperature stress. In: Ogihara Y, Takumi S, Handa H, editors. Advances in wheat genetics: from genome to field. Tokyo: Springer; 2015. p. 239–45.
Chapter Google Scholar
Mosleth EF, Lillehammer M, Pellny TK, Wood AJ, Riche AB, Hussain A, Griffiths S, Hawkesford MJ, Shewry PR. Genetic variation and heritability of grain protein deviation in European wheat genotypes. Field Crops Res. 2020;255:107896. https://doi.org/10.1016/j.fcr.2020.107896 .
Nehe AS, Misra S, Murchie EH, Chinnathambi K, Foulkes MJ. Genetic variation in N-use efficiency and associated traits in Indian wheat cultivars. Field Crops Res. 2018;225:152–62. https://doi.org/10.1016/j.fcr.2018.06.002 .
Nehe AS, Misra S, Murchie EH, Chinnathambi K, Tyagi BS, Foulkes MJ. Nitrogen partitioning and remobilization in relation to leaf senescence, grain yield and protein concentration in Indian wheat cultivars. Field Crops Res. 2020;251:107778. https://doi.org/10.1016/j.fcr.2020.107778 .
Palta JA, Kobata T, Turner NC, Fillery IR. Remobilization of carbon and nitrogen in wheat as influenced by post-anthesis water deficits. Crop Sci. 1994;34:118–24. https://doi.org/10.2135/cropsci1994.0011183X003400010021x .
Pan QM, Yu ZW, Wang YF, Tian QZ. Studies on uptake and distribution of nitrogen in wheat at the level of 9000 kg per hectare. (In Chinese). Acta Agron Sin. 1999;25:541–7.
Papakosta DK, Garianas AA. Nitrogen and dry matter accumulation, remobilization, and losses for Mediterranean wheat during grain filling. Agron J. 1991;83:864–70. https://doi.org/10.2134/agronj1991.00021962008300050018x .
Pask AJD, Sylvester-Bradley R, Jameison PD, Foulkes MJ. Quantifying how winter wheat crops accumulate and use nitrogen reserves during growth. Field Crop Res. 2012;126:104–18. https://doi.org/10.1016/j.fcr.2011.09.021 .
Paul K, Pauk J, Deák Z, Sass L, Vass I. Contrasting response of biomass and grain yield to severe drought in Cappelle Desprez and Plainsman V wheat cultivars. Peer J. 2016;4:e1708. https://doi.org/10.7717/peerj.1708 .
Shearman VJ, Sylvester-Bradley R, Scott RK, Foulkes MJ. Physiological processes associated with wheat yield progress in the UK. Crop Sci. 2005;45:175–85. https://doi.org/10.2135/cropsci2005.0175a .
Shiferaw W, Abinasa M, Tadesse W. Evaluation of bread wheat ( Triticum aestivum L.) genotypes for stem and yellow rust resistance in Ethiopia. Comput Biol Bioinform. 2020;8:43. https://doi.org/10.11648/j.cbb.20200802.13 .
Simmonds NW. The relation between yield and protein in cereal grain. J Sci Food Agric. 1995;67:309–15. https://doi.org/10.1002/jsfa.2740670306 .
Slafer GA, Andrade FH. Physiological attributes related to the generation of grain yield in bread wheat cultivars released at different eras. Field Crops Res. 1993;31:351–67. https://doi.org/10.1016/0378-4290(93)90073-V .
Slafer GA, Elía M, Savin R, García GA, Terrile II, Ferrante A, Miralles DJ, González FG. Fruiting efficiency: an alternative trait to further rise wheat yield. Food Energy Secur. 2015;4:92–109. https://doi.org/10.1002/fes3.59 .
Sosulski FW, Imafidon GI. Amino acid composition and nitrogen-to-protein conversion factors for animal and plant foods. J Agric Food Chem. 1990;8:1351–6. https://doi.org/10.1021/jf00096a011 .
Sun C, Chen L, Zhai L, Liu H, Wang K, Jiao C, Shen Z. National assessment of nitrogen fertilizers fate and related environmental impacts of multiple pathways in China. J Clean Prod. 2020;277:123519. https://doi.org/10.1016/j.jclepro.2020.123519 .
Tyagi BS, Foulkes J, Singh G, Sareen S, Kumar P, Broadley MR, Singh GP. Identification of wheat cultivars for low nitrogen tolerance using multivariable screening approaches. Agronomy. 2020;10:417. https://doi.org/10.3390/agronomy10030417 .
Uauy C, Distelfeld A, Fahima T, Blechl A, Dubcovsky J. A NAC gene regulating senescence improves grain protein, zinc, and iron content in wheat. Science. 2006a;314:1298–301. https://doi.org/10.1126/science.1133649 .
Uauy C, Brevis JC, Dubcovsky J. The high grain protein content gene Gpc-B1 accelerates senescence and has pleiotropic effects on protein content in wheat. J Exp Bot. 2006b;57:2785–94. https://doi.org/10.1093/jxb/erl047 .
Voss-Fels KP, Stahl A, Wittkop B, Lichthardt C, Nagler S, Rose T, Snowdon RJ. Breeding improves wheat productivity under contrasting agrochemical input levels. Nat Plants. 2019;5:706–14. https://doi.org/10.1038/s41477-019-0445-5 .
Article PubMed Google Scholar
Waters BM, UauyC, Dubcovsky J, Grusak MA (2009) Wheat ( Triticum aestivum ) NAM proteins regulate the translocation of iron, zinc, and nitrogen compounds from vegetative tissues to grain. J Exp Bot. 2009;60:4263–4274. https://doi.org/10.1093/jxb/erp257 .
Wingen LU, Orford S, Goram R, Leverington-Waite M, Bilham L, Patsiou TS, Griffiths S. Establishing the AE Watkins landrace cultivar collection as a resource for systematic gene discovery in bread wheat. Theor Appl Genet. 2014;127:1831–42. https://doi.org/10.1007/s00122-014-2344-5 .
Wingen LU, West C, Leverington-Waite M, Collier S, Orford S, Goram R, Griffiths S. Wheat landrace genome diversity. Genetics. 2017;205:1657–76. https://doi.org/10.1534/genetics.116.194688 .
Yang J, Zhang J, Wang Z, Zhu Q, Wang W. Remobilization of carbon reserves in response to water deficit during grain filling of rice. Field Crops Res. 2001;71:47–55. https://doi.org/10.1016/S0378-4290(01)00147-2 .
Ye Y, Wang G, Huang Y, Zhu Y, Meng Q. Understanding physiological processes associated with yield–trait relationships in modern wheat varieties. Field Crops Res. 2011;124:316–22. https://doi.org/10.1016/j.fcr.2011.06.023 .
Zadoks JC, Chang TT, Konzak CF. A decimal code for the growth stages of cereals. Weed Res. 1974;14:415–21. https://doi.org/10.1111/j.1365-3180.1974.tb01084.x .
Zaicou-Kunesch C, Trainor G, Shackley B, Curry J, Nicol D, Shankar M, Huberli D, Dhammu H. Wheat variety fact sheets for Western Australia, Department of Agriculture and Food, Western Australia. Bulletin 4881. 2017. ISSN: 1833–7236.
Zhang X, Wu Y, Liu X, Reis S, Jin J, Dragosits U, Gu B. Ammonia emissions may be substantially underestimated in China. Environ Sci Technol. 2017;51:12089–96. https://doi.org/10.1021/acs.est.7b02171 .
Zörb C, Ludewig U, Hawkesford MJ. Perspective on wheat yield and quality with reduced nitrogen supply. Trends Plant Sci. 2018;23:1029–37. https://doi.org/10.1016/j.tplants.2018.08.012 .
Download references
This work was supported by the Newton-Bhabha Fund Project co-funded by the Department of Biotechnology, Ministry of Science and Technology, Government of India (Grant number BT/IN/UK-VNC/43/KV/2015–16) (to RP) and the Biotechnology and Biological Sciences Research Council (BBSRC) of the UK [Grant number BB/J011827/1] (UK). Rothamsted Research and the John Innes Centre received strategic funding from BBSRC and the work forms part of the Designing Future Wheat strategic programme (BB/P016855/1).
Author information
Authors and affiliations.
Division of Plant Physiology, ICAR-Indian Agricultural Research Institute, New Delhi, 110012, India
Sandeep Sharma, Tarun Kumar, Lekshmy S. Nair & Renu Pandey
Division of Plant and Crop Sciences, School of Biosciences, University of Nottingham, Leicestershire, LE12 5RD, UK
M. John Foulkes
Crop Genetics, John Innes Centre, Norwich Research Park, Norwich, NR4 7UH, UK
Simon Orford, Luzie U. Wingen & Simon Griffiths
Division of Genetics, ICAR-Indian Agricultural Research Institute, New Delhi, 110012, India
Anju Mahendru Singh
ICAR-Indian Institute of Wheat and Barley Research, Karnal, Haryana, India
Venkatesh Karnam
National Institute of Plant Biotechnology, Pusa Campus, New Delhi, 110012, India
Pranab Kumar Mandal
Plant Sciences Department, Rothamsted Research, Harpenden, AL5 2JQ, UK
Malcolm J. Hawkesford & Peter R. Shewry
National Institute for Agricultural Botany, Cambridge, CB3 0LE, UK
Alison R. Bentley
Present Address: International Maize and Wheat Improvement Center (CIMMYT), El Batán, Texcoco, Mexico
You can also search for this author in PubMed Google Scholar
Contributions
SS: data collection, formal analysis, writing—original draft, writing—review and editing; TK: data collection, writing—review and editing; RP:—conceptualization, formal analysis, methodology, resources, supervision, fund acquisition, writing—review and editing; MJF, SO, AMS, LUW, LSN, VK, PKM, SG, MJH, PRS, ARB: conceptualization, writing—review and editing; SO, LUW, SG: also provided seed material. All authors read and approved the final manuscript.
Corresponding author
Correspondence to Renu Pandey .
Ethics declarations
Ethics approval and consent to participate.
Not applicable.
Consent for publication
Competing interests.
The authors declare that they have no competing interests.
Additional information
Publisher's note.
Springer Nature remains neutral with regard to jurisdictional claims in published maps and institutional affiliations.
Supplementary Information
Additional file 1: table s1..
Name, country of origin, and pedigree information of parents used to develop RIL lines. All RILs share a common parent ‘Paragon’ (P). Among 195 RILs, 132 were selected from eighteen populations of the wheat landrace nested associated mapping (NAM) panel and 63 RILs were selected from populations with modern cultivars. Table S2. Averaging across two years, date of anthesis (GS65) days after sowing (DAS), and date of physiological maturity (GS91) after GS65 of 195 wheat NAM RILs procured from John Innes Centre, UK and grown in low N field under north Indian condition. Table S3. Properties of soil used for growing wheat in the field. Soil sampling was done before sowing of crops. Table S4. Descriptive statistical analysis of 195 wheat NAM RILs grown in low N soil in the years 2016–17 and 2017–18. Table S5. Phenology, growth and physiological observation of common parent Paragon (average of 2 years) and average of different RILs belonging to each cluster created by Ward’s clustering on the basis of grain yield and NRE. Table S6. Pearson’s correlation coefficient amongst 195 wheat NAM RILs grown in low N field condition. Pooled data for two years (2016–17 and 2017–18) was used for correlation. Abbreviations: AGNa- above ground N uptake at anthesis; LNh- leaf N uptake at harvest; SNh- stem N uptake at harvest; GNh—grain N uptake at harvest; AGNh—above ground N uptake at harvest; AGNpa—above ground N uptake post-anthesis; AGBa—above ground biomass at anthesis; AGBh—above ground biomass at harvest; AGBpa—above ground biomass post-anthesis; TGW – 1000 grain weight; NRE—N remobilization efficiency; NNI—N nutrition index; StemNPI—stem N partitioning index; LeafNPI—leaf N partitioning index; GrainNPI—grain N partitioning index; GPC—grain protein concentration; GPD- grain protein deviation. *, **, *** denoted significant at 0.05, 0.01 and 0.001 probability level respectively. Figure S1. Weather data during wheat growing season in northern India. (A) 2016–17, and (B) 2017–18. Figure S2. The grain protein deviation (GPD) calculated from the regression of grain yield and grain protein concentration. Each data point represents mean GPD of two seasons (2016–17 and 2017–18) for each line. The numbers correspond to the name of RIL group presented in Table S1.
Rights and permissions
Open Access This article is licensed under a Creative Commons Attribution 4.0 International License, which permits use, sharing, adaptation, distribution and reproduction in any medium or format, as long as you give appropriate credit to the original author(s) and the source, provide a link to the Creative Commons licence, and indicate if changes were made. The images or other third party material in this article are included in the article's Creative Commons licence, unless indicated otherwise in a credit line to the material. If material is not included in the article's Creative Commons licence and your intended use is not permitted by statutory regulation or exceeds the permitted use, you will need to obtain permission directly from the copyright holder. To view a copy of this licence, visit http://creativecommons.org/licenses/by/4.0/ . The Creative Commons Public Domain Dedication waiver ( http://creativecommons.org/publicdomain/zero/1.0/ ) applies to the data made available in this article, unless otherwise stated in a credit line to the data.
Reprints and permissions
About this article
Cite this article.
Sharma, S., Kumar, T., Foulkes, M.J. et al. Nitrogen uptake and remobilization from pre- and post-anthesis stages contribute towards grain yield and grain protein concentration in wheat grown in limited nitrogen conditions. CABI Agric Biosci 4 , 12 (2023). https://doi.org/10.1186/s43170-023-00153-7
Download citation
Received : 08 August 2022
Accepted : 24 April 2023
Published : 04 May 2023
DOI : https://doi.org/10.1186/s43170-023-00153-7
Share this article
Anyone you share the following link with will be able to read this content:
Sorry, a shareable link is not currently available for this article.
Provided by the Springer Nature SharedIt content-sharing initiative
- Biomass partitioning
- Low soil nitrogen
- Triticum aestivum
CABI Agriculture and Bioscience
ISSN: 2662-4044
- Submission enquiries: Access here and click Contact Us
- General enquiries: [email protected]
ORIGINAL RESEARCH article
Improving the effects of drought priming against post-anthesis drought stress in wheat (triticum aestivum l.) using nitrogen.
- 1 Key Laboratory of Crop Physiology, Ecology and Production Management, Nanjing Agricultural University, Nanjing, China
- 2 Department of Soil Conservation, Narowal, Pakistan
- 3 Key Laboratory of Crop Cultivation and Tillage, Agricultural College of Guangxi University, Nanning, China
Water and nitrogen (N) deficiencies are the major limitations to crop production, particularly when they occur simultaneously. By supporting metabolism, even when tissue water capacity is lower, nitrogen and priming may reduce drought pressure on plants. Therefore, the current study investigates the impact of nitrogen and priming on wheat to minimize post-anthesis drought stress. Plant morphology, physiology, and biochemical changes were observed before, during, and after stress at the post-anthesis stage. The plants were exposed to three water levels, i.e., well watering (WW), water deficit (WD), and priming at jointing and water deficit (PJWD) at the post-anthesis stage, and two different nitrogen levels, i.e., N180 (N1) and N300 (N2). Nitrogen was applied in three splits, namely, sowing, jointing, and booting stages. The results showed that the photosynthesis of plants with N1 was significantly reduced under drought stress. Moreover, drought stress affected chlorophyll (Chl) fluorescence and water-related parameters (osmotic potential, leaf water potential, and relative water content), grain filling duration (GFD), and grain yield. In contrast, PJWD couple with high nitrogen treatment (N300 kg ha –1 ) induced the antioxidant activity of peroxidase (37.5%), superoxide dismutase (29.64%), and catalase (65.66%) in flag leaves, whereas the levels of hydrogen peroxide (H 2 O 2 ) and superoxide anion radical (O 2 – ) declined by 58.56 and 66.64%, respectively. However, during the drought period, the primed plants under high nitrogen treatment (N300 kg ha –1 ) maintained higher Chl content, leaf water potential, and lowered lipid peroxidation (61%) (related to higher activities of ascorbate peroxidase and superoxide dismutase). Plants under high nitrogen treatment (N300 kg ha –1 ) showed deferred senescence, improved GFD, and grain yield. Consequently, the research showed that high nitrogen dose (N300 kg ha –1 ) played a synergistic role in enhancing the drought tolerance effects of priming under post-anthesis drought stress in wheat.
Introduction
Plant growth and development are greatly associated with the available resources, such as nitrogen, water, and light. Studies have shown that water and nitrogen deficiencies alter numerous morphological and physiological processes in plants ( Wang et al., 2021 ). Water scarcity is viewed as the most limiting factor to plant development and yield ( Siddiqui et al., 2015 ; Jabborova et al., 2021 ). Wheat ( Triticum aestivum L.) is considered to be the largest cultivated cereal crop in the world and is extremely sensitive to drought stress, which regularly occurs at the post-anthesis stage and leads to significant yield losses ( Cossani and Reynolds, 2012 ; Wang et al., 2014a ). Drought stress induced during the post-anthesis stage of the wheat crop either halts grain filling or causes the grain to remain completely unfilled ( Dhanda and Sethi, 2002 ), as grain filling is predominantly a connected source–sink relationship ( Thakur et al., 2010 ). In cereals, photosynthesis of the flag leaf and inflorescence during post-anthesis plays a crucial role in grain development ( Murchie et al., 2002 ). Therefore, improving drought tolerance during post-anthesis in wheat is crucial for sustaining food security in the present global climate scenario.
The recent global climate events are continuously impacting agriculture, threatening global food security. Drought stress is the primary factor affecting plant metabolism and disturbing the photosynthesis machinery ( Ahmad S. et al., 2021 ; Nardino et al., 2022 ). In the process, drought stress reduces the stomata conductance to restrict water losses during evapo-transpiration and reduces the CO 2 in the grass ( Gaikwad et al., 2020 ), subsequently disturbing photosystem II (Fv/Fm) ( Grassi and Magnani, 2005 ). In fact, the effects of drought on wheat crops are multilayered, such as turgor loss, osmotic imbalance, and shrinkage in cell volume, as well as reduced membrane stability and accumulation of ROS ( Batra et al., 2014 ; Liu et al., 2015 ; Ahmad A. et al., 2021 ). Moreover, the failure of plants to scavenge ROS induces oxidative stress, which leads to membrane lipid peroxidation, protein oxidation, hormonal imbalance, and finally, cell death ( Niu et al., 2013 ; Liu et al., 2015 ). Drought tolerance is a complex quantitative trait ( Wu et al., 2022 ), and plants deploy multiple strategies to combat drought ( Ahmad et al., 2019 ). These include osmoprotectants, enzymatic and non-enzymatic antioxidants, biomarkers, and secondary metabolism-related enzymes ( Zeeshan et al., 2020 ; Siddiqui et al., 2021 ; Salam et al., 2022 ).
Previous studies indicated that pre-stress priming had a positive effect on plants by activating faster and more proficient defense mechanisms against the subsequent stress circumstances ( Abid et al., 2016b ; Wang et al., 2018 ). Pre-stress priming, which is known as “stress memory” ( Wang et al., 2018 ), could increase stress tolerance ( Abid et al., 2016b ). Previous experiments showed that induction of drought priming at the vegetative stage and/or pre-anthesis stage enhanced heat and/or drought tolerance during the grain filling stage in Triticum aestivum L. ( Wang et al., 2014a , b ) and enhanced freezing tolerance in wheat at the jointing stage ( Li et al., 2015 ). Similarly, high-temperature stress at the post-anthesis stage was relieved in response to drought priming in wheat, as evident by enhanced photosynthetic activity and mitigation of oxidative stress ( Zhang et al., 2016 ). Moreover, higher net photosynthesis along with dry biomass and grain yield was observed in response to the multiple water-logging priming induced at three different growth stages in wheat ( Li et al., 2011 ). Therefore, inducing priming at different growth stages against the corresponding stresses occurring during post-anthesis was found to be an effective tool for stress mitigation. But it should be noted that drought stress levels change during the vegetative development period of wheat, and therefore the effects of drought priming against post-anthesis drought stress require further investigation. Additionally, previous studies did not investigate the use of high nitrogen fertilizer along with pre-stress priming to enhance drought tolerance.
Nitrogen is a significant underlying part of nucleic acids, proteins, chlorophyll, rubisco, and some hormones, and can decrease drought stress damage by maintaining metabolic activities ( Zhang et al., 2007 ; Ata-Ul-Karim et al., 2016 ). Abid et al. (2016a) suggested that proper nitrogen supply can improve plant drought tolerance in wheat. Studies reported that the utilization of nitrogen fertilizer relieves the negative impact of drought stress ( Saneoka et al., 2004 ; Yang et al., 2012 ). Morgan (1986) suggested that nitrogen-fertilized wheat plants respond faster to drought stress by closing stomata and reducing net photosynthesis. Shabbir et al. (2016) found that a combined foliar spray of additional nitrogen affected the addition of osmoprotectants and the activities of nitrogen assimilation and antioxidant enzymes, thereby improving wheat yield and drought tolerance. Moreover, water limitation and low nitrogen supply are the main limiting factors for wheat yield. It is widely reported that both factors affect leaf–water relationship, chlorophyll fluorescence, and photosynthetic processes, thereby limiting the rate of plant development, inducing early senescence, and shortening the GFD period, thus causing limited grain weight and poor crop productivity ( Madani et al., 2010 ; Mobasser et al., 2014 ). However, Li et al. (2011) revealed that under drought stress, applying nitrogen prevents different grass species from changing their physiological functions. These varying outcomes may be accredited to differences in particular environments, species characteristics, drought stress levels, nitrogen, timing, or different growth phases of the crops. Even under constant water and nitrogen levels, the response of plants to drought stress varies at various phases of crop development ( Ernst et al., 2010 ; Shi et al., 2014 ). In addition, despite its significance, very few studies can completely compare the effects of different levels of drought stress on grain yield traits at different vegetative stages of wheat. Furthermore, the tandem use of high nitrogen fertilizer and priming to understand the plant ecophysiological mechanisms of drought resistance has not been explored. Therefore, an appropriate estimation of the effects of drought priming against post-anthesis drought stress using nitrogen on the physiological activities and yield attributes will provide significant insight into the cultivation of wheat under adverse conditions ( Teixeira et al., 2014 ).
In this study, we hypothesized that nitrogen application and pre-anthesis drought priming might lessen the effects of post-anthesis drought stress in wheat. It was further hypothesized that an appropriate increase in nitrogen application in water deficit conditions would enhance the drought tolerance of the wheat crops.
Materials and methods
Experimental design.
To assess the combined effects of nitrogen and pre-drought priming on the wheat plants during post-anthesis drought stress, a rain cover pot experiment was conducted at the Pailou Experimental Research Station of Nanjing Agricultural University, China (32°040 N, 118°760 E), during the growing period of 2018–2019. Plants were sown at a shallow depth of 3–5 cm in plastic pots, i.e., 25 cm high and 22 cm in diameter, and filled with 10 kg of clay loam air-dried soil (bulk density; 1.29 g cm –3 ), sieved with a 0.5-mm mesh, uniformly mixed, and placed in a shade house under natural light conditions. Meanwhile, at the time of pot filling, 0.5 g of P 2 O 5 and 1.1 g of K 2 O per pot were mixed with soil. Two wheat varieties, i.e., Yangmai-158 (medium gluten) and Yangmai-22 (low gluten), were seeded as experimental materials. Prior to sowing, seeds were disinfected with 1% (v/v) H 2 O 2 and then washed three times using ddH 2 O. Fourteen seeds of each variety were sown in each pot and were arranged in a completely randomized design (CRD) with a factorial arrangement. The experimental design is presented in Figure 1 . At emergence, seven uniform seedlings were left after thinning in all pots for subsequent experimental measurements and analysis. The details of the atmospheric temperature of the experimental area are given in Table 1 .
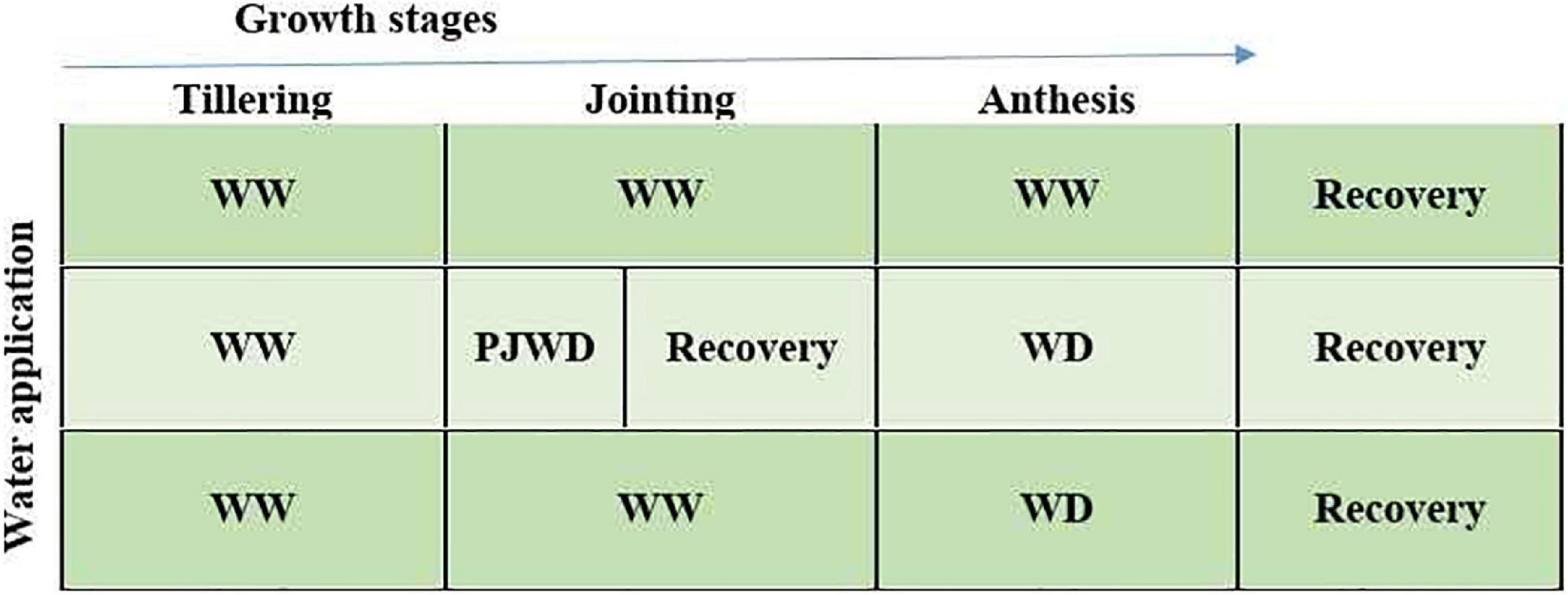
Figure 1. Experimental plan for the study: drought priming (P) was applied at jointing (J) by exposing the plants of two wheat cultivars (Yangmai-158 and Yangmai-22) to moderate drought stress at 55–60% field capacity (FC) for 10 days followed by re-watering. Meanwhile, non-priming plants (WW) were subjected to continued watering at 75–80% FC. At the post-anthesis stage, moderate drought stress at 55–60% FC was applied from 7 to 14 days after post-anthesis followed by re-watering. The treatments during post-anthesis drought stress were assigned as PJWD priming at jointing + post-anthesis drought stress, WD no priming at jointing + post-anthesis drought stress, and WW no priming at jointing + no post-anthesis drought stress. After priming at jointing and application of post-anthesis drought stress treatments, the plants were re-watered directly at 75%–80% FC for recuperation.

Table 1. Average temperatures during growing seasons of 2018–2019.
Treatments and applications
The experiment consisted of two wheat varieties, i.e., Yangmai-158 (YM-158) and Yangmai-22 (YM-22), and three water levels, i.e., well watering (WW), water deficit (WD), and priming at jointing and water deficit (PJWD) at post-anthesis stage. The experiment included two different nitrogen levels, i.e., N180 kg ha –1 (N1) and N300 kg ha –1 (N2). There were 12 treatments and 33 pots as replicates per treatment (2 variety* 3 water level* 2 nitrogen* 33 replications = 396 pots in total). Drought priming was induced at the jointing stage, and irrigation of pots was suspended until a moderate drought stress level from 55 to 60% field capacity (FC) was reached. This moderate drought stress level was maintained for 10 days as priming by compensating for water lost every day. Meanwhile, the control pots were kept on irrigation under well-watered conditions. After the completion of priming, the pots were re-watered to the level of control pots (78–80% FC) until the application ensured post-anthesis drought stress. Seven days after the anthesis appeared, plants were exposed to drought stress (at 30–40% field capacity) and re-watered to 78–80% FC till harvesting after the complication of treatment duration. The soil moisture content of each treatment was measured daily using an HH2 Moisture Meter (Delta-T Devices, Cambridge, United Kingdom). For N treatment, nitrogen fertilizer in the form of urea nitrogen [CO(NH 2 ) 2 ] was applied in three splits: 50% N of each level was applied at the time of sowing, another 30% N at jointing, and another 20% at booting. The quantity of water required for pot irrigation was determined using the procedure suggested by a previous study ( Abid et al., 2016a ).
where W is the quantity of water irrigation, H is the depth of soil, A is the vicinity of pot, Y is the bulk density of the soil, FC1 is the top edge of the needed soil FC, and FC0 is the real soil FC before irrigation.
Plant selection and trait measurement
At the post-anthesis stage, the uniform tillers/spikes from all treatments were tagged at the same time for testing and measurements. The topmost fully expended flag leaves were sampled from the three chosen pots (considered as one replication) of each treatment 1 day before the beginning of drought stress (0 DS), 5 days after drought stress (5 DS), and 5 and 10 days after re-watering (5 DRW and 10 DRW) for the determination of physiological and biochemical parameters. Plants in each pot were utilized only once per sample at each time point and then were discarded. All plant samplings and photosynthesis measurements were performed between 9:00 a.m. and 11:00 a.m. local time. The samples collected for protein assay and other related parameters were kept at –80°C until further determination.
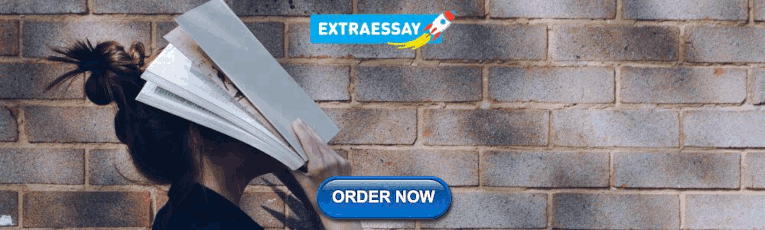
Osmotic potential, leaf water potential, and leaf relative water content
The flag was selected for measurement of leaf relative water content (LRWC) from each treatment in three replications as indicated by a previous study ( Barrs and Weatherley, 1962 ). The LRWC equation is as follows:
where FW indicates the fresh weight of leaf, TW indicates the fresh weight of turgid dipped leaves in distal water after drenching in the darkness for 24 h, and DW indicates the dry weight of leaves after dehydration at 75°C to a stable weight (oven drying).
The osmotic potential was resolved by following the technique of a previous study ( Ribaut and Pilet, 1991 ). The novel completely enlarged leaf was set in the nitrogen fluid at a defrosted point (25°C), and the cell sap liquid was separated by compacting the leaf in a syringe. The osmotic concentration was estimated using the fume osmometer pressure (Wescor Vapor 5600, United States) and determined using the van’t Hoff condition.
The leaves were immobilized in the fixed cover of the sample holder, and pressure was applied until liquid flowed from the uncovered end of the leaf stalk. At this point, careful perusing was noted, which demonstrated that water was held inside the leaf with negative power, expressed as –MPa.
Leaf gas exchange measurements
At the post-flowering stage, IRGA Li-COR portable photosynthesis system (LI-6400 Inc., Lincoln, NE, United States) was used to measure gas exchange measurements in the flag leaf. Photosynthesis was measured at 1,000 μmol photo m –2 s –1 at 25°C and 400 μmol mol –1 CO 2 (Ca). The light saturation net CO 2 assimilation rate (Pn) and stomatal conductivity ( gs ) were determined.
Chlorophyll fluorescence measurements
The measurements of chlorophyll fluorescence were performed using a modulated fluorimeter (FMS2; Hansatech, King’s Lynn, Norfolk, United Kingdom). Prior to measurements, the topmost fully expanded leaf that was uniformly oriented and fully exposed to light was kept in the dark for 30 min, and then exposed to light (∼1,000 m mol photons m –2 s –1 ) to record the ground state fluorescence (F0) and the maximum fluorescence (Fm). At this point, the steady-state fluorescence value (Fs) and maximum fluorescence (Fm’) of the light adaptation level were measured. Next, the actinic light was turned off, and the leaf was illuminated with far-red light for 3 s to determine the minimum fluorescence in the light-adapted state (F0’). Based on these standards, according to the recommendations from Maxwell and Johnson (2000) , the fluorescence was determined using the maximum efficiency of PSII (Fv/Fm) and the effective quantum yield of PSII (ΦPSII).
Chlorophyll and soluble protein content measurements
A handheld SPAD 502 Chl meter (Soil Plant Analysis Development; Minolta, Japan) was utilized to measure the chlorophyll content (SPAD) of flag leaves of each treatment. The SPAD readings were taken from five flag leaves of each pot, averaged, and considered as one replication. Similarly, three replications were taken per treatment, and the averaged values were taken as the chlorophyll content.
To quantify the total soluble protein content, fresh leaves (0.5 g) were ground in sodium phosphate solution (50 mM, pH 7.0). The amalgam was centrifuged at 4,000 × g at 4°C for 10 min. In order to measure the soluble proteins, bovine serum albumin was used to normalize the sample supernatant, and the absorbance value was recorded spectroscopically at 595 nm.
Superoxide production, malondialdehyde content, hydrogen peroxide content, and antioxidant enzyme activity
The O 2 – content was estimated according to the method suggested by Sui et al. (2007) . The H 2 O 2 and malondialdehyde (MDA) contents were determined according to the method by Zheng et al. (2009) . The activity of superoxide dismutase (SOD, EC 1.15.1.1) was assessed as suggested by Yang et al. (2007) , and the activity of peroxidase (POD, EC 1.11.1.7) was determined according to the method by Zheng et al. (2009) . The catalase activity (CAT, EC 1.11.1.6) was assayed using the method of Tan et al. (2008) .
Flag leaf area and weight, grain filling duration, and yield component determination
At the post-anthesis stage, the LI-3000 area meter (Li-Cor. Inc., Lincoln, NE, United States) was used to measure the green flag leaf area during drought stress treatment (WD). The specific leaf weight was calculated as follows:
where WL is the flag leaf weight and AL is the flag leaf area.
When half of the spikes under the treatment had reached grain maturity, the grain filling duration (GFD) (the number of days from blossoming to grain development) was recorded. At maturity, three pots were haphazardly chosen from every treatment to ascertain the number of tillers with spikes, the number of grains/spikes, spike length, the weight of 1,000 grains, grain yield/pot, plant biomass, and drought index (DI). The DI was determined as the proportion of grain yield under drought stress (YD) and grain yield under WW development (YW).
Statistical analysis
An analysis of variance (ANOVA) was performed by utilizing the general linear model (GLM) program to see the effects of various nitrogen levels, priming, and post-flowering drought stress on the physiological, morphological, and enzymatic exercises. The mean values for treatments were compared with the least significant difference (LSD t -test was applied to test the treatment differences.) with a probability of 0.05 (SPSS Inc., Chicago, IL, United States). The figures were drawn using Sigma Plot 10.
Leaf water potential, osmotic potential, and leaf relative water content
The leaf water potential (Ψw), osmotic potential (Ψs), and LRWC of flag leaves were measured at the post-anthesis stage and presented in Figures 2A–F . A decreasing trend of these parameters was observed from 12 to 14 days after anthesis (DAA) in both cultivars. The maximum value (less negative) of Ψw and Ψs was recorded in WW, PJWD, and WD under N2 supplementation. The Ψw, Ψs, and LRWC showed a gradual decrease in drought-stressed plants. Moreover, there was a significant difference in Ψw, Ψs, and LRWC in both N levels dose-dependently and WW, and there was also a significant difference between treatments under PJWD and WD conditions. The Ψw, Ψs, and LRWC values declined further under N1 compared to N2. The effect of drought stress decreased with higher nitrogen levels. For instance, N2 showed high performance as compared to N1 in WW, PJWD, and WD. Furthermore, under the post-anthesis drought stress, Ψw, Ψs, and LRWC were less reduced in primed plants when compared to the non-primed plants in both cultivars. After re-watering, the Ψw, Ψs, and LRWC recovered differently under different water and N levels. The drought-stressed plants showed better recovery in Ψw, Ψs, and LRWC under N2 when compared with N1.
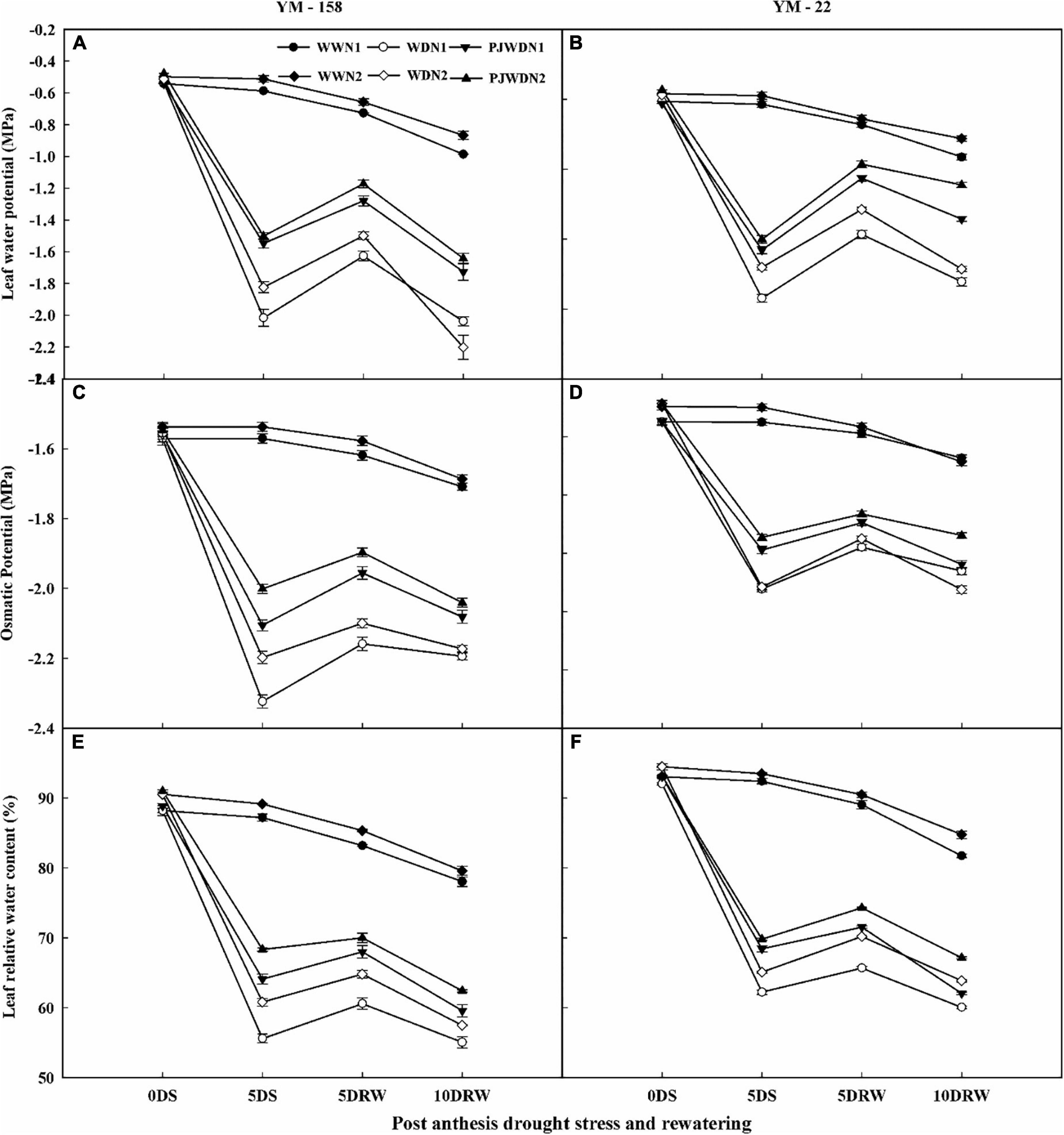
Figure 2. Effects of pre-drought priming on leaf water potential (Ψw), osmatic potential (Ψs), and leaf relative water content (LRWC) in response to post-anthesis drought stress under two nitrogen (N) rates (N1,180 and N2,300) in Yangmai-158 (A,C,E) and Yangmai-22 (B,D,F) wheat cultivars. Treatments: WW (control), no priming at jointing + no post-anthesis drought stress; PJWD, priming at jointing + post-anthesis drought stress; and WD, no priming at jointing + post-anthesis drought stress. Pre-drought priming was completed during the jointing stage, and post-anthesis drought stress was applied from 7 to 14 DAA. The horizontal axis shows the time course of the sampling: 1 day before drought stress (0DS), 5 days after drought stress (5DS), 5 and 10 days after re-watering (5DRW and 10DRW). Each vertical bar above the mean values indicates the standard error of three replicates ( n = 3) by using three-way ANOVA at P < 0.05.
Gas exchange parameters
The level of photosynthesis was maximum from 12 to 14 DAA in both cultivars, and then it started to decrease gradually after 14 days post-anthesis. Analysis showed that the photosynthesis rate (Pn) and stomatal conductance (gs) were reduced under post-anthesis WD and PJWD treatments relative to the WW treatment. However, under WD, the photosynthesis (Pn), and stomatal conductance (gs) were reduced relative to PJWD in YM-158 and YM-22 varieties. Lower Pn and gs were observed in WW, WD, and PJWD conditions under N1 compared to N2 ( Figures 3A–D ). The Pn and gs were influenced more under drought stress than priming treatments when compared to WW, depending on the N level. After re-watering, the PJWD plants showed higher recovery than the WD plants in both cultivars. Furthermore, slow recovery was observed under N1 compared to N2. The results indicated that higher nitrogen and priming have positive effects on Pn and gs under drought conditions.
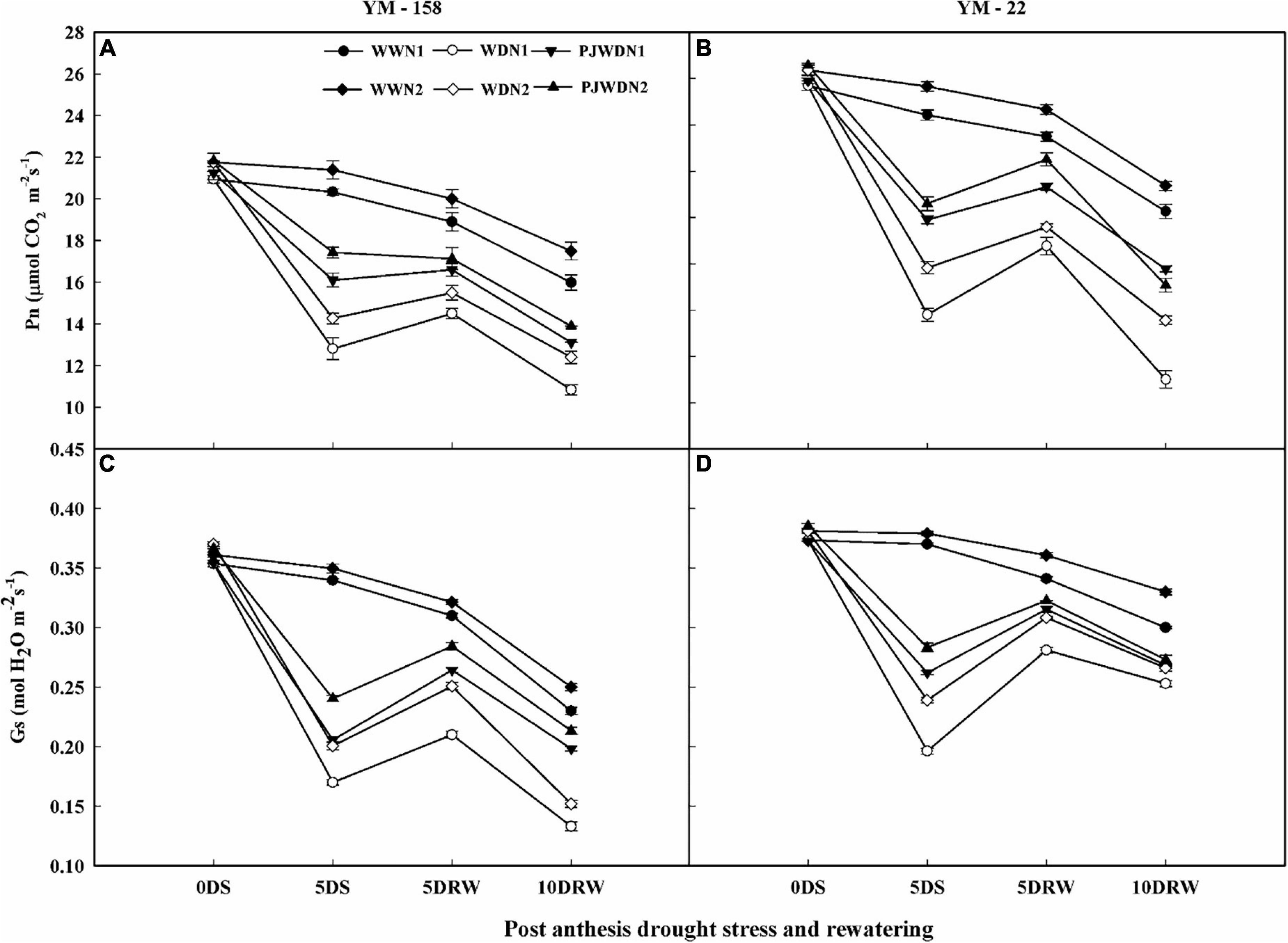
Figure 3. Effects of pre-drought priming on net photosynthetic rate (Pn) and stomatal conductance (gs) in response to post-anthesis drought stress under two nitrogen (N) rates (N1,180 and N2, 300) in Yangmai-158 (A,C) and Yangmai-22 (B,D) wheat cultivars. Treatments: WW (control), no priming at jointing + no post-anthesis drought stress; PJWD, priming at jointing + post-anthesis drought stress; and WD, no priming at jointing + post-anthesis drought stress. Pre-drought priming was completed during the jointing stage, and post-anthesis drought stress was applied from 7 to 14 days after anthesis. The horizontal axis shows the time course of the sampling: 1 day before drought stress (0DS), 5 days after drought stress (5DS), and 5 and 10 days after re-watering (5DRW and 10DRW). Each vertical bar above the mean value indicates the standard error of three replicates ( n = 3) by using three-way ANOVA at P < 0.05.
The decline in Fv/Fm and Φ PSII values started from 12 to 14 DAA. There was a significant alteration in Fv/Fm and Φ PSII rates in N1 and N2 under WW, WD, and PJWD conditions. Drought-stressed WD significantly decreased the Fv/Fm and Φ PSII rates of flag leaves, but PJWD in both cultivars maintained higher Fv/Fm and Φ PSII values than WD. On the other hand, N2 supplement plants showed better stability and reduced effects of drought stress on these parameters than N1 ( Figures 4A–D ). The Fv/Fm and Φ PSII rates in drought-stressed plants also varied from the rates of WW under drought stress and N treatments. The decline in Fv/Fm and Φ PSII was more prominent in WD under N1 compared to N2. After re-watering, the value of Fv/Fm and Φ PSII increased. The primed plants showed higher recovery rates than the non-primed plants in both varieties, and N2 recovered quicker than N1.
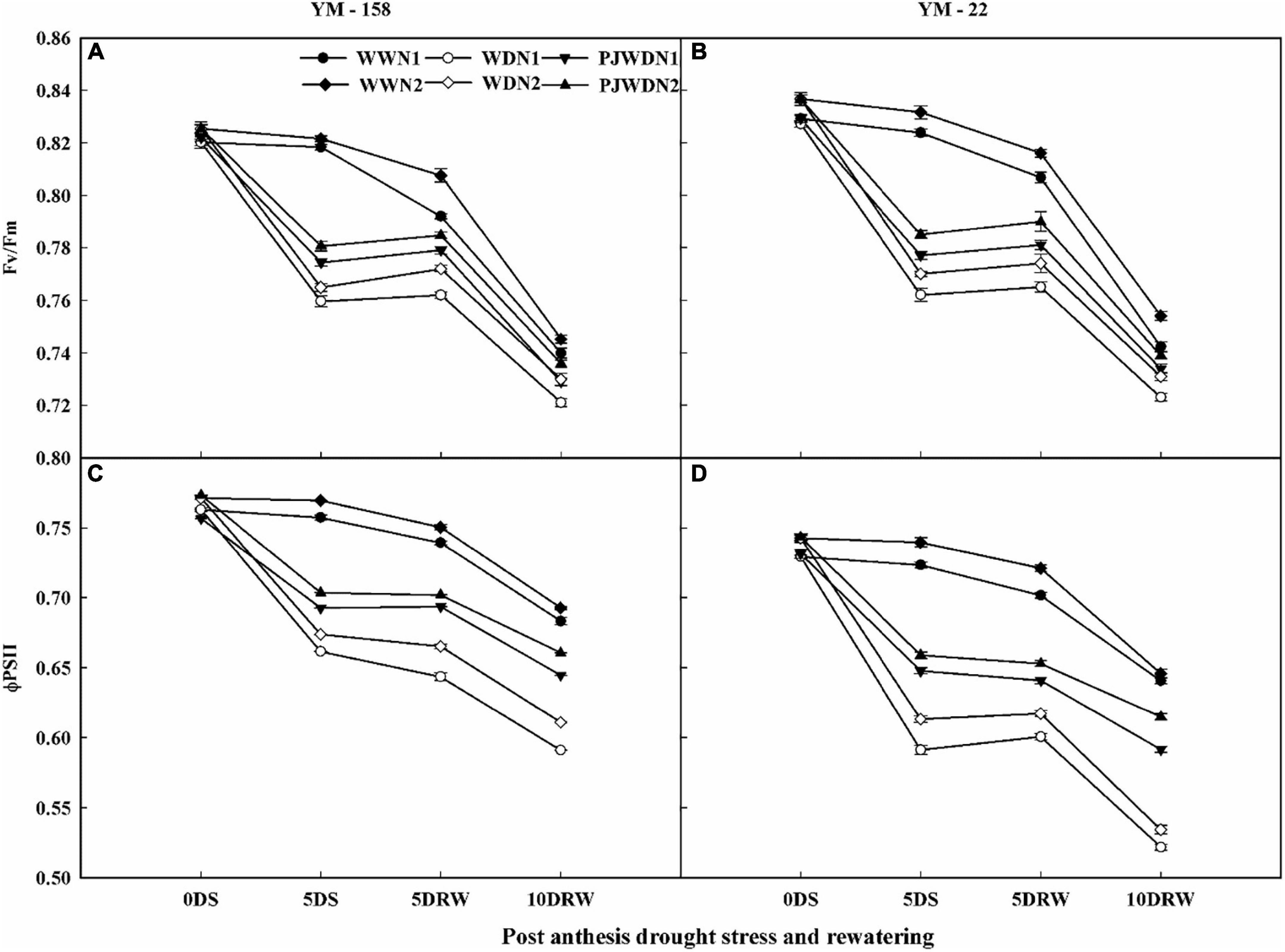
Figure 4. Effects of pre-drought priming on the quantum efficiency of the photochemical reaction in PSII (Fv/Fm) and the quantum yield of the PSII electron transport (ΦPSII), in response to post-anthesis drought stress under two nitrogen (N) rates (N1,180 and N2, 300) in Yangmai-158 (A,C) and Yangmai-22 (B,D) wheat cultivars. Treatments: WW (control), no priming at jointing + no post-anthesis drought stress; PJWD, priming at jointing + post-anthesis drought stress; and WD, no priming at jointing + post-anthesis drought stress. Pre-drought priming was completed during the jointing stage, and post-anthesis drought stress was applied from 7 to 14 days after anthesis. The horizontal axis shows the time course of the sampling: 1 day before drought stress (0DS), 5 days after of drought stress (5DS), and 5 and 10 days after re-watering (5DRW and 10DRW). Each vertical bar above the mean value indicates the standard error of three replicates ( n = 3) by using three-way ANOVA at P < 0.05.
The Chl and soluble protein content showed significant differences in all three water levels and N doses ( Figures 5A–D ). The content of Chl and soluble protein was significantly affected under the WD treatments depending on the strength of WD and N application doses. WD and PJWD significantly decreased the value of Chl and soluble protein in both wheat cultivars compared with WW. The plants under N1 showed a lesser value of Chl and soluble protein content compared to N2. Chlorophyll and soluble protein content under N2 showed more stability in WW, WD, and PJWD treatments compared to N1. After re-watering, the recovery of Chl and soluble protein was slower in N1 than in N2.
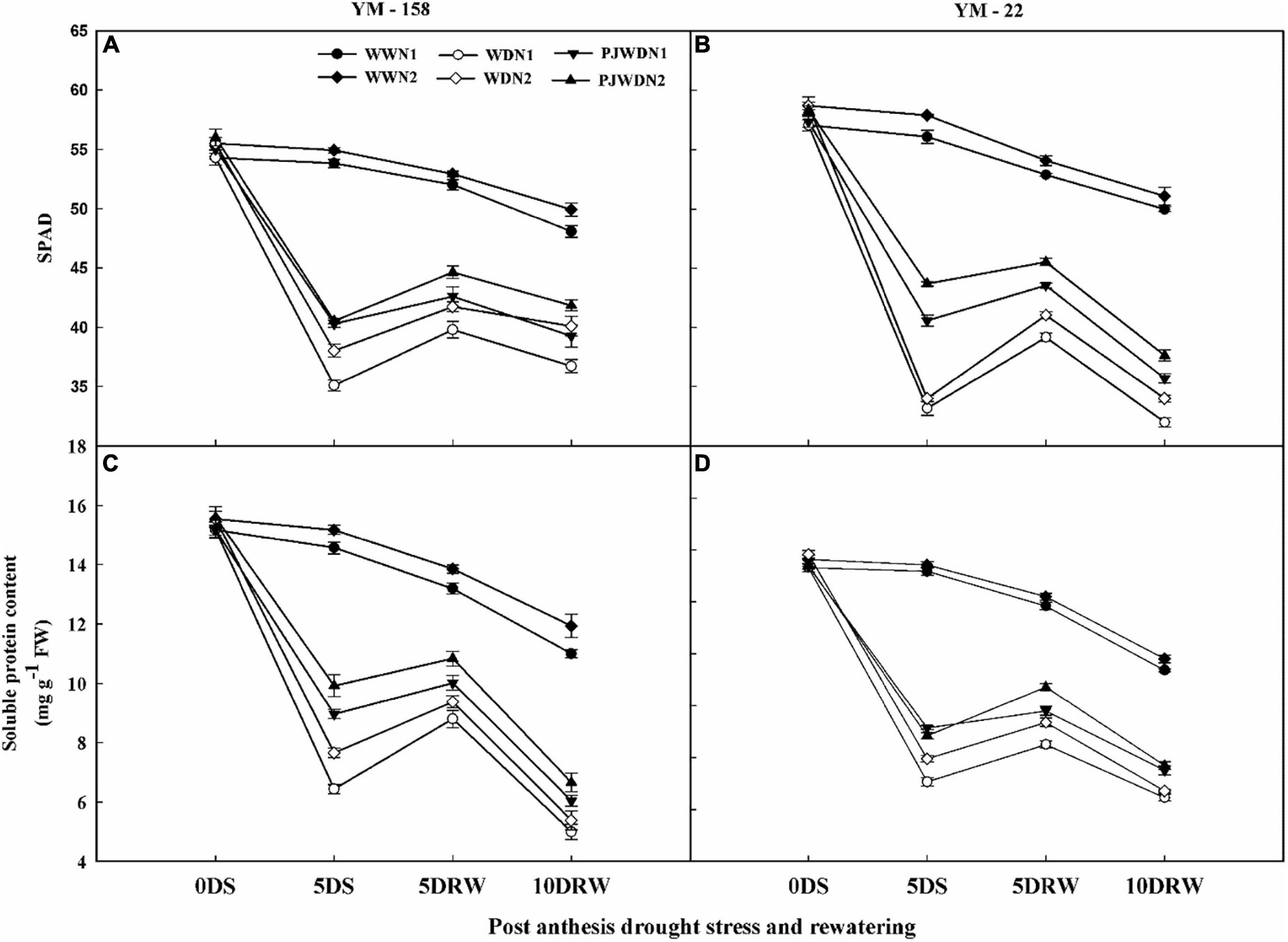
Figure 5. Effects of pre-drought priming on chlorophyll (SPAD value) and soluble protein content in response to post-anthesis drought stress under two nitrogen (N) rates (N1,180 and N2, 300) in Yangmai-158 (A,C) and Yangmai-22 (B,D) wheat cultivars. Treatments: WW (control), no priming at jointing + no post-anthesis drought stress; PJWD, priming at jointing + post-anthesis drought stress; and WD, no priming at jointing + post-anthesis drought stress. Pre-drought priming was completed during the jointing stage, and post-anthesis drought stress was applied from 7 to 14 days after anthesis. The horizontal axis shows the time course of the sampling: 1 days before drought stress (0DS), 5 days after drought stress (5DS), and 5 and 10 days after re-watering (5DRW and 10DRW). Each vertical bar above the mean value indicates the standard error of three replicates ( n = 3) by using three-way ANOVA at P < 0.05.
Superoxide, hydrogen peroxide, and malondialdehyde content
The O 2 – , H 2 O 2 , and MDA content increased rapidly in drought stress and in priming plants when compared to the WW plants at the post-anthesis stage. Significant differences in the concentrations of O 2 – , H 2 O 2 , and MDA were observed among the N levels in the WW, WD, and PJWD conditions dose-dependently. However, the concentration of these parameters decreased with increasing N levels in the WD and PJWD treatments. Therefore, in N2, the magnitude of O 2 – , H 2 O 2 , and MDA increment was less than N1 ( Figures 6A–F ). Compared to drought stress treatment, the primed plants showed less accumulation of O 2 – , H 2 O 2 , and MDA. Similarly, cultivar YM-158 showed higher content of O 2 – , H 2 O 2 , and MDA than that of YM-22, which suggested that YM-158 is more sensitive to drought than YM-22. After re-watering, the accumulation of O 2 – , H 2 O 2 , and MDA decreased, particularly in the primed and N2 plants, when compared with drought-stressed and N1-supplemented plants.
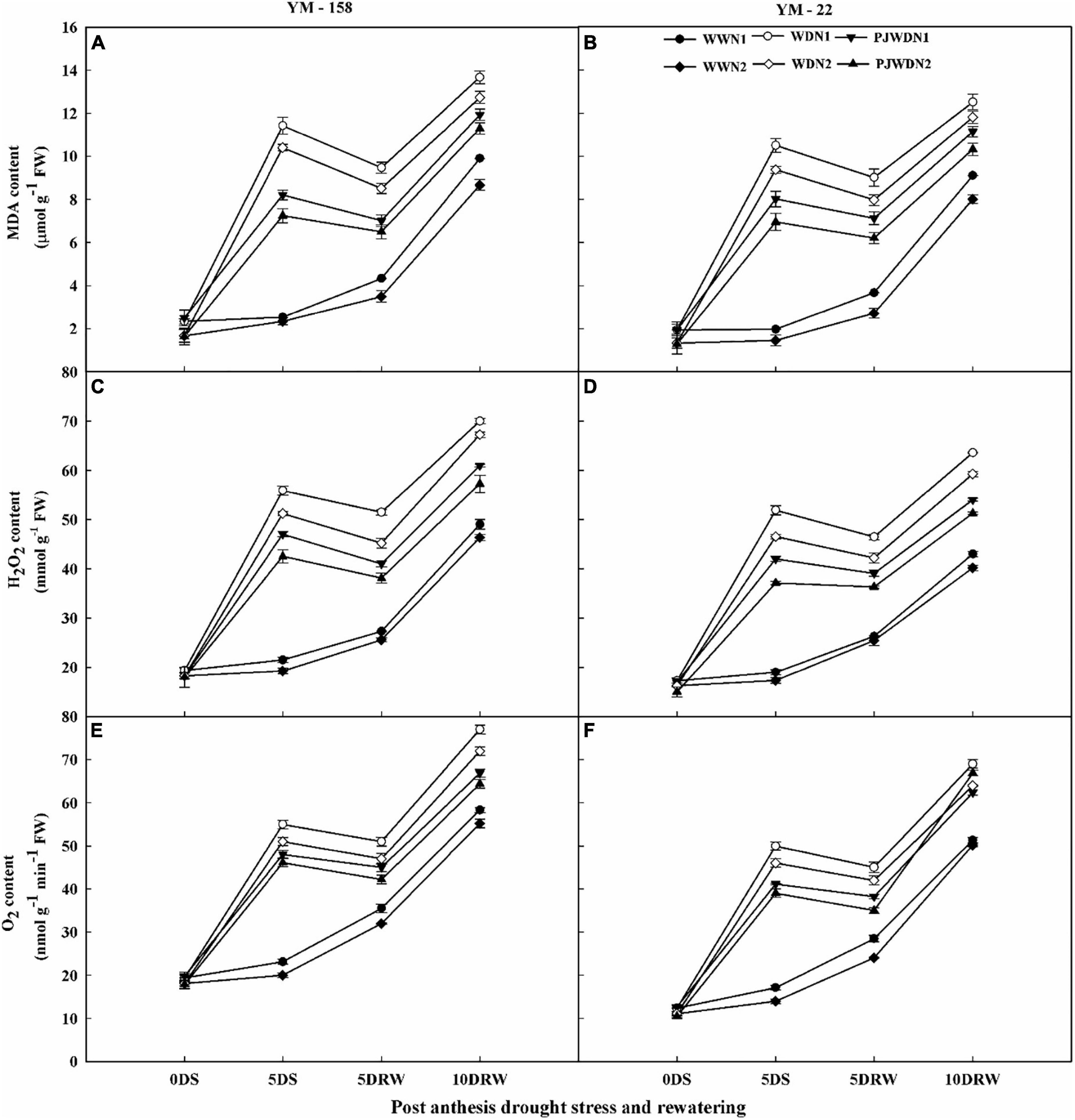
Figure 6. Effects of pre-drought priming on malondialdehyde (MDA), hydrogen peroxide (H 2 O 2 ), and superoxide anion radical (O 2 – ) content in response to post-anthesis drought stress under two nitrogen (N) rates (N1, 180 and N2, 300) in Yangmai-158 (A,C,E) and Yangmai-22 (B,D,F) wheat cultivars. Treatments: WW (control), no priming at jointing + no post-anthesis drought stress; PJWD, priming at jointing + post-anthesis drought stress; and WD, no priming at jointing + post-anthesis drought stress. Pre-drought priming was completed during the jointing stage, and post-anthesis drought stress was applied from 7 to 14 days after anthesis. The horizontal axis shows the time course of the sampling: 1 day before drought stress (0DS), 5 days after drought stress (5DS), and 5 and 10 days after re-watering (5DRW and 10DRW). Each vertical bar above the mean value indicates the standard error of three replicates ( n = 3) by using three-way ANOVA at P < 0.05.
Activities of anti-oxidative enzymes
The POD, SOD, and CAT activities were significantly reduced under drought stress and priming treatments compared to the WW treatment ( Figures 7A–F ). There were significant differences in SOD, POD, and CAT activities between different N doses and water levels. In the WD condition, the POD, SOD, and CAT showed significant differences. In contrast, nitrogen treatment dose-dependently increased the activities of SOD, POD, and CAT in primed plants under drought stress treatment. After re-watering, the N2 plants recovered quickly when compared to N1 plants. The maximum value of all of these parameters was observed in the control treatment with N2. Under post-anthesis drought stress, the SOD, POD, and CAT activities slightly declined in primed plants when compared to the non-primed plants in both cultivars.
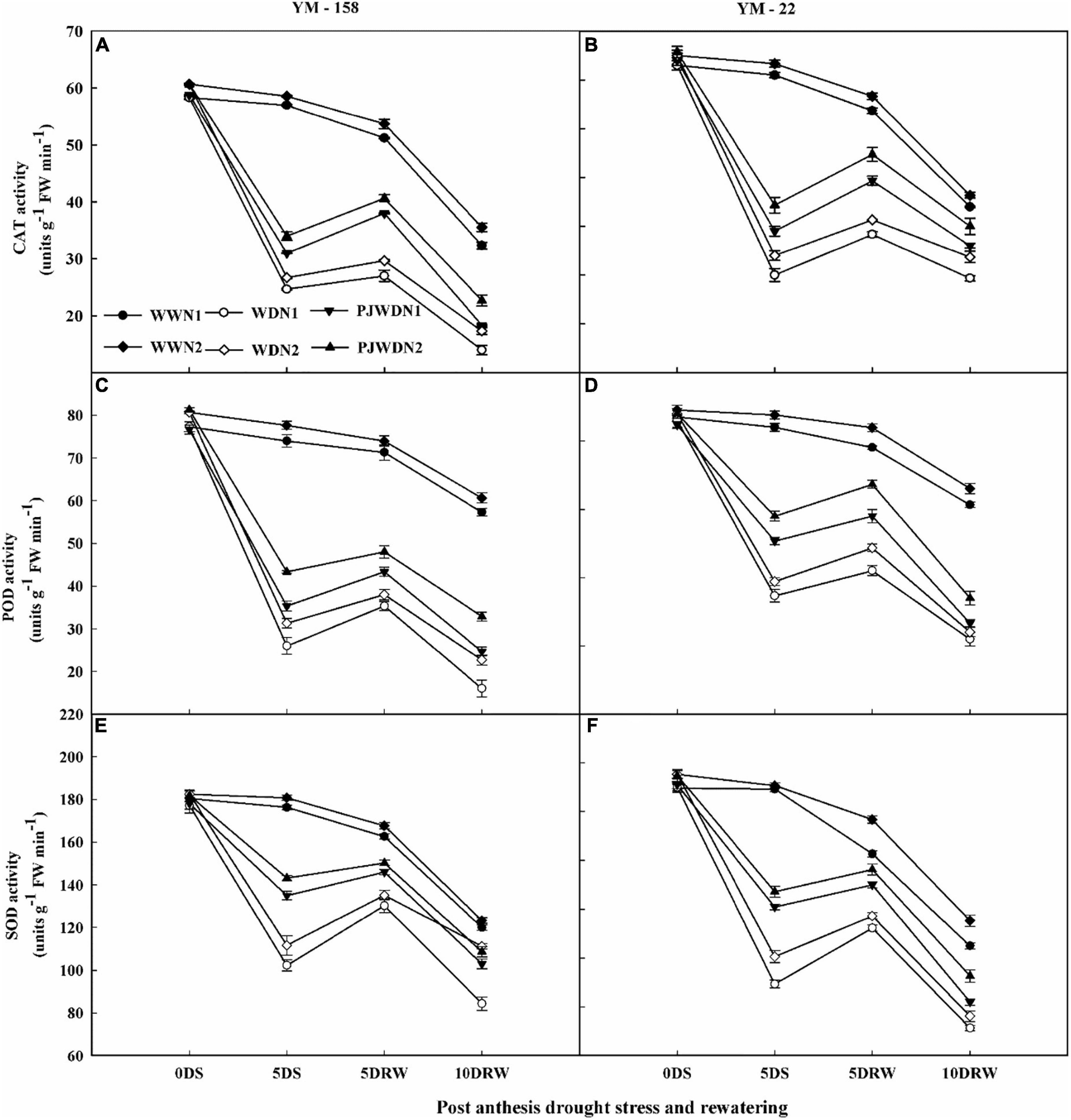
Figure 7. Effects of pre-drought priming on catalase (CAT), peroxidase (POD), and superoxide dismutase (SOD) content in response to post-anthesis drought stress under two nitrogen (N) rates (N1, 180 and N2, 300) in Yangmai-158 (A,C,E) and Yangmai-22 (B,D,F) wheat cultivars. Treatments: WW (control), no priming at jointing + no post-anthesis drought stress; PJWD, priming at jointing + post-anthesis drought stress; and WD, no priming at jointing + post-anthesis drought stress. Pre-drought priming was completed during the jointing stage, and post-anthesis drought stress was applied from 7 to 14 days after anthesis. The horizontal axis shows the time course of the sampling: 1 day before drought stress (0DS), 5 days after drought stress (5DS), and 5 and 10 days after re-watering (5DRW and 10DRW). Each vertical bar above the mean value indicates the standard error of three replicates ( n = 3) by using three-way ANOVA at P < 0.05.
Flag leaf area and specific leaf weight
In the post-flowering stage, during the drought stress period, the flag leaf area and specific leaf weight of YM-22 were higher than those of YM-158 under WW, WD, and PJWD conditions ( Figures 8A,B ). The drought stress decreased flag leaf area and weight, while nitrogen and priming improved the flag leaf area and weight. The minimum flag leaf area and weight were observed in N1 under the WD condition. The maximum flag leaf area was recorded in WW with N2 in both cultivars. PJWD enhanced the flag leaf area and specific leaf weight more than WD. These outcomes showed that pre-anthesis PJWD treatment could bring a superior change in leaf morphology under post-anthesis WD conditions, supporting the accumulation of photosynthetic products.
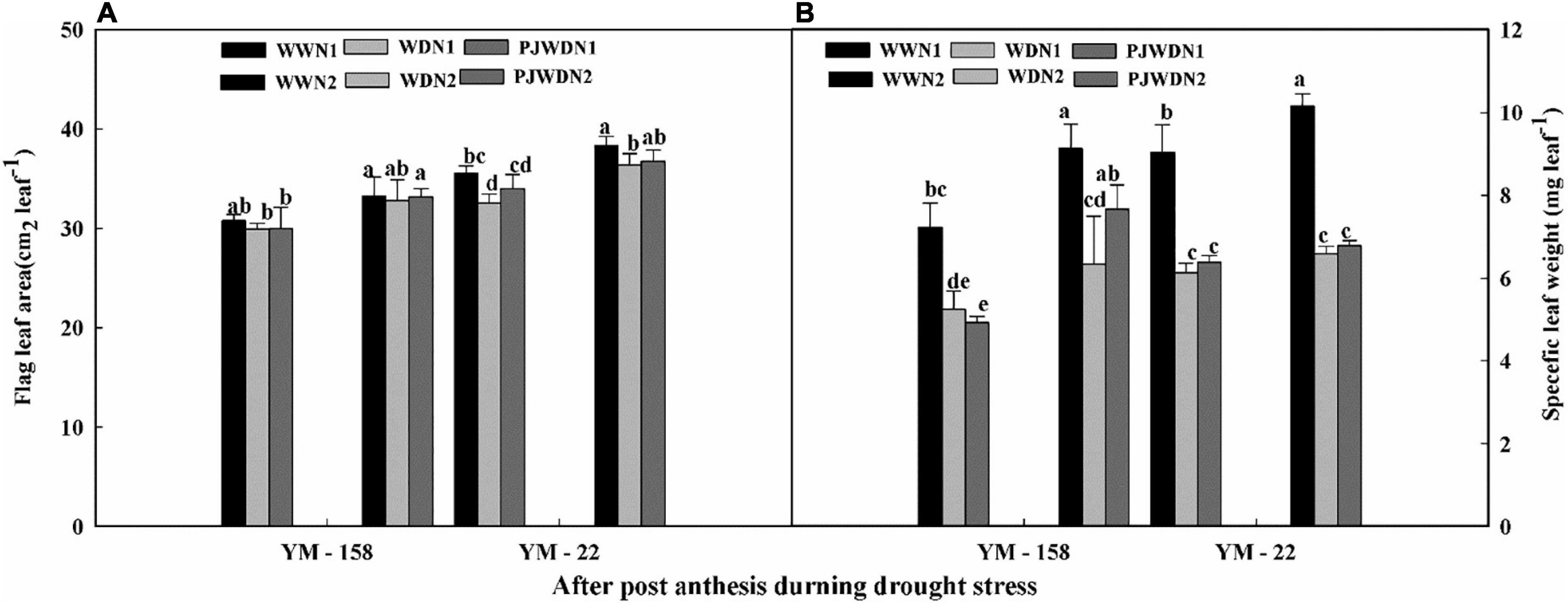
Figure 8. Effects of pre-drought priming on flag leaf area and specific leaf weight in response to post-anthesis drought stress under two nitrogen (N) rates (N1, 180 and N2, 300) in Yangmai-158 and Yangmai-22 (A) , and Yangmai-158 and Yangmai-22 (B) wheat cultivars. Treatments: WW, (control) no priming at jointing + no post-anthesis drought stress; PJWD, priming at jointing + post-anthesis drought stress; and WD, no priming at jointing + post-anthesis drought stress. Pre-drought priming was completed during the jointing stage, and post-anthesis drought stress was applied from 7 to 14 days after anthesis. Each vertical bar above the mean value indicates the standard error of three replicates ( n = 3) by using three-way ANOVA at P < 0.05.
Grain filling duration, grain yield characteristics, and related parameters
The drought stress treatment produced significant changes in GFD, spikes pot –1, grains spike –1 , spike length, biomass pot –1 , thousand-grain weight, and grain yield pot –1 ( Table 2 ). However, drought priming lessened the loss caused by post-anthesis drought stress. Low N aggravated the effects of drought stress on these attributes. The level of grain yield reduction increased with increasing drought and N insufficiency. Drought-primed plants indicated less reduction in grain yield when compared to the non-primed plants in both cultivars under normal water conditions. Similarly, the grain yield reduced less under N2, which resulted in a more prominent drought index (DI) for drought stress treatments. Considerably higher DI was noted in N2 (PJWD) and lower DI was seen in N1 (WD). The impacts of drought stress treatments and N amount on grain yield were assigned to their remarkably similar effects on spikes pot –1 , grains spike –1 , spike length, biomass pot –1 , and thousand-grain weight under N1 drought stress conditions. The water level and N showed significant effects on the spikes pot –1 . N2 showed improved results compared to N1. Under WW, the number of grains spike –1 was better than WD and PJWD, whereas the number of grains spike –1 increased with an increasing level of nitrogen. Spike length significantly decreased as a response to PJWD and WD, and nitrogen content also affected the spike length. The thousand-grain weight was also influenced by drought stress and nitrogen level. However, the response to nitrogen was different than the water level and increased the thousand-grain weight. Furthermore, biomass at maturity decreased significantly due to post-anthesis drought stress and priming when compared to WW treatment, and its reduction was also greater in N1 compared to N2. Therefore, it can be said that a high dose of nitrogen N2 and priming before anthesis can reduce the impact of post-anthesis drought stress and increase drought resistance in plants.
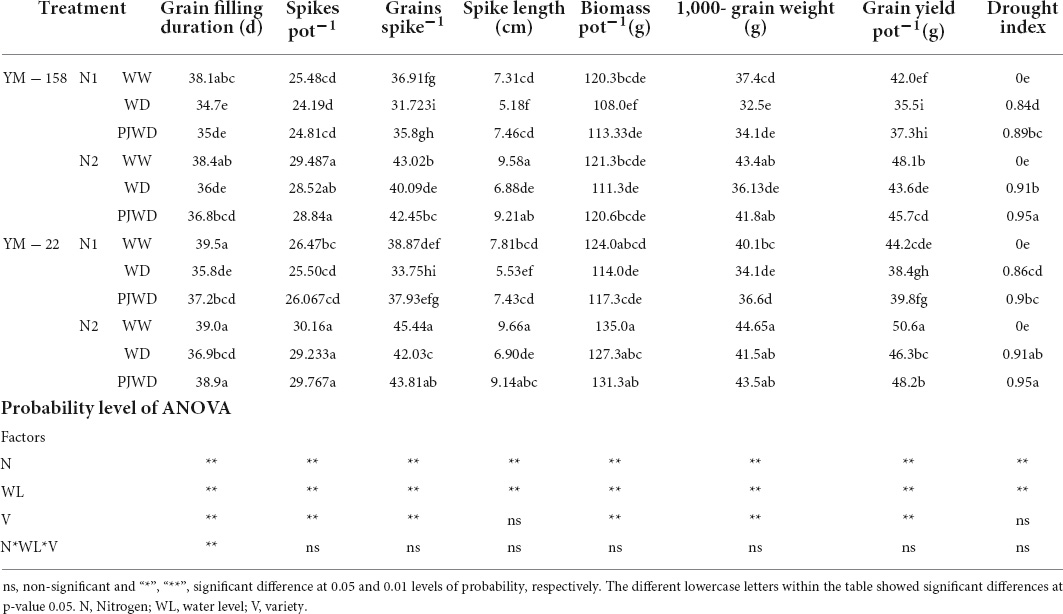
Table 2. Effects of pre-drought priming and nitrogen on grain yield and its components under post-anthesis drought stress in Yangmai-158 (YM-158) and Yangmai-22 (YM-22) wheat cultivars.
The ability of the wheat plant to counteract drought stress in the post-anthesis stage varied with the variation in its treatments with priming and N level ( Abid et al., 2016a ). The results of the present study showed that drought stress reduced plant growth, while higher N application and pre-anthesis moderate drought stress (termed as priming) eased the negative effects of drought stress. In addition, under drought stress, stomatal conductance was decreased, which led to reduced photosynthesis when compared with other treatments. LRWC is the main characteristic that shows plant water level, and when LRWC drops, it causes stomatal closure and affects the availability of CO 2 during photosynthesis under water deficit conditions ( Moshelion et al., 2015 ). In this study, higher Ψw, Ψs, and LRWC values were observed under higher N application and pre-anthesis drought priming in response to drought stress when compared with non-primed plants, indicating that priming and N application trigger leaf activity that enables plants to continue normal functioning under drought stress conditions and helps plants to recover quickly once the drought is over, as demonstrated by the re-watering of the primed plants. Wang et al. (2014a , b) observed higher LRWC and stomatal conductance in the primed plants than in non-primed plants under drought stress conditions in wheat. Similarly, higher N application increased the stomatal conductance and leaf characteristics relative to low N application in drought-stressed plants, suggesting that under low N conditions, the leaf water potential would be decreased, which limits cell growth. As previously mentioned, reduced Ψw under abiotic stresses is the major source of decreased accumulation of dry matter and grain yield ( Gepstein and Glick, 2013 ). Our result showed that under drought conditions, the osmotic potential and LRWC in primed plants in both varieties were higher than those in the non-primed plants. These findings confirmed that drought-primed plants could maintain good water status by better controlling the osmotic potential to cope with drought stress after flowering.
In this study, the decrease in Chl content under drought stress might be the reason for the decline of the photochemical activity of chloroplast, which ultimately reduces photosynthetic activity ( Moshelion et al., 2015 ). However, compared with the non-primed plants and N1 supplementation, the primed and N2-supplemented plants restored the photochemical activities to some extent. It is worth noting that the obvious effect of limited N supply on the droughted plants is the decrease of the Chl and Rubisco contents ( Abid et al., 2016a ). As we know from the previous research, the larger part of N is stored in enzymes, such as Rubisco (which is the main source of the N recycling), and is a necessary component of enzymes involved in the photosynthetic process ( Abid et al., 2016a ). Previously, similar effects of nitrogen limitation on Rubisco concentration and photosynthesis were pointed out in rose plants by Gonzalez-Real and Baille (2000) , as well as in rice and wheat plants ( Makino, 2011 ) . Under severe drought stress, the carboxylation efficiency of Rubisco was incredibly reduced, and Rubisco acts as an oxygenase rather than a carboxylase, where it can reduce the carbon assimilation of photosynthesis ( Makino, 2011 ). N is the main part of Chl and proteins, influencing the entire metabolism of plants under drought stress. Grassi and Magnani, (2005) found that adequate N content partially restored the photosynthetic biochemistry compared to limited N availability. Previous studies have reported that when the drought stress level intensifies, it reduces the leaf area, which subsequently affects photosynthetic pigmentation and reduces stomatal conductance and transpiration rate ( Ahmad et al., 2022 ). In this manner, supporting the plant’s water status and stomatal opening under high N is important to maintaining the conductance of leaf to CO 2 , photosynthetic responses, and electron transfer ( Liu et al., 2013 ).
MDA is the main product of lipid peroxidation, which reflects the degree of damage to the plant photosynthetic functions under adverse conditions ( Zeeshan et al., 2020 , 2021 ). Severe drought stress under low nitrogen application causes excessive accumulation of MDA. The excessive accumulation of MDA triggers early leaf senescence, causes degradation of chlorophyll and macromolecules, and increases cell membrane permeability, which eventually largely affect the development of grains ( Zeeshan et al., 2020 ). We reported higher MDA and ROS accumulation in response to N1-supplemented and droughted plants (DS) when compared with N2-supplemented and primed plants (PJWD) under the same conditions. The strict control of ROS and MDA production and accumulation is to plant defense mechanisms and is a necessary phenomenon under unfavorable conditions because the excessive accumulation of these cellular toxicants can cause cell death, thereby decreasing plant growth and development ( Bieker and Zentgraf, 2013 ).
In order to cope with oxidative damage and keep a balance between the production and scavenging of reactive oxygen species and restoration of metabolic homeostasis, plants have to evolve a well-established antioxidant enzyme system ( Najafi et al., 2021 ; Salam et al., 2022 ). A study has found a strong correlation between oxidative stress caused by drought and the activities of antioxidant enzymes ( Adrees et al., 2020 ). The antioxidative enzyme system of plants, mainly comprising SOD, POD, and CAT, has a prominent role in the mitigation of oxidative stress ( Szalai et al., 2009 ). Our study reported elevated activity of SOD, POD, and CAT in flag leaves in response to the PJWD and higher application of N (N2) over non-primed plants supplemented with limited N (N1). These results suggest that the observed increase in SOD and POD activities would compensate for the accumulation of ROS. In addition, the increase in CAT activity would lead to scavenging the H 2 O 2 , thereby reducing oxidative damage to the membrane. SOD is a key enzyme for the scavenging of reactive oxygen species and conversion of O 2 − to H 2 O 2 . Finally, CAT and POD subsequently scavenge the H 2 O 2 produced due to dismutation and convert it into H 2 O and O 2 using ascorbate in the process ( Khan et al., 2020 ). This study recommends that priming and high doses of N can increase the antioxidant capacity after flowering to reduce the damage caused due to oxidative stress, which can be advantageous in improving the physiological activity of plant leaves. Under higher nitrogen application, the higher ROS detoxification by antioxidant systems in drought-stressed plants may help in protecting photosynthesis. Wang et al. (2014a , b) found higher antioxidant activities and lower accumulation of ROS and MDA in pre-stress primed plants over the non-primed plants under droughted conditions in wheat at different growth stages. Furthermore, Saneoka et al. (2004) observed that N nutrition promotes the drought tolerance of bent grass by stopping cell membrane damage, reducing MDA accumulation, and improving osmoregulation in Agrostis palustris Huds.
Under the droughted condition, crops alter their phenophases: they trigger early anthesis and early maturity in order to avoid stressful conditions, and this alteration is considered vital for maintaining higher grain yield, particularly in cereal ( Chaves et al., 2002 ). This alteration could shift the post-anthesis stage for suitable conditions for leaf photosynthesis and grain filling in wheat ( Fan et al., 2017 ). In the current study, GFD was reduced under the WD condition relative to other treatments, which in turn decreased the grain weight. Previously, Lv et al. (2021) mentioned that in wheat crops, both grain filling rate (GFR) and GFD have a direct impact on grain weight. Similarly, Wu et al. (2011) observed that during the vegetative growth stage, the drought stress alone and/or limited N supply significantly reduced the grain weight and hindered the yield. In the current study, upon supplementation with high N treatment (N2), plants produced more grains and increased plant green canopy, resulting in elevated photosynthetic activity and a high carbon accumulation. On the other hand, droughted plants (WD) under low nitrogen supplementation produced fewer grains and a smaller canopy, resulting in decreased photosynthetic activity. According to an estimation, about 30–50% of the photosynthetic products required for wheat grain filling are delivered through the current photosynthesis during grain filling, so the “keep green” feature can extend the duration of its availability ( Inoue et al., 2004 ; Abid et al., 2016c ). In addition, the ability of the PJWD plants to maintain a higher photosynthetic rate and chlorophyll content may be the ultimate cause for the increase in grain filling rate and duration, and ultimately higher grain yield as compared to the WD plants. The grain filling rate and duration are the key factors that determine the final grain weight, and the final grain weight is crucial for final grain yield ( Altenbach, 2012 ). Therefore, compared with WD plants at post-anthesis, the PJWD plants maintained a higher sink strength, had satisfactory translocation, and received grain reserves, confirming the successful development of grains, and limiting their severe dry weight and grain yield decline.
Furthermore, the PJWD plants efficiently prevented water loss in grains under drought conditions, which might avoid the vascular physiochemical gap within the grain. As the endosperm and embryo dehydration could limit the metabolism of obtained assimilates, the hydraulic response of the developing grain to drought could have a direct impact on filling quality ( Egli and TeKrony, 1997 ; Gupta et al., 2011 ). Under unfavorable environmental conditions, particularly at the post-anthesis stage, plant physiological activities can be reduced and affect the grain yield. Therefore, to face the unfavorable conditions and reduce yield losses, the application of N and priming are needed before post-anthesis drought stress. A higher application of N (N2) along with PJWD plants produced notably higher grain yield and yield-related parameters when compared to remaining treatments except WW. Taken together, the tandem application of higher N with pre-anthesis drought priming can reduce the drought stress on the wheat plants at the post-anthesis stage and can improve the yield of wheat in response to drought stress.
The results of this study indicated that wheat plants pre-exposed to moderate drought stress (priming) and an adequate supply of nitrogen have reduced adverse effects of drought stress after anthesis. Nitrogen and priming had significant effects on wheat’s physiological activities and grain yield. The study found that high nitrogen and priming before anthesis induced greater photosynthetic capacity by expanding the green flag leaf area, elevating the chlorophyll and soluble protein content, increasing sink strength, and increasing the GFD, thereby increasing the grain weight and yield. Furthermore, high nitrogen and priming also increased antioxidant capacity and reduced oxidative stress and MDA accumulation. Drought priming in the early growth stages (especially during the jointing period) highlighted the importance of drought timing and was confirmed to be the best approach to trigger the plants to initiate an effective tolerance mechanism against drought stress. Agriculture will face severe water shortage in the future, and hence to face the challenge of water scarcity, we should not only rely on breeding new cultivars that can tolerate water scarcity, but also invent crop management techniques that can increase crop potential to achieve sustainable crop production.
Data availability statement
The original contributions presented in this study are included in the article/supplementary material, further inquiries can be directed to the corresponding author.
Author contributions
AU, ZT, and TD conceived and organized the experiment. AU conducted the experiment, collected the samples, analyzed the data, and wrote the draft. LX, KL, CS, AK, and YJ helped in plant sampling and data analysis. MA and MZ critically revised the manuscript. TD acquired the funding. All authors contributed to the article and approved the submitted version.
This work was supported by the National Key R&D Program of China (grant no. 2017YFD0301204) and the National Natural Science Foundation of China (grant nos. 31471443 and 31501262). We also acknowledge the support from the Jiangsu Collaborative Innovation Center for Modern Crop Production (JCIC-MCP).
Acknowledgments
We appreciate and acknowledge the effort of K. G. Daniels from Cell Design Institute, Department of Cellular and Molecular Pharmacology, University of California, San Francisco, CA 94158, United States, for revising and proofreading this manuscript.
Conflict of interest
The authors declare that the research was conducted in the absence of any commercial or financial relationships that could be construed as a potential conflict of interest.
Publisher’s note
All claims expressed in this article are solely those of the authors and do not necessarily represent those of their affiliated organizations, or those of the publisher, the editors and the reviewers. Any product that may be evaluated in this article, or claim that may be made by its manufacturer, is not guaranteed or endorsed by the publisher.
Abid, M., Tian, Z., Ata-Ul-Karim, S. T., Liu, Y., Cui, Y., Zahoor, R., et al. (2016b). Improved tolerance to post-anthesis drought stress by pre-drought priming at vegetative stages in drought-tolerant and-sensitive wheat cultivars. Plant Physiol. Biochem. 106, 218–227. doi: 10.1016/j.plaphy.2016.05.003
PubMed Abstract | CrossRef Full Text | Google Scholar
Abid, M., Tian, Z., Ata-Ul-Karim, S. T., Cui, Y., Liu, Y., Zahoor, R., et al. (2016a). Nitrogen nutrition improves the potential of wheat ( Triticum aestivum L.) to alleviate the effects of drought stress during vegetative growth periods. Front. Plant Sci. 7:981. doi: 10.3389/fpls.2016.00981
Abid, M., Tian, Z., Ata-Ul-Karim, S. T., Wang, F., Liu, Y., Zahoor, R., et al. (2016c). Adaptation to and recovery from drought stress at vegetative stages in wheat ( Triticum aestivum ) cultivars. Funct. Plant Biol 43, 1159–1169. doi: 10.1071/FP16150
Adrees, M., Khan, Z. S., Ali, S., Hafeez, M., Khalid, S., ur Rehman, M. Z., et al. (2020). Simultaneous mitigation of cadmium and drought stress in wheat by soil application of iron nanoparticles. Chemosphere 238:124681. doi: 10.1016/j.chemosphere.2019.124681
Ahmad, A., Aslam, Z., Naz, M., Hussain, S., Javed, T., Aslam, S., et al. (2021). Exogenous salicylic acid-induced drought stress tolerance in wheat ( Triticum aestivum L.) grown under hydroponic culture. PLoS One 16:e0260556. doi: 10.1371/journal.pone.0260556
Ahmad, S., Kamran, M., Ding, R., Meng, X., Wang, H., Ahmad, I., et al. (2019). Exogenous melatonin confers drought stress by promoting plant growth, photosynthetic capacity and antioxidant defense system of maize seedlings. PeerJ 7:e7793. doi: 10.7717/peerj.7793
Ahmad, S., Muhammad, I., Wang, G. Y., Zeeshan, M., Yang, L., Ali, I., et al. (2021). Ameliorative effect of melatonin improves drought tolerance by regulating growth, photosynthetic traits and leaf ultrastructure of maize seedlings. BMC Plant Biol 21:368. doi: 10.1186/s12870-021-03160-w
Ahmad, S., Wang, G. Y., Muhammad, I., Chi, Y. X., Zeeshan, M., Nasar, J., et al. (2022). Interactive effects of melatonin and nitrogen improve drought tolerance of maize seedlings by regulating growth and physiochemical attributes. Antioxidants 11:359. doi: 10.3390/antiox11020359
Altenbach, S. B. (2012). New insights into the effects of high temperature, drought and post-anthesis fertilizer on wheat grain development. J. Cereal Sci. 56, 39–50. doi: 10.1016/j.jcs.2011.12.012
CrossRef Full Text | Google Scholar
Ata-Ul-Karim, S. T., Liu, X., Lu, Z., Yuan, Z., Zhu, Y., and Cao, W. (2016). In-season estimation of rice grain yield using critical nitrogen dilution curve. Field Crops Res. 195, 1–8. doi: 10.1016/j.fcr.2016.04.027
Batra, N. G., Sharma, V., and Kumari, N. (2014). Drought-induced changes in chlorophyll fluorescence, photosynthetic pigments, and thylakoid membrane proteins of Vigna radiata . J. Plant Interact 9, 712–721.
Google Scholar
Barrs, H. D., and Weatherley, P. E. (1962). A re-examination of the relative turgidity technique for estimating water deficits in leaves. Aus. J. Biol. Sci 15, 413–428. doi: 10.1071/BI9620413
Bieker, S., and Zentgraf, U. (2013). Plant senescence and nitrogen mobilization and signaling. Senescence Senescence Relat. Disord. 104, 53–83. doi: 10.5772/54392
Chaves, M. M., Pereira, J. S., Maroco, J., Rodrigues, M. L., Ricardo, C. P., Osório, M. L., et al. (2002). How plants cope with water stress in the field? Photosynthesis and growth. Ann. Bot. 89, 907–916. doi: 10.1093/aob/mcf105
Cossani, C. M., and Reynolds, M. P. (2012). Physiological traits for improving heat tolerance in wheat. Plant Physiol. 160, 1710–1718. doi: 10.1104/pp.112.207753
Dhanda, S. S., and Sethi, G. S. (2002). Tolerance to drought stress among selected Indian wheat cultivars. J. Agri. Sci. 139, 319–326. doi: 10.1017/S0021859602002526
Egli, D. B., and TeKrony, D. M. (1997). Species differences in seed water status during seed maturation and germination. Seed Sci. Res. 7, 3–12. doi: 10.1017/S0960258500003305
Ernst, L., Goodger, J. Q., Alvarez, S., Marsh, E. L., Berla, B., Lockhart, E., et al. (2010). Sulphate as a xylem-borne chemical signal precedes the expression of ABA biosynthetic genes in maize roots. J. Exp. Bot. 61, 3395–3405. doi: 10.1093/jxb/erq160
Fan, Y., Tian, Z., Yan, Y., Hu, C., Abid, M., Jiang, D., et al. (2017). Winter night-warming improves post-anthesis physiological activities and sink strength in relation to grain filling in winter wheat ( Triticum aestivum L.). Front. Plant Sci. 8:992. doi: 10.3389/fpls.2017.00992
Gaikwad, P. N., Sidhu, G. S., Gahukar, S. J., Kharade, S. J., Chavan, R. S., and Bhojane, P. N. A. (2020). Review: advances in draught stress tolerance in wheat ( Triticum aestivum L.). Int. J. Curr. Microbiol. App. Sci. 9, 2873–2884. doi: 10.20546/ijcmas.2020.905.330
Gepstein, S., and Glick, B.R. (2013) Strategies to ameliorate abiotic stress-induced plant senescence. Plant Molecul. Biol. 82, 623–633. doi: 10.1007/s11103-013-0038-z
Grassi, G., and Magnani, F. (2005). Stomatal, mesophyll conductance and biochemical limitations to photosynthesis as affected by drought and leaf ontogeny in ash and oak trees. Plant Cell Environ. 28, 834–849.
Gonzalez-Real, M. M., and Baille, A. (2000). Changes in leaf photosynthetic parameters with leaf position and nitrogen content within a rose plant canopy (Rosa hybrida). Plant Cell Environ. 23, 351–363.
Gupta, A. K., Kaur, K., and Kaur, N. (2011). Stem reserve mobilization and sink activity in wheat under drought conditions. Amer. J. Plant Sci. 2:70. doi: 10.4236/ajps.2011.21010
Inoue, T., Inanaga, S., Sugimoto, Y., and El Siddig, K. (2004). Contribution of pre-anthesis assimilates and current photosynthesis to grain yield, and their relationships to drought resistance in wheat cultivars grown under different soil moisture. Photosynthetica 42, 99–104.
Jabborova, D., Annapurna, K., Kakhramon, D., Abdujalil, N., Yuriy, E., Asad, S., et al. (2021). Co inoculation of rhizobacteria promotes growth, yield and nutrient contents in soybean and improves soil enzymes and nutrients under drought conditions. Sci. Rep. 11:22081. doi: 10.1038/s41598-021-01337-9
Khan, I., Samrah, A. A., Rizwana, I., Muhammad, R., Nosheen, A., Humaira, Y., et al. (2020). Effect of 24-Epibrassinolide on plant growth, antioxidants defense system and endogenous hormones in two wheat varieties under drought stress. Physiol. Planta 172, 696–706. doi: 10.1111/ppl.13237
Li, C., Jiang, D., Wollenweber, B., Li, Y., Dai, T., and Cao, W. (2011). Waterlogging pretreatment during vegetative growth improves tolerance to waterlogging after anthesis in wheat. Plant Sci. 180, 672–678. doi: 10.1016/j.plantsci.2011.01.009
Li, X., Pu, H., Liu, F., Zhou, Q., Cai, J., Dai, T., et al. (2015). Winter wheat photosynthesis and grain yield responses to spring freeze. Agronom. J. 107, 1002–1010. doi: 10.2134/agronj14.0460
Liu, H., Sultanm, M. A., Liu, X. L., Zhang, J., Yu, F., and Zhao, H. X. (2015). Physiological and comparative proteomic analysis reveals different drought responses in roots and leaves of drought-tolerant wild wheat ( Triticum boeoticum ). PLoS One 10:e0121852. doi: 10.1371/journal.pone.0121852
Liu, X., Fan, Y., Long, J., Wei, R., Kjelgren, R., Gong, C., et al. (2013). Effects of soil water and nitrogen availability on photosynthesis and water use efficiency of Robinia pseudoacacia seedlings. J. Environ. Sci. 25, 585–595. doi: 10.1016/S1001-0742(12)60081-3
Lv, X., Ding, Y., Long, M., Liang, W., Gu, X., Liu, Y., et al. (2021). Effect of foliar application of various nitrogen forms on starch accumulation and grain filling of wheat ( Triticum aestivum L.) under drought stress. Front. Plant Sci. 12:463. doi: 10.3389/fpls.2021.645379
Madani, A., Rad, A. S., Pazoki, A., Nourmohammadi, G., and Zarghami, R. (2010). Wheat ( Triticum aestivum L.) grain filling and dry matter partitioning responses to source: sink modifications under postanthesis water and nitrogen deficiency. Acta Sci. Agron. 32, 145–151.
Makino, A. (2011). Photosynthesis, grain yield, and nitrogen utilization in rice and wheat. Plant Physiol. 155, 125–129. doi: 10.1104/pp.110.165076
Maxwell, K., and Johnson, G. N. (2000). Chlorophyll fluorescence—a practical guide. J. Exp. Bot. 51, 659–668. doi: 10.1093/jexbot/51.345.659
Mobasser, H. R., Mohammadi, G. N., Abad, H. H., and Rigi, K. (2014). Effect of application elements, water stress and variety on nutrients of grain wheat in Zahak region. Iran. JBES. 5, 105–110.
Morgan, J. A. (1986). The effects of N nutrition on the water relations and gas exchange characteristics of wheat (Triticum aestivum L.). Plant Physiol. 80, 52–58. doi: 10.1104/pp.80.1.52
Moshelion, M., Halperin, O., Wallach, R., Oren, R. A., and Way, D. A. (2015). Role of aquaporins in determining transpiration and photosynthesis in water-stressed plants: crop water-use efficiency, growth and yield. Plant Cell Environ. 38, 1785–1793. doi: 10.1111/pce.12410
Murchie, E. H., Yang, J., Hubbart, S., Horton, P., and Peng, S. (2002). Are there associations between grain-filling rate and photosynthesis in the flag leaves of field-grown rice? Environ. Exp. Bot. 53, 2217–2224. doi: 10.1093/jxb/erf064
Najafi, S., Nazari Nasi, H., Tuncturk, R., Tuncturk, M., Sayyed, R. Z., and Amirnia, R. (2021). Bio fertilizer application enhances drought stress tolerance and alters the antioxidant Enzymes in medicinal pumpkin ( Cucurbita pepo convar. pepo var. Styriaca ). Horticulturae 7:588.
Nardino, M., Perin, E. C., Aranha, B. C., Carpes, S. T., Fontoura, B. H., de Sousa, D. J. P., et al. (2022). Understanding drought response mechanisms in wheat and multi-trait selection. PloS one 17:e0266368. doi: 10.1371/journal.pone.0266368
Niu, Y., Wang, Y., Li, P., Zhang, F., Liu, H., and Zheng, G. (2013). Drought stress induces oxidative stress and the antioxidant defense system in ascorbate-deficient vtc1 mutants of Arabidopsis thaliana . Acta Physiol. Planta. 35, 1189–1200. doi: 10.1007/s11738-012-1158-9
Ribaut, J. M., and Pilet, P. E. (1991). Effects of water stress on growth, osmotic potential and abscisic acid content of maize roots. Physiol. Planta. 81, 156–162.
Salam, A., Khan, A. R., Liu, L., Yang, S., Azhar, W., Ulhassan, Z., et al. (2022). Seed priming with zinc oxide nanoparticles downplayed ultrastructural damage and improved photosynthetic apparatus in maize under cobalt stress. J. Hazard. Mat. 423:127021. doi: 10.1016/j.jhazmat.2021.127021
Saneoka, H., Moghaieb, R. E., Premachandra, G. S., and Fujita, K. (2004). Nitrogen nutrition and water stress effects on cell membrane stability and leaf water relations in Agrostis palustris Huds. Environ. Exp. Bot. 52, 131–138. doi: 10.1016/j.envexpbot.2004.01.011
Shabbir, R. N., Waraich, E. A., Ali, H., Nawaz, F., Ashraf, M. Y., Ahmad, R., et al. (2016). Supplemental exogenous NPK application alters biochemical processes to improve yield and drought tolerance in wheat ( Triticum aestivum L.). Environ. Sci. Pollut. Res. 23, 2651–2662. doi: 10.1007/s11356-015-5452-0
Shi, J., Yasuor, H., Yermiyahu, U., Zuo, Q., and Ben-Gal, A. (2014). Dynamic responses of wheat to drought and nitrogen stresses during re-watering cycles. Agri. Water Manage. 146, 163–172. doi: 10.1016/j.agwat.2014.08.006
Siddiqui, M. H., Mutahhar, Y. Al-K, Al-Q Mohammed, A., Al-W Mohamed, H., Anil, G., Hayssam, M. A., et al. (2015). Response of different genotypes of faba bean plant to drought stress. Int. J. Mol. Sci. 16, 10214–10227. doi: 10.3390/ijms160510214
Siddiqui, M. H., Nasir, K. M., Soumya, M., Saud, A., Riyadh, A. B., Abdullah, A., et al. (2021). Hydrogen sulfide (H2S) and potassium (K+) synergistically induce drought stress tolerance through regulation of H+ ATPase activity, sugar metabolism, and antioxidative defense in tomato seedlings. Plant Cell Rep. 40, 1543–1564. doi: 10.1007/s00299-021-02731-3
Sui, N., Li, M., Liu, X. Y., Wang, N., Fang, W., and Meng, Q. W. (2007). Response of xanthophyll cycle and chloroplastic antioxidant enzymes to chilling stress in tomato over-expressing glycerol-3-phosphate acyltransferase gene. Photosynthetica 45, 447–454. doi: 10.1007/s11099-007-0074-5
Szalai, G., Kellõs, T., Galiba, G., and Kocsy, G. (2009). Glutathione as an antioxidant and regulatory molecule in plants under abiotic stress conditions. J. Plant Growth Regul. 28, 66–80.
Tan, W., Liu, J., Dai, T., Jing, Q., Cao, W., and Jiang, D. (2008). Alterations in photosynthesis and antioxidant enzyme activity in winter wheat subjected to post-anthesis water-logging. Photosynthetica 46, 21–27. doi: 10.1007/s11099-008-0005-0
Teixeira, E. I., George, M., Herreman, T., Brown, H., Fletcher, A., Chakwizira, E., et al. (2014). The impact of water and nitrogen limitation on maize biomass and resource-use efficiencies for radiation, water and nitrogen. Field Crops Res. 168:109. doi: 10.1016/j.fcr.2014.08.002
Thakur, P., Kumar, S., Malik, J. A., Berger, J. D., and Nayyar, H. (2010). Cold stress effects on reproductive development in grain crops: an overview. Environ. Exp. Bot. 67, 429–443. doi: 10.1016/j.envexpbot.2009.09.004
Wang, G. Y., Hu, Y. X., Liu, Y. X., Ahmad, S., and Zhou, X. B. (2021). Effects of supplement irrigation and nitrogen application levels on soil carbon–nitrogen content and yield of one-year double cropping maize in subtropical region. Water 13:1180. doi: 10.3390/w13091180
Wang, X., Vignjevic, M., Liu, F., Jacobsen, S., Jiang, D., and Wollenweber, B. (2014a). Drought priming at vegetative growth stages improves tolerance to drought and heat stresses occurring during grain filling in spring wheat. Plant Growth Regul. 75, 677–687. doi: 10.1007/s10725-014-9969-x
Wang, X., Vignjevic, M., Jiang, D., Jacobsen, S., and Wollenweber, B. (2014b). Improved tolerance to drought stress after anthesis due to priming before anthesis in wheat ( Triticum aestivum L.) var. Vinjett. J. Exp. Bot. 65, 6441–6456. doi: 10.1093/jxb/eru362
Wang, X., Zhang, X., Chen, J., Wang, X., Cai, J., Zhou, Q., et al. (2018). Parental drought-priming enhances tolerance to post-anthesis drought in offspring of wheat. Front. Plant Sci. 9:261. doi: 10.3389/fpls.2018.00261
Wu, Y., Liu, W., Li, X., Li, M., Zhang, D., Hao, Z., et al. (2011). Low-nitrogen stress tolerance and nitrogen agronomic efficiency among maize inbreds: comparison of multiple indices and evaluation of genetic variation. Euphytica 180, 281–290. doi: 10.1007/s10681-011-0409-y
Wu, Y., Wang, Y., Shi, H., Hu, H., Yi, L., and Hou, J. (2022). Time-course transcriptome and WGCNA analysis revealed the drought response mechanism of two sunflower inbred lines. PLoS One 17:e0265447. doi: 10.1371/journal.pone.0265447
Yang, S. H., Wang, L. J., and Li, S. H. (2007). Ultraviolet-B irradiation-induced freezing tolerance in relation to antioxidant system in winter wheat ( Triticum aestivum L.) leaves. Environ. Exp. Bot. 60, 300–307. doi: 10.1016/j.envexpbot.2006.12.003
Yang, Y., Guo, J., Wang, G., Yang, L., and Yang, Y. (2012). Effects of drought and nitrogen addition on photosynthetic characteristics and resource allocation of Abies fabri seedlings in eastern Tibetan Plateau. New For. 43, 505–518. doi: 10.1007/s11056-011-9295-3
Zeeshan, M., Hu, Y. X., Iqbal, A., Salam, A., Liu, Y. X., Muhammad, I., et al. (2021). Amelioration of AsV toxicity by concurrent application of ZnO-NPs and Se-NPs is associated with differential regulation of photosynthetic indexes, antioxidant pool and osmolytes content in soybean seedling. Ecotoxicol. Environ. Saf. 225:112738. doi: 10.1016/j.ecoenv.2021.112738
Zeeshan, M., Lu, M., Sehar, S., Holford, P., and Wu, F. (2020). Comparison of biochemical, anatomical, morphological, and physiological responses to salinity stress in wheat and barley genotypes deferring in salinity tolerance. Agronomy 10:127. doi: 10.3390/agronomy10010127
Zhang, L. X., Li, S. X., Zhang, H., and Liang, Z. S. (2007). Nitrogen rates and water stress effects on production, lipid peroxidation and antioxidative enzyme activities in two maize (Zea mays L.) genotypes. J. Agronom. Crop Sci 193, 387–397.
Zhang, X., Wang, X., Zhong, J., Zhou, Q., Wang, X., and Cai, J. (2016). Drought priming induces thermo-tolerance to post-anthesis high-temperature in offspring of winter wheat. Environ. Exp. Bot. 127, 26–36. doi: 10.3389/fpls.2016.00501
Zheng, C., Jiang, D., Liu, F., Dai, T., Jing, Q., and Cao, W. (2009). Effects of salt and waterlogging stresses and their combination on leaf photosynthesis, chloroplast ATP synthesis, and antioxidant capacity in wheat. Plant Sci. 176, 575–582. doi: 10.1016/j.plantsci.2009.01.015
Keywords : drought priming, antioxidant, abiotic stress, photosynthesis, wheat
Citation: Ullah A, Tian Z, Xu L, Abid M, Lei K, Khanzada A, Zeeshan M, Sun C, Yu J and Dai T (2022) Improving the effects of drought priming against post-anthesis drought stress in wheat ( Triticum aestivum L.) using nitrogen. Front. Plant Sci. 13:965996. doi: 10.3389/fpls.2022.965996
Received: 10 June 2022; Accepted: 05 July 2022; Published: 10 August 2022.
Reviewed by:
Copyright © 2022 Ullah, Tian, Xu, Abid, Lei, Khanzada, Zeeshan, Sun, Yu and Dai. This is an open-access article distributed under the terms of the Creative Commons Attribution License (CC BY) . The use, distribution or reproduction in other forums is permitted, provided the original author(s) and the copyright owner(s) are credited and that the original publication in this journal is cited, in accordance with accepted academic practice. No use, distribution or reproduction is permitted which does not comply with these terms.
*Correspondence: Tingbo Dai, [email protected]
Disclaimer: All claims expressed in this article are solely those of the authors and do not necessarily represent those of their affiliated organizations, or those of the publisher, the editors and the reviewers. Any product that may be evaluated in this article or claim that may be made by its manufacturer is not guaranteed or endorsed by the publisher.
Thank you for visiting nature.com. You are using a browser version with limited support for CSS. To obtain the best experience, we recommend you use a more up to date browser (or turn off compatibility mode in Internet Explorer). In the meantime, to ensure continued support, we are displaying the site without styles and JavaScript.
- View all journals
- My Account Login
- Explore content
- About the journal
- Publish with us
- Sign up for alerts
- Open access
- Published: 07 March 2019
Developmental and physiological responses of Brachypodium distachyon to fluctuating nitrogen availability
- L. C. David 1 na1 ,
- T. Girin ORCID: orcid.org/0000-0002-5738-7731 1 na1 ,
- E. Fleurisson 1 ,
- E. Phommabouth 1 ,
- A. Mahfoudhi 1 ,
- S. Citerne 1 ,
- P. Berquin 1 ,
- F. Daniel-Vedele 1 ,
- A. Krapp 1 &
- S. Ferrario-Méry 1
Scientific Reports volume 9 , Article number: 3824 ( 2019 ) Cite this article
2757 Accesses
9 Citations
4 Altmetric
Metrics details
- Plant molecular biology
- Plant physiology
The Nitrogen Use Efficiency (NUE) of grain cereals depends on nitrate (NO 3 − ) uptake from the soil, translocation to the aerial parts, nitrogen (N) assimilation and remobilization to the grains. Brachypodium distachyon has been proposed as a model species to identify the molecular players and mechanisms that affects these processes, for the improvement of temperate C3 cereals. We report on the developmental, physiological and grain-characteristic responses of the Bd21-3 accession of Brachypodium to variations in NO 3 − availability. As previously described in wheat and barley, we show that vegetative growth, shoot/root ratio, tiller formation, spike development, tissue NO 3 − and N contents, grain number per plant, grain yield and grain N content are sensitive to pre- and/or post-anthesis NO 3 − supply. We subsequently described constitutive and NO 3 − -inducible components of both High and Low Affinity Transport Systems (HATS and LATS) for root NO 3 − uptake, and BdNRT2/3 candidate genes potentially involved in the HATS. Taken together, our data validate Brachypodium Bd21-3 as a model to decipher cereal N nutrition. Apparent specificities such as high grain N content, strong post-anthesis NO 3 − uptake and efficient constitutive HATS, further identify Brachypodium as a direct source of knowledge for crop improvement.
Similar content being viewed by others
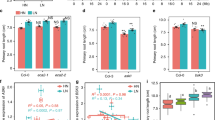
Natural variation of BSK3 tunes brassinosteroid signaling to regulate root foraging under low nitrogen
Zhongtao Jia, Ricardo F. H. Giehl, … Nicolaus von Wirén
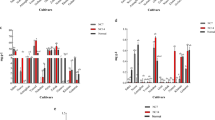
Investigation of morpho-physiolgical traits and gene expression in barley under nitrogen deficiency
Zohreh Hajibarat, Abbas Saidi, … Zahra Hajibarat
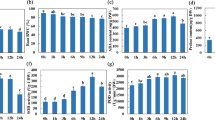
Root Physiological Traits and Transcriptome Analyses Reveal that Root Zone Water Retention Confers Drought Tolerance to Opisthopappus taihangensis
Yongjuan Yang, Yanhong Guo, … Ming Sun
Introduction
The Poaceae family includes cereal crops such as wheat, rice, maize and barley, which provides a major source of food for humans and cattle. It is predicted that by 2050, the human population will increase by 20–50% in parallel to an increase of the living standard and thus, a doubling of grain demand is expected 1 , 2 , 3 . As arable land becomes limited, crop grain yields need to be improved. Nitrogen (N) is a major limiting macronutrient in the fields for crop production and as a consequence, N fertilizers are largely applied in modern agriculture to enhance plant vegetative growth and grain production. From an ecological point of view, the use of large amounts of N fertilizer on fields results in nitrate (NO 3 − ) leaching, which causes eutrophication and biodiversity depletion. Thus, increasing crop yields while diminishing environmental impacts of agriculture is a major goal of today’s agriculture. However, high-yielding modern cultivars are not suitable, as they have high nutrient requirements, due to their breeding under high nutrient availability 4 , 5 . Fundamental knowledge on molecular mechanisms of plant nutrition must be increased to engineer new cultivars giving high yields under low nutrient availability.
Mechanisms directly affecting the plant Nitrogen Use Efficiency (NUE) have been largely described at the physiological level in crop species (including wheat, barley, rice and corn) and in the model species, Arabidopsis thaliana (Arabidopsis) 6 , 7 , 8 , 9 . Most characterizations at the molecular level have however been restricted to the dicot Arabidopsis 10 , 11 , 12 . Brachypodium distachyon (Brachypodium) has been proposed as a good model to enhance this knowledge in C3 temperate cereals 13 . This non-domesticated monocot species offers convenient characteristics for academic research (simple diploid sequenced genome, short life cycle, simple growth requirement, large mutant collections, efficient genetic transformation), and is phylogenetically closely related to wheat and barley, enabling an efficient translational approach.
For grain crops, NUE is defined as the ratio between grain yield and available N in the soil per unit of field surface, and is often approximated as the ratio between yield and added N 14 . It is dependent on processes such as N uptake, translocation, assimilation and remobilization 11 . N is mainly taken up from the soil as nitrate (NO 3 − ) and ammonium (NH 4 + ) ions, thanks to specialized root transporters. NO 3 − being the most abundant form in aerobic fields, it constitutes the main N source for most temperate crops 11 , 15 , 16 , 17 . NO 3 − uptake relies on the activity of root High and Low Affinity Transport Systems (HATS and LATS, respectively), involving NO 3 − transporters of the NRT2 and NPF families 18 , 19 , 20 , 21 , 22 , 23 . HATS is active in low nitrate conditions (<1 mM) while LATS is predominant in higher nitrate concentrations. In many species, it has been shown that HATS is partly inducible by nitrate supply subsequently to nitrate deprivation (iHATS). In addition, a constitutive HATS is active under all environmental conditions (cHATS). The induction of HATS by NO 3 − is transient, and long exposure to NO 3 − and/or to other sources of N triggers a repression of NO 3 − influx 24 , 25 , 26 , 27 . Furthermore, HATS is a multi-component system since the interaction between NRT2 and its partner protein NRT3 is required for a functional NO 3 − transport activity 28 . In Brachypodium, 5 BdNRT2 genes have initially been identified by phylogenetic analysis and 2 more have been found in a subsequent analysis 13 , 29 .
After being taken up from the soil, NH 4 + is mainly assimilated in roots, while NO 3 − is mostly translocated to aerial organs, where it is successively assimilated into nitrite, NH 4 + , glutamine and glutamate by the consecutive actions of Nitrate Reductase (NR), Nitrite reductase (NiR) and the GS/GOGAT (Glutamine Synthetase/Glutamate Synthase) cycle 11 , 30 . Glutamate constitute the precursor of other amino acids and N-containing organic molecules of the plant 11 , 30 . During plant aging, remobilization processes take place, reallocating N from the source to sink organs 12 . In annual plants, the ultimate remobilization process is the monocarpic senescence (senescence of all vegetative organs), leading to plant death, while grains are filled with reserves 12 . The final NUE can potentially be improved by adjusting these physiological processes.
The potential of enhancing NO 3 − uptake/assimilation or N remobilization for increased grain yield is currently a matter of debate. Studies performed in rice, wheat and maize showed that more than half of the Grain Nitrogen Content (GNC) originate from leaf remobilization, and that this proportion depends on genotypes and soil N availability 31 , 32 , 33 . The importance of remobilization is also highlighted by an increased productivity of stay-green lines, having a delayed senescence 34 . Usually, this yield increase is however associated with a decrease in Grain Protein Content (GPC, directly related to grain N content), which is detrimental to the nutritional and food-processing qualities of the grain. Functional studies of wheat and barley NAM-B1 transcription factors suggest that, in some conditions, a tight control of senescence processes can lead to a higher GPC without decrease in grain yield 35 , 36 . On the other hand, it has been suggested that enhancing the post-anthesis N uptake is the main way to increase both yield and GPC in wheat 37 , 38 .
To our knowledge, three studies have been published on N-related mechanisms in Brachypodium. Ingram and colleagues 39 analyzed the effects of N availability on root architecture and highlighted the variability of the response between accessions. Poiré and colleagues 40 revealed the stimulation of Brachypodium growth, leaf N content and photosynthetic capacity by high N availability during the vegetative stages. Hong and colleagues 41 showed that the interaction with symbiotic arbuscular mycorrhiza stimulates the expression of putative NH 4 + transporters, but no effect was seen on N leaf content. As far as we know, no reported study in Brachypodium investigated the dependency of grain yield or GPC on physiological mechanisms such as N uptake, assimilation or remobilization.
Here, we report on a general characterization of Brachypodium physiology and responses to N availability, with a specific focus on grain production. We focussed on the Bd21-3 accession, as it is now the reference accession around the world due to its high transformation efficiency and to the availability of mutants in this background, allowing reverse-genetic approaches. We characterized the effects of NO 3 − availability at both vegetative and reproductive stages on growth, architecture, tissue composition and grain characteristics. We subsequently highlighted the unusual importance of post-anthesis NO 3 − uptake for grain N loading. Finally, NO 3 − transport systems were described at the physiological level, and candidate genes for root high affinity NO 3 − uptake were identified. This data provides a basis for molecular deciphering of NUE-related mechanisms in Brachypodium, as a model for temperate C3 cereals.
Results and Discussion
No 3 − availability at vegetative stage impacts plant growth, development and tissue composition.
The effect of external NO 3 − availability on vegetative growth and development was investigated in 35-days-old plants, grown on sand and watered with a nutritive solution containing 0.1, 2 or 10 mM NO 3 − as sole source of N. The total fresh weight of plants grown on 0.1 mM NO 3 − was reduced when compared to the other conditions (64% reduction as compared to 10 mM NO 3 − ). No significant difference was observed between plants grown on 2 and 10 mM NO 3 − (Fig. 1a ; Supplementary Information Fig. S1 ). A similar effect was observed on the root fresh weight. In contrast, a reduction of shoot fresh weight (Fig. 1a ) was already observed on 2 mM NO 3 − as compared to 10 mM NO 3 − , and more severe on 0.1 mM NO 3 − . Brachypodium Bd21-3 shoot biomass was thus more sensitive to NO 3 − limitation than root biomass, corresponding to the well documented decrease of shoot/root ratio under N limiting conditions (Supplementary Information Fig. S1b ) in a variety of plants 42 , 43 , and to a previous study on Brachypodium Bd21-3 40 .
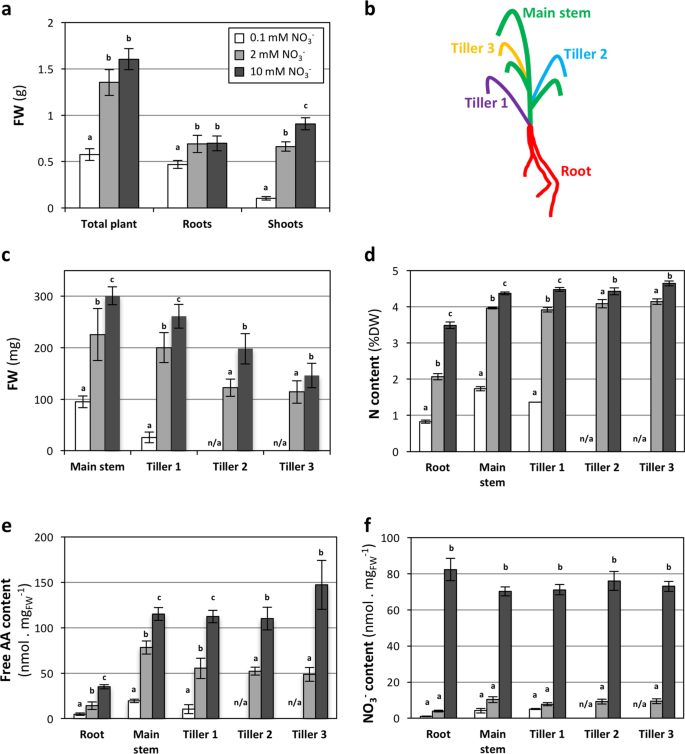
Effects of NO 3 − availability at vegetative stage. Plants were grown on sand for 35 days, watered with nutritive solution containing 0.1, 2 or 10 mM NO 3 − . (a) Fresh weight of total plant, roots and shoots. (b) Schematics of tiller development. Tiller were numbered according to their sequential apparition. (c) Fresh weight, (d) N content, (e) Free AA content and (f) NO 3 − content of main stem and tillers. Values correspond to the mean of 4 or 5 biological replicates (2 plants each) +/−SD. Letters indicate statistical groups for each plant part (Non-parametric ANOVA, p < 0.05). N/a: no data available, due to the lack of development of tillers 2 and 3 in the 0.1 mM NO 3 − condition.
Tillering is a primordial characteristic of cereals, as it influences the number of spikes and thus the grain yield. To further characterize the effect of N availability, the main stem and the different tillers (Fig. 1b ) were separated. At 0.1 mM NO 3 − , only 40% of the plants developed a first tiller (data not shown) and none developed tillers of higher ranks. In the other conditions, all plants produced 3 tillers, in addition to the main stem. This effect of N availability on tiller number is consistent with observations made in wheat and barley 44 , 45 , 46 . As for total shoot, the fresh weight of the main stem and of tillers of different ranks were progressively reduced with the reduction of NO 3 − availability (Fig. 1c ). Thus, both shoot growth and development were limited by low N availability on 0.1 mM NO 3 − , whereas only shoot growth was affected on 2 mM NO 3 − , with a similar effect on the main stem and tillers.
To characterize the effect of NO 3 − availability on the plant, Nitrogen (N), Carbon (C), free amino acids (AA) and NO 3 − contents of the tissues were measured. Nitrogen content was reduced in all parts of the plant (roots, main stem and tillers) on both 0.1 and 2 mM conditions as compared to 10 mM NO 3 − , with a stronger effect on 0.1 mM (Fig. 1d ). The nitrate availability had a minor effect on C content (Supplementary Information Fig. S1c ). Consequently, C/N ratio was reduced by increasing NO 3 − availability, with similar patterns for the different plant parts (Supplementary Information Fig. S1d ). The effect on AA content was qualitatively similar to that observed on N content, with significant differences between the three conditions, and comparable effects on all parts of the plant (Fig. 1e ). Free AA content was quantitatively more affected than N content at the whole plant level, with 91% and 55% global decrease on 0.1 mM and 2 mM, respectively, when compared to 10 mM (whole plant N content was reduced in parallel by 76% and 25%) (Supplementary Information Fig. S1e,f ). Nitrate content was also strongly affected in all plant parts by low external NO 3 − availability (Fig. 1f ), being globally reduced by 98% and 91% on 0.1 and 2 mM, respectively (Supplementary Information Fig. S1g ).
Thus, external NO 3 − limitation led to a strong decrease of internal NO 3 − pools and a strong to moderate decrease of both free AA and N contents, leading to a moderate effect on plant growth and development. Interestingly, the main stem and initiated tillers responded similarly to reduced NO 3 − availability, suggesting there is no prioritization for N allocation between main stem and tillers.
Both pre- and post-anthesis NO 3 − availability affect vegetative growth and grain yield
Based on the effects seen at the vegetative stage, we further investigated the effects of pre- and post-anthesis N availability on end of cycle plant and grain characteristics. Plants were grown hydroponically on a medium containing NO 3 − as a sole source of N. Control plants (Ctrl) were maintained on media containing 1 mM NO 3 − . This condition was chosen based on the previous experiment. This nitrate concentration should lead to intermediate growth and N physiological state of the plants, enabling to test both positive and negative effects of changes in external NO 3 − availability. Four treatment conditions were used: plants grown on media containing either low N (LN; 0.1 mM NO 3 − ) or high N (HN; 10 mM NO 3 − ) until anthesis of the first spikes, then transferred to 1 mM NO 3 − ; and conversely, plants grown on 1 mM NO 3 − until anthesis, then transferred to LN or HN. Plants grown in the three different conditions at vegetative stage sequentially reached the anthesis stage (Supplementary Information Fig. S2a ). Plants grown at vegetative stages on 0.1 and 1 mM NO 3 − exhibited similar fresh weights at anthesis, despite a shift to reach this stage, whereas plants grown on 10 mM NO 3 − were more developed (Supplementary Information Fig. S2b ).
At the end of the cycle, grain yield per plant was increased by pre-anthesis HN treatment (+68%), reduced by post-anthesis LN treatment (−42%), and not affected in other conditions (Fig. 2a ). The shoot dry weight followed a similar pattern (+56%, −26% and no effect, respectively; Fig. 2b ). Accordingly, the harvest index (grain/total above-ground dry weights) was not affected by the treatments as compared to the control condition (Supplementary Information Fig. S2c ), suggesting that the variations in grain yield are a consequence of variations in vegetative growth. In barley and wheat, grain yield is similarly dependent on the vegetative biomass, which related to the nitrogen fertilization 44 , 47 . In contrast, the effect of post-anthesis N availability (LN treatment) on grain yield and shoot biomass was surprising, as it is usually not observed in cereals 37 , 48 , 49 . This was linked to the continuation of shoot growth after anthesis, whereas it is generally admitted that vegetative growth stops around anthesis stage in cereals 37 . The maintenance of vegetative growth after anthesis in Brachypodium Bd21-3 might be linked to the undomesticated nature of the species. In addition, it could have been enhanced by the growing conditions used in our study, as it has been reported that tillering hydroponic-grown wheat can continue for an indefinite time, under non-limiting conditions 44 .
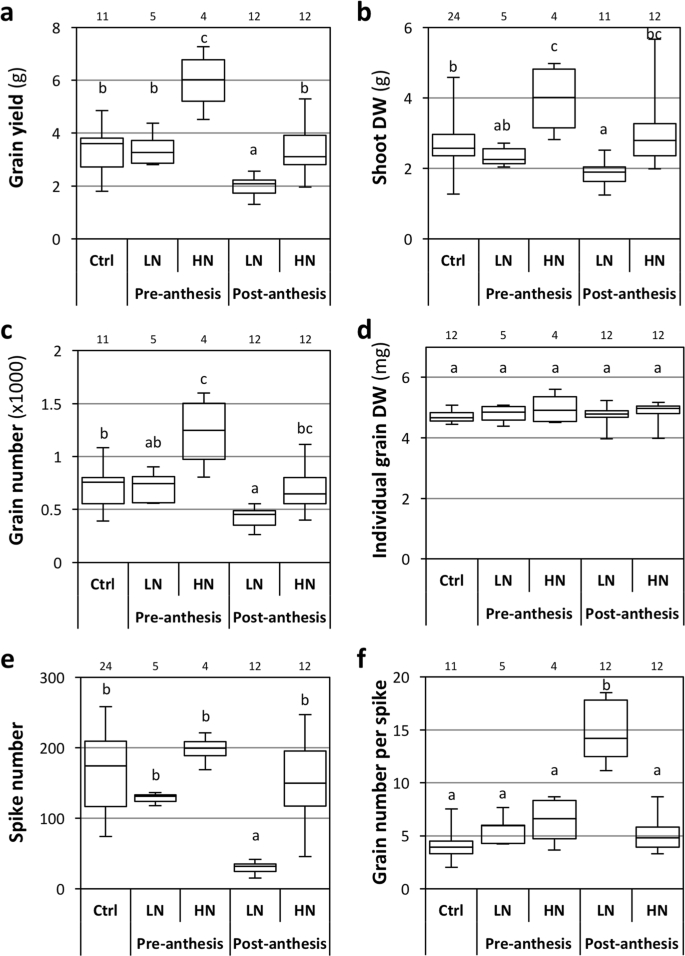
Effects of pre- and post-anthesis NO 3 − availability on end of cycle characteristics. Plants were grown in hydroponics. Ctrl: control plants grown on 1 mM NO 3 − during the entire life cycle. Pre-anthesis: plant grown on 0.1 or 10 mM NO 3 − (LN and HN, respectively) until anthesis, and subsequently on 1 mM NO 3 − . Post-anthesis: plant grown on 1 mM NO 3 − until anthesis, and subsequently on LN or HN. (a) Grain yield per plant. (b) Shoot Dry Weight (DW). (c) Grain number per plant. (d) DW of individual grain. (e) Spike number per plant. (f) Grain number per spike. The boxplots represent minimum, 1 st quartile, median, 3 rd quartile and maximum values. Letters indicate statistical groups (Non-parametric ANOVA, p < 0.05). Number of biological replicates for each condition are indicated above the charts.
Plants grown on 10 mM NO 3 − up to anthesis and subsequently on 1 mM NO 3 − presented higher end-of-cycle shoot biomass and grain yield than the control plants. In contrast, shoot biomass and grain yield were not stimulated by the 10 mM post-anthesis treatment when compared to the control, and were reduced by the 0.1 mM post-anthesis treatment. Thus, in our conditions, 1 mM NO 3 − was sufficient for maximal growth in late, but not in early development. This is consistent with the observation made by Gastal and Lemaire 50 , that the N uptake demand from the plant to achieve maximal growth decreases as the plant gets bigger. Our observation might thus reflect the negative relationship between plant biomass and N supply necessary for maximum growth, known as the critical N curve and observed for many crops 50 , 51 .
Grain yield depends on grain number and grain abortion rate
Grain yield in C3 temperate cereals is known to be affected by environmental conditions through both grain number and grain size 52 , 53 , 54 , 55 . Thus, we explored the effects of N availability on these traits. The grain number per plant was increased by pre-anthesis HN treatment and reduced by post-anthesis LN treatment (+65% and −40%, respectively; Fig. 2c ), whereas the individual grain weight was not affected (Fig. 2d ). This established a direct link between grain yield and grain number. Such an absence of an effect of N nutrition on grain weight has previously been described for wheat grown in pots or in hydroponics 44 , 56 . It is thus possible that grain weight is more sensitive to N availability in the field than in controlled conditions.
We subsequently analyzed the basis of the variation of grain number. The pre-anthesis HN treatment did not statistically increase the number of spikes (equal to the number of tillers, as all tillers developed spikes in the 5 conditions), nor the grain number per spike (Fig. 2e,f ). This suggests that the increased grain yield in this condition is due to slight multiplicative increases in both the number of tillers and the grain number per spike. On the other hand, the yield decrease in the post-anthesis LN treatment correlated with a strong reduction of spike number (−82%; Fig. 2e ) and a high increase in average grain number per spike (+261%; Fig. 2f ). This increase in grain number per spike was due to a reduction of grain abortion to 25% of grain initiation, as compared to 53% in the control condition (Supplementary Information Fig. S2d ). Thus, the post-anthesis initiation of tillers was restricted by low N availability, but grain production was partially compensated by a greater development of the spikes. Interestingly, the general high proportion of grain abortion confirms the observation by Oscarson 44 , stating that in most N conditions, wheat plants produce slightly more tillers than can be supported for grain filling. The author hypothesized that producing extra tillers enables the plant to produce more grains in case of a late increase in nutrient availability.
Post-anthesis N availability affects N and protein contents and free AA composition of the grains
We investigated the effect of the pre- and post-anthesis N treatments on grain composition, including N and protein contents. Grain protein content (GPC), directly related to grain N content (GNC), is a main criterion for cereal grain quality, in relation to the end-use. For malting barley, the GPC should be in the range of 8.5–12.5% DW (corresponding to GNC of 1.4–2% DW), and can be higher for feed barley 57 , 58 . A high protein content is favorable for the baking quality of wheat flour 59 , and GPC between 10–15% are thus usually reported for bread wheat grains (corresponding to GNC of 1.75–2.6%) 8 , 37 , 60 , 61 . Recently, high GNC (ranging 2.3–3.0%) has been reported in bread wheat grown in a semi-hydroponic system 49 . In our experiment, GNC was around 3% DW (Fig. 3a ), corresponding to an estimated GPC of 17% DW when using the accepted conversion factor of 5.7 62 . Our results are in agreement with the high GNC (2.9–3.7%) reported for Brachypodium Bd21-3 in different growth conditions 63 , 64 . These results underline the generally high grain N content of Brachypodium Bd21-3, and identify this species (or at least this accession) as a source of knowledge for the improvement of bread wheat GPC.
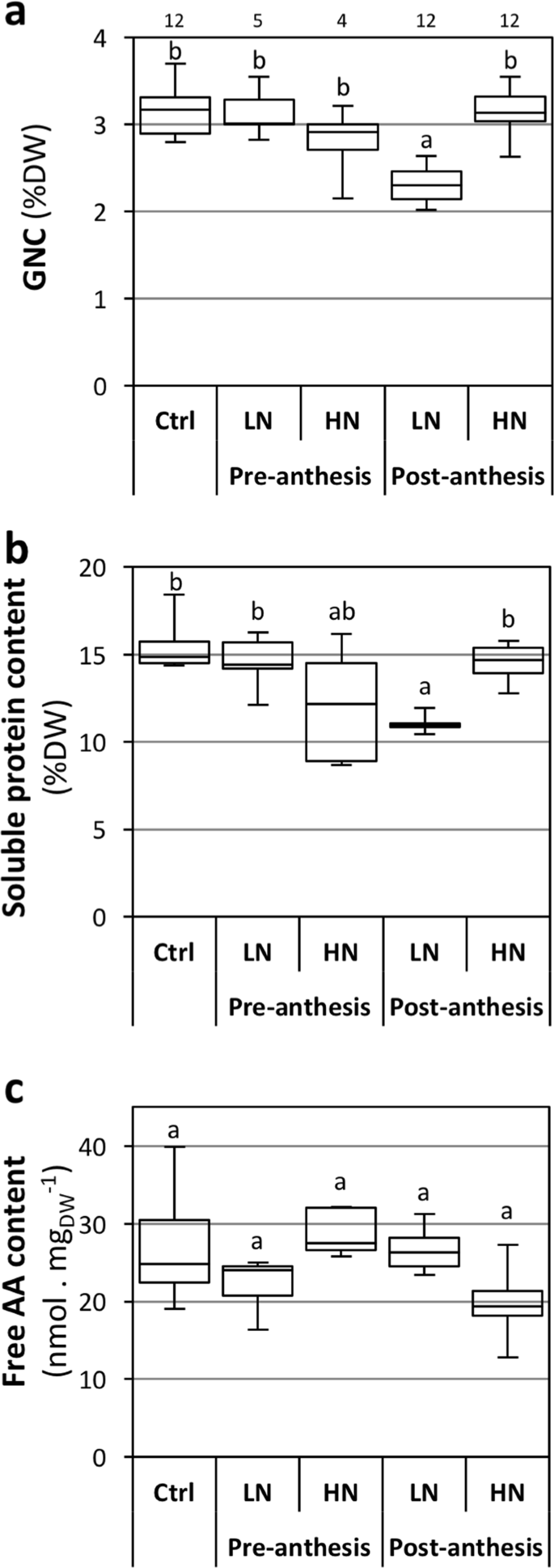
Effects of pre- and post-anthesis NO 3 − availability on grain composition. Growing conditions and treatments were as described in Fig. 2 . LN, Ctrl and HN: 0.1, 1 and 10 mM NO 3 − , respectively. Grain contents in (a) total N (GNC), (b) extractable proteins and (c) free AA. The boxplots represent minimum, 1 st quartile, median, 3 rd quartile and maximum values. Letters indicate statistical groups (Non-parametric ANOVA, p < 0.05). Number of biological replicates for N content are indicated above the chart; other charts are based on 5 biological replicates per condition.
The GNC was quite unaltered by pre- and post-anthesis N treatments (Fig. 3a ), as it was statistically affected only by the post-anthesis LN treatment (−27%). In combination with the reduction of grain yield in this condition, this highlights the sensitivity of Bd21-3 to low N availability for grain quality and quantity during the reproductive phase. Interestingly, GNC was unaffected by the pre-anthesis HN treatment that triggered an enhanced grain yield, underlining independency between the traits. Cereal GNC is known to be highly dependent on post-anthesis N uptake and on remobilization from vegetative organs of N assimilated before anthesis 37 , 48 , 65 . Accordingly, a late field application of fertilizer is usually recommended to improve grain N uptake during grain filling, and thus grain protein content 66 , as observed in our experiment. The source limitation of GNC was apparent for low N treatment, showing that N remobilization could not compensate the reduction of external N availability. This is in agreement with the dominance of post-anthesis uptake over remobilization for grain N filling in our conditions (see thereafter).
Compared to the control condition, the grain C content was marginally reduced under all treatments, except by the pre-anthesis LN treatment (Supplementary Information Fig. S2e ). The C/N ratio was only significantly modified in the post-anthesis LN treatment (Supplementary Information Fig. S2f ). The grain content in soluble proteins followed the same pattern as the GNC, with only the post-anthesis LN condition presenting a significant difference with the control (−26%; Fig. 3b ). The grain soluble protein content ranged between 10–15%, which is consistent with published studies in Brachypodium Bd21-3 63 , 64 . The grain composition in soluble protein was visualized by SDS PAGE (Supplementary information Fig. S3 ). The protein profile was consistent with a previous report on Brachypodium grain protein composition 63 , and no major differences in the protein profiles was found between the treatments.
Although grain free amino acid (AA) content represents a small proportion of total grain N content (less than 1%) 67 when compared to seed protein (around 60%), it could indicate an effect of N availability on grain N storage capacity 68 . The free AA pool results from the balance between neo-synthesis in grain and AA remobilization from leaves on the one hand, and seed protein synthesis during grain development on the other. In our experiment, no significant change in total free AA content was observed (Fig. 3c ), suggesting an absence of global nitrogen imbalance between grain AA supply and seed protein synthesis. In contrast, the analysis of the free AA composition revealed some specific changes. For instance, Asn (27–44% of free AA), Pro (4–17%), Gln (5–10%), Ala (5–10%), Asp (5–7%), Ser (3–6%), Glu (3–6%) and Arg (3–6%) represented the main free AA’s in the grain (Supplementary Information Table S1 ). Post-anthesis treatments induced significant changes in the content of some of the free AA’s (Fig. 4 ). Post-anthesis LN treatment triggered a large increase in Trp (x45), moderate increases in Thr, Ser, and Ala, and a decrease in Pro (x1/3) (Fig. 4 ). Post-anthesis HN treatment triggered moderate decreases (x1/2) in Thr, Ser and Val. The effects of post-anthesis treatments on the free AA pool may result from specific variations in N remobilization or protein synthesis.
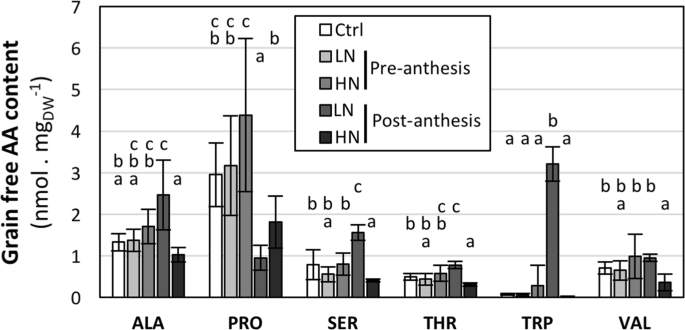
Effects of pre- and post-anthesis NO 3 − availability on grain free AA composition. Growing conditions and treatments were as described in Fig. 2 . LN, Ctrl and HN: 0.1, 1 and 10 mM NO 3 − , respectively. Only free AA levels affected by the treatments are presented; see Table S1 (Supplementary Information) for full set of data. Values are means of 5 biological replicates +/−SD. Letters indicate statistical groups for each AA (Non-parametric ANOVA, p < 0.05).
The storage proteins in Brachypodium Bd21-3 grains are composed mainly of globulins (50–60%) and prolamins (12%), similar to that in rice and oat 64 . The major AA’s of grain proteins are Glx, Asx, Pro and Gly (20%, 10%, 8.6% and 8.5% respectively) 64 . In wheat, accumulation of prolamins correlates with the post-anthesis N supply 65 , 69 . Interestingly, Brachypodium prolamins are homologous to wheat avenin-like and γ-gliadins, the latter being characterized by a high content in Pro (17.4%) 64 . Thus, the decrease of grain free Pro content in our post-anthesis LN treatment could correlate with a limitation in prolamin synthesis. Additionally, the lower protein level observed in post-anthesis LN suggests that N fertilization has the same effect on prolamin as it does in wheat. The other major free AAs (Glx, Asx, and Gly) found in grain proteins of Brachypodium Bd21-3 did not show significant changes in the free AA pool. Some less abundant amino acids (Thr, Ser, Ala, and Val) were increased by post-anthesis N limitation and/or decreased by post-anthesis N abundance, thus were likely not limiting for globulin synthesis. Trp is an essential amino acid for human and animal nutrition and is usually in limiting concentrations in wheat grain. We observed a spectacular increase of Trp in the grains after post-anthesis LN treatment. Trp was below the detection threshold in a previous study 64 , due to the methodology (acidic hydrolysis of protein) that degrades this AA. Trp content in prolamin varies between grain cereals, being lower in wheat prolamin than in the globulin fraction 67 , 70 . Thus, our results highlight a limitation in seed protein synthesis, probably in prolamin but also in globulin after post-anthesis LN treatment.
Grain N loading depends primarily on post-anthesis N uptake
Our data highlighted an important correlation of both grain quality and quantity with post-anthesis NO 3 − availability (Figs 2 and 3 ), thus we further characterized N fluxes in the plant at this stage. N grain loading in cereals is dependent on both N remobilization from vegetative organs (taken up before anthesis) and post-anthesis N uptake 37 , 48 , 65 . We thus quantified both processes and investigated their coordinated responses to variations in NO 3 − availability. Plants were grown hydroponically on media containing 0.2 mM NO 3 − until anthesis of the first spikes, then transferred to conditions of either N deprivation, limitation or abundance (0.01 (A), 0.2 (B) and 10 (C) mM NO 3 − , respectively). These contrasting conditions were chosen to maximize the effect of the treatments on both grain yield per plant and GNC (Supplementary Information Fig. S4 ). A pulse-chase strategy was used at the vegetative phase (replacement of the NO 3 − by 15 NO 3 − for 5 days) to enrich the vegetative tissues with 15 N stable isotope. Plants were harvested either at anthesis (to map the 15 N distribution at the end of vegetative phase) or after full senescence of the plant. Roots, shoots and spikes were collected separately, and dry weight, N content and 15 N enrichment were measured (Supplementary Information Table S2 ). Nitrogen fluxes between plant parts after anthesis were then calculated as previously described 71 .
The post-anthesis NO 3 − uptake was stronger than remobilization fluxes from vegetative shoots and roots in all conditions, and was highly stimulated by NO 3 − availability (Fig. 5 ; see Supplementary Information Table S3 for statistical tests). Hence, end of cycle grain N originated mainly from NO 3 − taken up at the post-anthesis stage (59% to 91%, in deprivation and abundance conditions, respectively). Grain N originating from remobilization was significantly reduced under high N availability, both on relative values (9% and 41% of grain N under abundance and deprivation, respectively) and on absolute quantities (4.8 and 6.5 mg N ), reflecting a negative effect of N availability on N remobilization. The proportion of total plant N localized in the grain at the end of the cycle was relatively high in all conditions, ranging from 65% under abundance to 73% under deprivation (Fig. 5 ). This was consistent with the strong allocation of post-anthesis N uptake to the grain (65% to 70%).
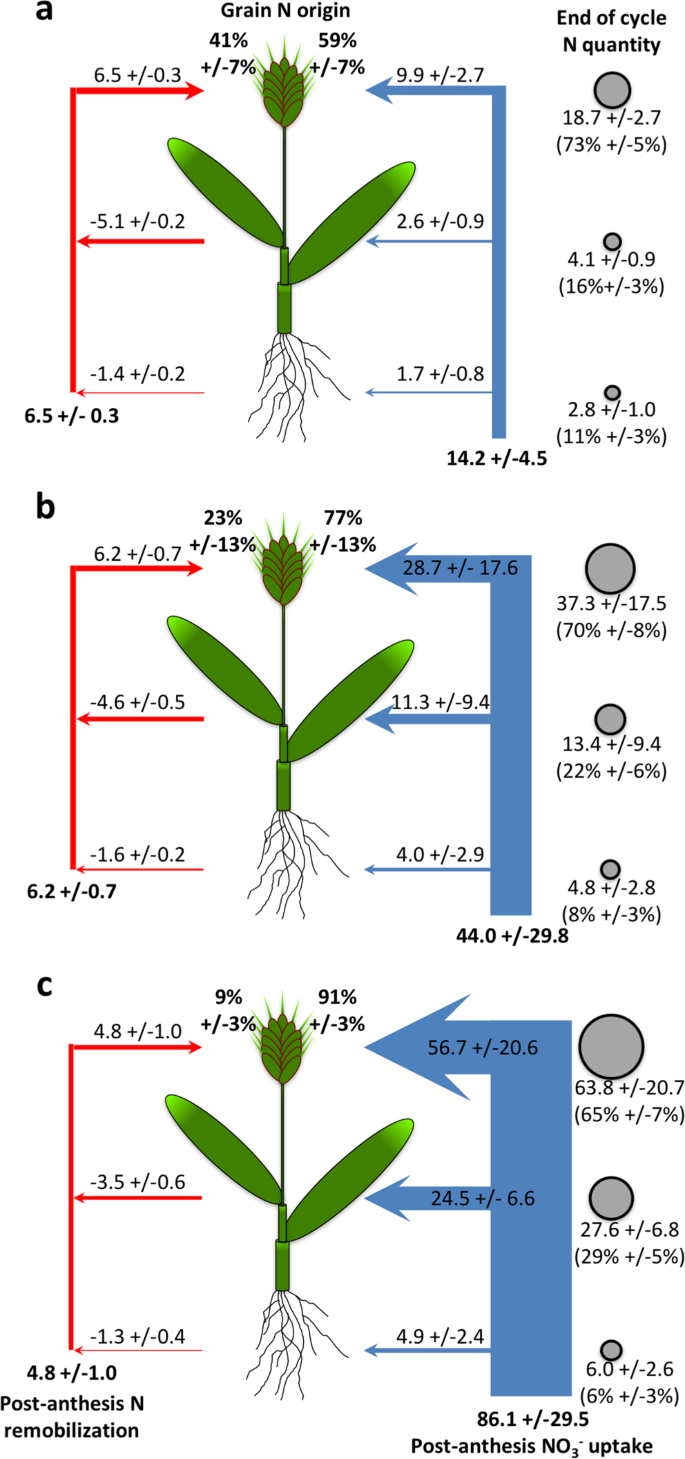
Effect of post-anthesis NO 3 − availability on N fluxes. Plants were grown hydroponically on 0.2 mM NO 3 − and transferred at anthesis to (a) 0.01, (b) 0.2 or (c) 10 mM NO 3 − . Left arrows (red): post-anthesis remobilization of pre-anthesis N pools from vegetative plant parts. Right arrows (blue): post-anthesis NO 3 − uptake. Arrows indicate the N fluxes from/to spikes, shoots or roots; values of the fluxes (mg N ) are specified above the arrows. Bold values at the bottom of the panels correspond to total N remobilization (left) and total NO 3 − uptake (right) at the plant level (mg N ). Percentages above the spikes indicate the proportion of grain N originating from remobilization (left) and from post-anthesis uptake (right). Total N quantities in spikes, shoots and roots after full senescence of the plants are indicated by circles on the right of each panel; absolute values (mg N ) are indicated; distribution of N quantity between spikes, shoots and roots are specified in brackets (% of the plant total N). Values correspond to the mean of 5 (panel a) or 7 (panels b and c) biological replicates +/−SD. Raw data (DW, N content and 15 N enrichment of the different plant parts) and results of statistical tests are presented in Supplementary tables ST2 and ST3, respectively.
In cereals such as wheat, barley, rice and maize, remobilization is regarded as the main N source for grain loading, providing 60–90% of the final grain N, depending on genotypes and environmental conditions 32 , 33 , 48 , 56 , 72 . In our experiment, remobilization had a minor role, furnishing 9–41% of grain N depending on post-anthesis NO 3 − availability. A low importance of remobilization has recently been described in wheat: out of 8 measurements (2 genotypes grown in 4 conditions, including low and high N availability), 7 ranged from 0% to 37%, and only one had a value above 50% 49 . This study, based on plants grown in a semi-hydroponic system in a growth chamber, could suggest that our observed low values are linked to our experimental setup. Alternatively, it has been reported that a low pre-anthesis and/or a high post-anthesis N availability reduce the contribution of remobilization for grain loading 49 , 73 , 74 , which may be the case in our study. Finally, it is also possible that grain N loading in Brachypodium Bd21-3 is mainly based on post-anthesis N uptake, rather than on N remobilization. A similar case has been reported for instance in sorghum 75 , 76 and in stay-green maize genotypes 77 . In the case of Brachypodium, the relative importance of post-anthesis NO 3 − uptake could be related to the non-domesticated nature of the species. Investigating several Brachypodium accessions, each grown in various conditions, would be necessary to confirm whether a genetic and/or environmental effect is the reason for this high contribution of post-anthesis NO 3 − uptake for grain N loading.
High external NO 3 − availability had a strong positive effect on NO 3 − uptake and a weak, but significant, repressive effect on N remobilization from shoots to grains (6.2 mg N on 0.2 mM NO 3 − versus 4.8 mg N on 10 mM NO 3 − ). This reduced remobilization in situations of high post-anthesis N uptake have frequently been observed in wheat 37 , 49 , 78 , 79 , 80 . As highlighted by Bancal 81 , the difference in remobilization has a limited impact on the N distribution to the grain (1.4 mg N difference in remobilization between 0.2 and 10 mM conditions, to be compared to a total grain N content of 37.3 and 63.8 mg N ; Fig. 5 ). Accordingly, the NO 3 − availability had no statistically significant effect on N partitioning (73% of the total plant N was located in the grain on 0.01 mM NO 3 − , and 65% on 10 mM NO 3 − ). The Nitrogen Harvest Index (NHI; proportion of above-ground N localized in the grains) was between 70% and 82% (on 10 mM and 0.01 mM NO 3 − , respectively), which is comparable to values observed in wheat, barley and oilseed rape 8 , 61 , 82 , and much higher than in Arabidopsis (25–53%) 7 .
NO 3 − uptake is mediated by constitutive and inducible HATS and LATS
Due to the importance of NO 3 − uptake for grain N loading, we further investigated characteristics of NO 3 − influx. In most studied plants – such as barley, wheat, Arabidopsis and Brassica napus – root NO 3 − uptake is performed by three classes of systems: constitutive High Affinity Transport Systems (cHATS), inducible HATS (iHATS) and constitutive Low Affinity Transport System (cLATS) 26 , 83 , 84 , 85 , 86 , 87 .
N-deprived plants grown hydroponically were either induced with 1 mM NO 3 − for 24 h or remained uninduced. Root NO 3 − influx was then measured at external concentrations ranging from 0 to 10 mM. Influx was enhanced by the NO 3 − pre-treatment for all the tested concentrations, highlighting the inducibility of the uptake capacity (Fig. 6a ). The influx curves were composed of two phases, indicating the involvement of HATS and LATS. HATS was active at low NO 3 − concentration, reaching saturation at around 0.2 mM NO 3 − (Fig. 6b ). Constitutive HATS (cHATS) was active in the absence of NO 3 − pre-treatment, whereas both cHATS and inducible HATS (iHATS) contributed to the activity of HATS after NO 3 − pre-treatment (Fig. 6b ). Both cHATS and iHATS followed Michaelis-Menten kinetics, with similar Vmax and Km values (Fig. 6b,c ). The induction by NO 3 − of HATS was relatively low (factor 2.5) compared to other studies, where induction factors of 5–30 are often reported 26 . Interestingly, cHATS Vmax was higher than what has been described in wheat and barley in similar conditions (1.9 compared to 0.3–0.8 µmol N .g FW −1 .h −1 ), whereas cHATS + iHATS Vmax corresponded to the low range seen in wheat and barley (4.8 compared to 4–12 µmol N .g FW −1 .h −1 ) 26 , 83 , 88 , 89 , 90 . This suggests that the HATS is characterized by both a strong basal activity and a relatively weak inducibility by NO 3 − in Brachypodium Bd21-3. These characteristics might reveal an adaptation to N-poor natural habitats, in accordance with the origin of this accession from an arid region in Iraq, a typical ecosystem where N availability is low 91 , 92 .
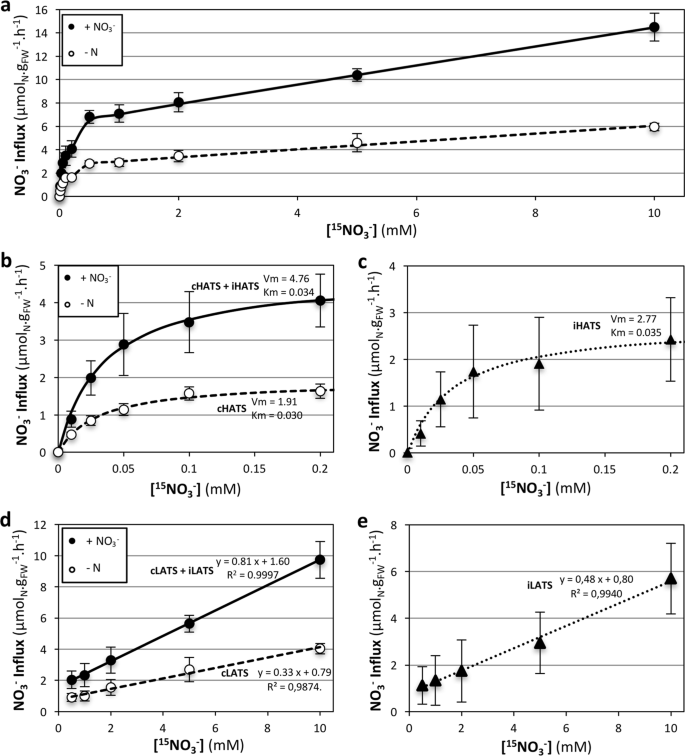
Characterization of High and Low NO 3 − Transport Systems (HATS and LATS, respectively). Plants were grown hydroponically on 2 mM NO 3 − for 7 days, then N starved for 8 days (−N, open circles and dashed lines) or N starved for 6 days and induced with 1 mM NO 3 − for 24 h (+NO 3 − , closed circles and solid lines). (a) 15 NO 3 − influx at external concentrations ranging from 0.01 to 10 mM 15 NO 3 − . (b) 15 NO 3 − influx at external concentrations ranging from 0.01 to 0.2 mM 15 NO 3 − . HATS kinetics were fitted with Michaelis-Menten curves ( Influx = Vmax * [ 15 NO 3 − ]/( Km + [ 15 NO 3 − ]). Vmax and Km values were obtained by linear regression on the Lineweaver-Burk representation ( 1/Influx = a * ( 1 /[ 15 NO 3 − ]) + b , with Vmax = 1 / b and Km = a/b ). cHATS and iHATS: constitutive and inducible HATS (respectively). (c) iHATS influx at external concentrations of 0.01–0.2 mM 15 NO 3 − , obtained by subtracting −N values from +NO 3 − values in panel b and fitted with Michaelis-Menten curve. (d) LATS 15 NO 3 − influx at external concentrations ranging from 0.5 to 10 mM 15 NO 3 − , obtained by subtracting HATS Vmax values (panel b) from corresponding influx values (panel a). LATS kinetics were fitted with linear curves. cLATS and iLATS: constitutive and inducible LATS (respectively). ( e ) iLATS curve, obtained by subtracting values from −N condition to values from +NO 3 − condition in panel d, fitted with a linear curve. Values correspond to the mean of 5 biological replicates +/−SD.
LATS activity was calculated by subtracting the Vmax values of the HATS from the corresponding measured influx in the range 0.5–10 mM NO 3 − . Surprisingly, the LATS activity was induced by NO 3 − pre-treatment (Fig. 6d ). Both constitutive LATS (cLATS) and inducible LATS (iLATS) followed a linear curve in the studied range (Fig. 6d,e ). This existence of iLATS has not been observed in most species including barley, in which extensive studies have been performed 26 , 83 , 84 , 93 . To our knowledge, an iLATS has only been unambiguously reported in wheat and in Populus tremuloides 94 , 95 . This highlights the suitability of Brachypodium Bd21-3 as a model for NO 3 − uptake systems in wheat.
HATS is regulated by N availability, likely involving BdNRT2/3 genes
High affinity NO 3 − transport systems are of prime importance for crop improvement, as ideal varieties should have a high uptake capacity at low NO 3 − availability. The regulations of HATS activity were further investigated by cultivating plants under contrasting conditions of steady state N availability or by treating them for 24 h with NH 4 NO 3 . HATS activity was then measured by transferring the plants for 5 minutes on 0.2 mM 15 NO 3 − nutrient solution. HATS influx was gradually reduced by increasing NO 3 − availability from 0.1 to 10 mM NO 3 − (Fig. 7a ). Similarly, HATS activity was repressed by the presence of ammonium (NH 4 + ) in the medium (Fig. 7b ). This is consistent with the repression of HATS by increased levels of NO 3 − or by other sources of N, described in other species 24 , 25 , 26 , 27 .
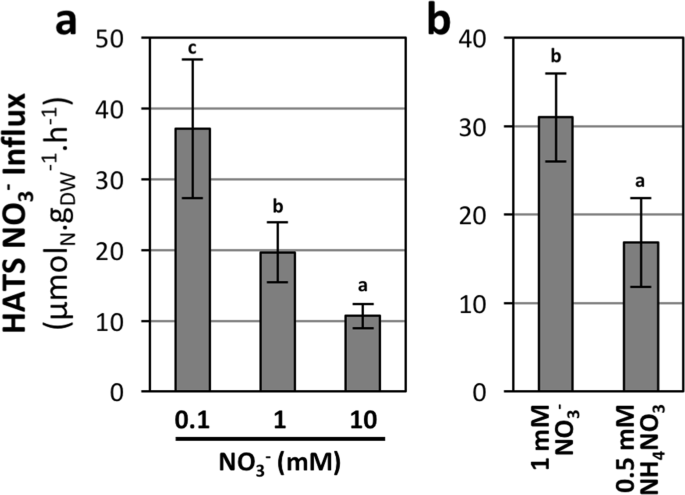
Responses of HATS to N availability. HATS was quantified at 0.2 mM 15 NO 3 − on 17-day-old plants grown hydroponically on either (a) 0.1, 1 or 10 mM NO 3 − or (b) 1 mM NO 3 − and transferred for 24 h to 1 mM NO 3 − or 0.5 mM NH 4 NO 3 . Values correspond to the mean of 5 biological replicates +/−SD. Letters indicate statistical groups (Non-parametric ANOVA, p < 0.05).
HATS has been described in several species as mediated by NRT2 transporters, in interaction with NRT3 28 , 96 . We previously identified seven NRT2 and two NRT3 genes in Brachypodium 13 . We investigated the expression of four co-orthologs ( BdNRT2A to BdNRT2D ) of the major NRT2 genes identified in Arabidopsis and barley ( AtNRT2 . 1 and HvNRT2 . 1 , respectively). Due to nearly identical coding sequences of BdNRT2A and BdNRT2B in Bd21-3 accession, these genes were quantified simultaneously. The quantification of gene expression was done by RT-qPCR in parallel to the characterization of HATS regulations (above), on independent plants. The investigated genes were more expressed in roots than in shoots (Fig. 8a ). Whereas BdNRT2C and BdNRT2D expressions were insensitive to NO 3 − availability, BdNRT2A/B were repressed by high NO 3 − (Fig. 8a ) and NH 4 + (Fig. 8b ), as observed for the HATS (Fig. 7 ). BdNRT3 genes were also mainly expressed in the roots (Fig. 8c ). Expression of BdNRT3 . 1 was unchanged under all NO 3 − supplies, whereas BdNRT3 . 2 was repressed by high NO 3 − concentrations (Fig. 8c ). BdNRT2A/B and BdNRT3 . 2 thus constitute good candidates to be involved in HATS activity in these conditions in Brachypodium Bd21-3, as they are mainly expressed in the roots, and their expression pattern follows the HATS activity.
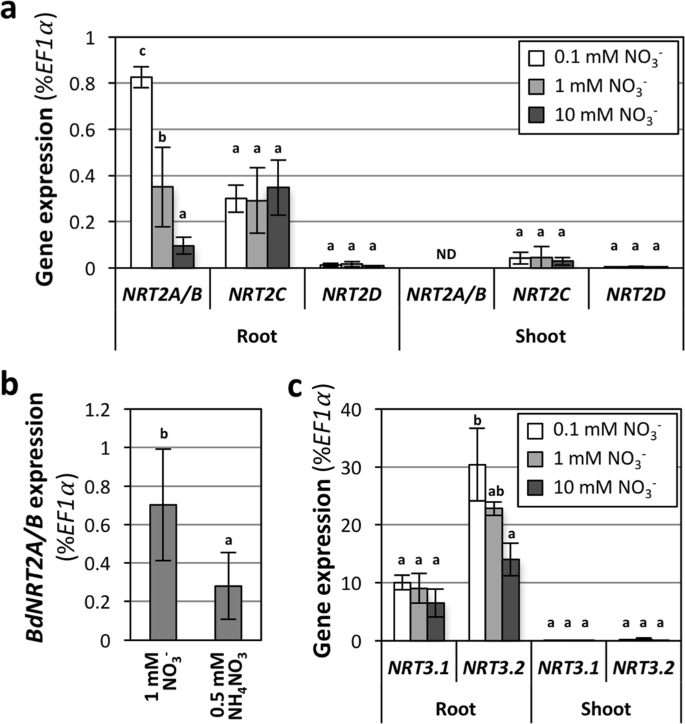
Response of BdNRT2/3 expression to N availability at vegetative stage. Plants were grown in hydroponics for 17 days under the indicated N condition. (a) Response of BdNRT2 genes to NO 3 − availability. BdNRT2A and BdNRT2B were quantified simultaneously due to high sequence homology. ND: not detected. (b) Response of BdNRT2A/B expression to NH 4 + . (c) Response of BdNRT3 genes to NO 3 − availability. Values are normalized by BdEF1α expression; similar results were obtained after normalization by BdUBC18 or BdUbi10 . Values correspond to the mean of 4–5 biological replicates +/−SD. Letters indicate statistical groups for each gene and plant organ system (ANOVA on Log2-transformed values, p < 0.05).
The expression of BdNRT2A/B and BdNRT3 . 2 was also investigated at post-anthesis stage in the experiment dedicated to the characterization of the effects of NO 3 − availability pre-anthesis and post-anthesis. BdNRT2A/B expression was stimulated by the pre-anthesis HN treatment (Fig. 9a ). In this condition, the vegetative biomass production was enhanced before anthesis (see Fig. S2B ), triggering a high post-anthesis N demand from the plant on 1 mM NO 3 − and thus explaining the stimulation of BdNRT2A/B expression. In the post-anthesis LN condition, the expression seemed also higher than in the control condition, despite being not statistically significant (Fig. 9a ). In both situations, a high N demand from the shoots could explain this stimulation, presumably leading to a raise of the HATS activity. The increased demand is, however, likely due to two different physiological situations: an increased shoot biomass in the case of the pre-anthesis HN treatment (leading to an increased grain yield) and an N deficiency in the case of the post-anthesis LN treatment (leading to decreased grain yield and GNC). No effect was observed in the other conditions, which did not affect grain yield or GNC. This suggests a functional importance of the BdNRT2A/B in the responses to NO 3 − availability. The expression of BdNRT3 . 2 was insensitive to the treatments (Fig. 9b ).
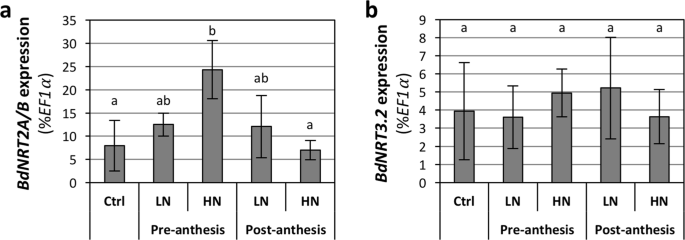
Effects of pre- and post-anthesis NO 3 − availability on root BdNRT2/3 expression at reproductive stage. Growing conditions and treatments were as described in Fig. 2 . LN, Ctrl and HN: 0.1, 1 and 10 mM NO 3 − , respectively. Plants were harvested 2 weeks after anthesis. (a) BdNRT2A/B expression. The genes were quantified simultaneously due to high sequence homology. (b) BdNRT3 . 2 expression. Values are normalized by BdEF1α expression; similar results were obtained after normalization by BdUBC18 or BdUbi10 . Values correspond to the mean of 4–5 biological replicates +/−SD. Letters indicate statistical groups (ANOVA on Log2-transformed values, p < 0.05).
Taken together, our data indicate that the developmental and physiological responses of Brachypodium (Bd21-3 accession) to N availability are comparable to previously studied C3 temperate cereals, such as wheat and barley. Specifically, NO 3 − supply affects vegetative growth, shoot/root ratio, tiller formation, spike development, grain number per plant, grain yield and grain protein content. A high NHI, similar to what has been described in major cereals, was also observed. In addition to constitutive/inducible HATS and to a constitutive LATS, an inducible LATS was identified as a component of root NO 3 − uptake systems, as previously highlighted in wheat.
Additionally, we revealed several specificities of Brachypodium Bd21-3 that are of special interest for crop improvement. For instance, understanding the basis of the unusually high GNC (despite a low N remobilization) in this model would be valuable towards an increased GPC in wheat. The post-anthesis NO 3 − uptake seems to furnish a large part of grain N in Brachypodium Bd21-3, even under low supply condition. This will need to be further investigated under different experimental conditions and/or on several Brachypodium accessions. The knowledge gained from this could subsequently be used to improve NUE in cereals. Similarly, the identification of the molecular origin of the high constitutive HATS in Brachypodium Bd21-3 would be instrumental in enhancing the NO 3 − uptake capacity of cereals in conditions of low availability. As a first insight, we identified BdNRT2/3 genes potentially involved in constitutive and/or inducible HATS component.
Overall, the data identify the Bd21-3 accession of Brachypodium as a good model to decipher, at the physiological and molecular levels, the mechanisms involved in N metabolism in C3 temperate cereals. Based on our results, it will be beneficial to characterize a set of Brachypodium accessions, in order to distinguish traits that are specific to Bd21-3 from traits conserved in the entire species. Thus, our study provides the bases for candidate-gene and natural-variability approaches to identify molecular players affecting N nutrition and NUE in Brachypodium, towards the development of new cereal varieties with enhanced NUE in low N supply conditions.
Materials and Methods
Plant material and culture conditions.
In this study, Brachypodium distachyon accession Bd21-3 was used. In the experiments, anthesis stage was defined as 7 days after emergence of the first spike 63 .
Plants grown on sand were cultivated in a growth chamber under the following conditions: 8/16 h light/dark cycle, 150 µmol photons.m −2 .s −1 irradiation, 21/17 °C day/night temperature, 80% hygrometry. The basal nutrient medium contained 0.25 mM KH 2 PO 4 , 0.25 mM MgSO 4 , 27 µM Na-Fe(III)-EDTA, 243 µM (NH 4 ) 6 Mo 7 O 24 , 0.4 µM H 3 BO 3 , 118 µM MnSO 4 , 10 µM de CuSO 4 and 34.8 µM ZnSO 4 . For 0.1 mM NO 3 − solution, the medium was supplemented with 0.1 mM KNO 3 , 0.35 mM K 2 SO 4 and 0.25 mM CaCl 2 . For 2 mM NO 3 − solution, the medium was supplemented with 1.75 mM KNO 3 , 0.125 mM Ca(NO 3 ) 2 and 0.125 mM CaCl 2 . For 10 mM NO 3 − solution, the medium was supplemented with 5 mM KNO 3 , 2.5 mM Ca(NO 3 ) 2 and 0.2 mM NaCl. Plants were provided with nutrient solution 3 times a week in excess, and flow-through was discarded. Plants were harvested 35 days after sowing, 1 h after the start of the light period. Root system and individual tillers were weighed separately, frozen in liquid nitrogen and stored at −80 °C.
Plants grown in hydroponics were cultivated in a growth chamber under the following conditions: 18/6 h light/dark cycle, 250 µmol photons.m −2 .s −1 irradiation, 22/19 °C day/night temperature, 65/90% hygrometry day/night. Grains were stratified 3–4 days at 4 °C in water, germinated in the dark at 20 °C in water for one week, and transferred to a hydroponic system composed of 15 L opaque tanks covered with perforated opaque lids. Each tank hosted 32 young plants at the beginning of the experiment, and down to 6 plants after anthesis to avoid a rapid depletion of the nutrient solution. Solution was fully renewed 2–3 times a week, and the steadiness of NO 3 − concentration was validated using MQuant TM Nitrate Test strips (Merck) and Nitrachek 404 quantification device (Quomed Ltd). Basal hydroponic solution was adapted from wheat studies 74 , 97 and contained 1 mM KH 2 PO 4 , 2 mM MgSO 4 , 5 mM KCl, 50 µM Na-Fe(III)–EDTA, 4.5 µM MnCl 2 , 10 µM H 3 BO 3 , 0.7 µM ZnCl 2 , 0.4 µM CuSO 4 , 0.22 µM MoO 4 Na 2 . N-depleted medium was supplemented with 3.25 mM CaCl 2 . The 0.01 mM NO 3 − medium was supplemented with 0.005 mM KNO 3 , 0.0025 mM Ca(NO 3 ) 2 and 3 mM CaCl 2 . The 0.1 mM NO 3 − medium was supplemented with 0.05 mM Ca(NO 3 ) 2 and 3 mM CaCl 2 . The 0.2 mM NO 3 − medium was supplemented with 0.1 mM KNO 3 , 0.05 mM Ca(NO 3 ) 2 and 3 mM CaCl 2 . The 1 mM NO 3 − medium was supplemented with 0.5 mM Ca(NO 3 ) 2 and 3 mM CaCl 2 . The 2 mM NO 3 − medium was supplemented with 1 mM KNO 3 , 0.5 mM Ca(NO 3 ) 2 and 3 mM CaCl 2 . The 10 mM NO 3 − medium was supplemented with 5 mM KNO 3 , 2.5 mM Ca(NO 3 ) 2 and 2 mM CaCl 2 and was deprived of KCl. For characterization on fresh material, plants were harvested at the middle of light period, roots were rinsed 1 min in 0.1 mM CaSO 4 , the plant parts were weighed separately, frozen in liquid nitrogen and stored at −80 °C until analyses. For end of cycle analyses, plants were harvested after full senescence, roots were rinsed 1 min in 0.1 mM CaSO 4 , roots, shoots and grains were collected separately and dried 48 h at 70 °C.
Measurement of 15 N, N and C contents and procedures for 15 N labeling
Total N, C and 15 N contents were quantified on 2–5 mg DW aliquots of ground tissues, dried at 70 °C for 48 h. The method is based on the Dumas combustion analysis which consist in a flash combustion of the samples in presence of He and O 2 at 950 °C. The C and N elements are detected by gas chromatography on a FLASH 2000 Organic Elemental Analyzer (Thermo Fisher Scientific, Villebon, France). The 15 N/ 14 N isotopic ratio is subsequently quantified by a coupled mass spectroscope (Delta V advantage IRMS; Thermo Fisher Scientific, Villebon, France).
Protocol for NO 3 − influx was adapted from Delhon and colleagues 98 . Plants grown in hydroponics were sequentially transferred to 0.1 mM CaSO 4 for 1 min and to the basal nutrient solution (see above) supplemented with 3 mM CaCl 2 and 0, 0.01, 0.025, 0.05, 0.1, 0.2, 0.5, 1, 2, 5 or 10 mM 15 NO 3 − (added as a mix of K 15 NO 3 − and Ca( 15 NO 3 ) 2 at a molar ratio 2:1; 99% 15 N atom) for 5 min. The roots were washed for 1 min in 0.1 mm CaSO 4 , shoots and roots were harvested separately, weighted and frozen in liquid nitrogen.
15 N labeling procedure and calculation for the estimation of post-anthesis N fluxes were performed as previously described 71 . Five days 15 N pulse was performed on 30 days-old plants (vegetative stage) by replacing the NO 3 − source of 0.2 mM NO 3 − medium by 15 NO 3 − (mix of K 15 NO 3 − and Ca( 15 NO 3 ) 2 at a molar ratio 2:1; 2.5% 15 N atom). Roots were subsequently rinsed 1 min in 0.1 mM CaSO 4 and plants were transferred back to a non-labeled 0.2 mM NO 3 − medium, before being transferred to 0.01, 0.2 or 10 mM NO 3 − media at anthesis stage. Plants were harvested either at anthesis (to map the 15 N distribution at the end of vegetative phase) or after full senescence of the plant. Roots, shoots and spikes were collected separately, dried 48 h at 70 °C and weighted.
Measure of NO 3 − content
Nitrate content was measured by a spectrophotometric method adapted from Miranda and colleagues 99 after extraction in water of 10 mg FW or 1–2 mg DW ground tissues. The principle of this method is a reduction of nitrate by vanadium (III) through the acidic Griess reaction. The Griess reagent is a mixed solution of VCl 3 (2.5% w/v), N-1-naphtylethylenediamine (0.05% w/v) and sulfanilamide (1% w/v) in 0.5 M HCl. It was added to plant extracts in equal proportion. After 2 hours of incubation at 60 °C, the diazoniium product was measured spectrophotometrically at 540 nm.
Amino Acid and protein analyses
Free amino acids content and composition were determined after a three-step ethanol–water extraction (80%, 50% (v/v), then water at 4 °C) on 30 mg DW (for vegetative tissues) or 50 mg DW (for grains) of ground material, according to Ferrario-Méry and colleagues 100 . Free amino acid content was quantified by ninhydrin colorimetric analysis 101 . The amino acid composition was determined by ion-exchange chromatography using the AminoTac JLC-500/V amino acid analyzer according to the instructions of the manufacturer (JEOL (Europe), Croissy-sur-Seine, France).
Protein content was determined after extraction of 50 mg DW of grain powder in 0.062 M Tris-HCl pH 6.8, and 2% SDS 102 . Extractable proteins were quantified by the the RC DC™ (reducing agent and detergent compatible) protein assay kit (Bio-Rad Laboratories, Hercules, California, USA) based on the Lowry protocol. Samples of extractable proteins were separated on 10% SDS-PAGE gels and stained with Coomassie Brilliant Blue R250 (Sigma, St Louis, MO, USA).
RNA extraction and qRT-PCR
Total RNAs were isolated using Trizol ® reagent (Ambion, Life Technologies), treated by DNase I (Thermo Scientific) and reverse-transcribed using oligo(dT) 18 primer and RevertAid H Minus Reverse Transcriptase (Thermo Scientific), according to the manufacturer’s protocols. Reactions of qPCR were performed using LightCycler-FastStart DNA Master SYBR Green I kit (Roche) on a Realplex MasterCycler (Eppendorf), according to the manufacturer’s protocol. Primer sequences are specified in Supporting Information Table S4 . Primers for housekeeping genes BdEf1 α, BdUBC18 and BdUbi10 were selected from previous report 103 . Primer efficiency (eff) was determined at each run using a standard curve on a pool of cDNA. Normalized expression value was calculated using the formula: Normalized Relative Quantity = (1/eff Ct ) Hk /(1/eff Ct ) GOI , with Hk and GOI corresponding to Housekeeping gene and Gene Of Interest 104 , respectively. Statistical analyses of qRT-PCR data were performed by ANOVA on log2-transformed values 104 .
Statistical analyses
All statistical tests have been performed with the R software, using the multcomp , coin and RVAideMemoire packages 105 , 106 , 107 . One-way ANOVA tests (qRT-PCR data) were used in conjunction with Tukey tests for the analysis of qRT-PCR data. Non-parametric one-way ANOVAs were performed using an approximate Fisher-Pitman permutation test, followed by a pairwise comparison test with the calculation of an adjusted P -value (fdr method).
Data Availability
All the relevant data supporting the findings are available from the corresponding author on reasonable request.
Alexandratos, N. & Bruinsma, J. World agriculture: towards 2015/2030: an FAO perspective. Land use policy 20 , 375 (2012).
Google Scholar
Tilman, D., Cassman, K. G., Matson, P. A., Naylor, R. & Polasky, S. Agricultural sustainability and intensive production practices. Nature 418 , 671–7 (2002).
Article ADS CAS PubMed Google Scholar
Mann, C. C. Crop Scientists Seek a New Revolution. Science (80-.). 283 , 310–316 (1999).
Article CAS Google Scholar
Kamprath, E. J., Moll, R. H. & Rodriguez, N. Effects of nitrogen fertilization and recurrent selection on performance of hybrid populations of corn. Agron. J. 74 , 955–958 (1982).
Article Google Scholar
Cassman, K. G. Ecological intensification of cereal production systems: yield potential, soil quality, and precision agriculture. Proc. Natl. Acad. Sci. USA 96 , 5952–9 (1999).
Article ADS CAS PubMed PubMed Central Google Scholar
Masclaux-Daubresse, C. et al . Nitrogen uptake, assimilation and remobilization in plants: challenges for sustainable and productive agriculture. Ann. Bot. 105 , 1141–57 (2010).
Article PubMed PubMed Central Google Scholar
Chardon, F., Noël, V. & Masclaux-Daubresse, C. Exploring NUE in crops and in Arabidopsis ideotypes to improve yield and seed quality. J. Exp. Bot. 63 , 3401–12 (2012).
Article CAS PubMed Google Scholar
Sylvester-Bradley, R. & Kindred, D. R. Analysing nitrogen responses of cereals to prioritize routes to the improvement of nitrogen use efficiency. J. Exp. Bot. 60 , 1939–51 (2009).
Gojon, A. Nitrogen nutrition in plants: rapid progress and new challenges. J. Exp. Bot. 68 , 2457–2462 (2017).
Article CAS PubMed PubMed Central Google Scholar
Dechorgnat, J. et al . From the soil to the seeds: the long journey of nitrate in plants. J. Exp. Bot. 62 , 1349–1359 (2011).
Xu, G., Fan, X. & Miller, A. J. Plant nitrogen assimilation and use efficiency. Annu. Rev. Plant Biol. 63 , 153–82 (2012).
Havé, M., Marmagne, A., Chardon, F. & Masclaux-Daubresse, C. Nitrogen remobilisation during leaf senescence: lessons from Arabidopsis to crops. J. Exp. Bot. 68 , erw365 (2017).
Girin, T. et al . Brachypodium: a promising hub between model species and cereals. J. Exp. Bot. 65 , 5683–5696 (2014).
Dawson, J. C., Huggins, D. R. & Jones, S. S. Characterizing nitrogen use efficiency in natural and agricultural ecosystems to improve the performance of cereal crops in low-input and organic agricultural systems. F. Crop. Res. 107 , 89–101 (2008).
Marschner, H. Mineral Nutrition of Higher Plants. Mineral Nutrition of Higher Plants , https://doi.org/10.1016/B978-012473542-2/50004-3 (Elsevier, 1995).
Miller, A. J., Fan, X., Orsel, M., Smith, S. J. & Wells, D. M. Nitrate transport and signalling. J. Exp. Bot. 58 , 2297–2306 (2007).
Miller, A. J. & Cramer, M. D. Root Nitrogen Acquisition and Assimilation. Plant Soil 274 , 1–36 (2005).
Huang, N., Liu, K., Lo, H. & Tsay, Y. Cloning and Functional Characterization of an Arabidopsis Nitrate Transporter Gene That Encodes a Constitutive Component of Low-Affinity Uptake. Plant Cell 11 , 1381–1392 (1999).
Filleur, S. et al . An arabidopsis T-DNA mutant affected in Nrt2 genes is impaired in nitrate uptake. FEBS Lett. 489 , 220–4 (2001).
Vidmar, J. J. et al . Regulation of high-affinity nitrate transporter genes and high-affinity nitrate influx by nitrogen pools in roots of barley. Plant Physiol. 123 , 307–18 (2000).
Yin, L.-P., Li, P., Wen, B., Taylor, D. & Berry, J. O. Characterization and expression of a high-affinity nitrate system transporter gene (TaNRT2.1) from wheat roots, and its evolutionary relationship to other NTR2 genes. Plant Sci. 172 , 621–631 (2007).
Krapp, A. et al . Expression studies of Nrt2:1Np, a putative high-affinity nitrate transporter: Evidence for its role in nitrate uptake. Plant J. 14 , 723–731 (1998).
Kiba, T. & Krapp, A. Plant nitrogen acquisition under low availability: Regulation of uptake and root architecture. Plant Cell Physiol. 57 , 707–714 (2016).
Vidmar, J. J., Zhuo, D., Siddiqi, M. Y. & Glass, A. D. M. Isolation and Characterization of HvNRT2.3 and HvNRT2.4, cDNAs Encoding High-Affinity Nitrate Transporters from Roots of Barley. Plant Physiol. 122 , 783–792 (2000).
Glass, A. D. M. et al . Nitrogen transport in plants, with an emphasis on the regulation of fluxes to match plant demand §. 1 , 199–207 (2001).
Crawford, N. M. & Glass, A. D. M. Molecular and physiological aspects of nitrate uptake in plants. Trends Plant Sci. 3 , 389–395 (1998).
Girin, T. et al . Identification of a 150 bp cis-acting element of the AtNRT2.1 promoter involved in the regulation of gene expression by the N and C status of the plant. Plant. Cell Environ. 30 , 1366–80 (2007).
Orsel, M. et al . Characterization of a two-component high-affinity nitrate uptake system in Arabidopsis. Physiology and protein-protein interaction. Plant Physiol. 142 , 1304–17 (2006).
Plett, D. et al . Dichotomy in the NRT Gene Families of Dicots and Grass Species. PLoS One 5 , e15289 (2010).
Morot-Gaudry, J.-F. Nitrogen assimilation by plants - Physiological, biochemical and molecular espects . (CRC Press, 2001).
Masclaux, C., Quilleré, I., Gallais, A. & Hirel, B. The challenge of remobilisation in plant nitrogen economy. A survey of physio agronomic and molecular approaches. Ann . Appl . 69–81 (2001).
Van Sanford, D. A. & Mackown, C. T. Cultivar Differences in Nitrogen Remobilization During Grain Fill in Soft Red Winter Wheat. Crop Sci. 300 , 295–300 (1987).
Przulj, N. & Momčilović, V. Dry matter and nitrogen accumulation and use in spring barley. 2003 , 36–47 (2003).
Gregersen, P. L., Culetic, A., Boschian, L. & Krupinska, K. Plant senescence and crop productivity. Plant Mol. Biol. 82 , 603–22 (2013).
Brevis, J. C. & Dubcovsky, J. Effects of the Chromosome Region Including the Locus on Wheat Grain and Protein Yield. Crop Sci. 50 , 93 (2010).
Uauy, C., Distelfeld, A., Fahima, T., Blechl, A. & Dubcovsky, J. A NAC Gene regulating senescence improves grain protein, zinc, and iron content in wheat. Science (80-.). 314 , 1298–301 (2006).
Article ADS CAS Google Scholar
Bogard, M. et al . Deviation from the grain protein concentration-grain yield negative relationship is highly correlated to post-anthesis N uptake in winter wheat. J. Exp. Bot. 61 , 4303–12 (2010).
Monaghan, J. M., Snape, J. W., Chojecki, A. J. S. & Kettlewell, P. S. The use of grain protein deviation for identifying wheat cultivars with high grain protein concentration and yield. Euphytica 122 , 309–317 (2001).
Ingram, P. A. et al . High-throughput imaging and analysis of root system architecture in Brachypodium distachyon under differential nutrient availability. Philos. Trans. R. Soc. Lond. B. Biol. Sci. 367 , 1559–69 (2012).
Poiré, R. et al . Digital imaging approaches for phenotyping whole plant nitrogen and phosphorus response in Brachypodium distachyon . J. Integr. Plant Biol. 56 , 781–796 (2014).
Hong, J. J. et al . Diversity of morphology and function in arbuscular mycorrhizal symbioses in Brachypodium distachyon . Planta 236 , 851–65 (2012).
Lawlor, D. W., Lemaire, G. & Gastal, F. In Plant Nitrogen (eds Lea, P. J. & Morot-Gaudry, J.-F.) 343–367, https://doi.org/10.1007/978-3-662-04064-5_13 (Springer Berlin Heidelberg, 2001).
Brouwer, R. Nutritive influences on the distribution of dry matter in the plant. Netherl. J. Agric. Sci. 10 , 361–376 (1962).
Oscarson, P. The strategy of the wheat plant in acclimating growth and grain production to nitrogen availability. J. Exp. Bot. 51 , 1921–1929 (2000).
Dupont, F. M. & Altenbach, S. B. Molecular and biochemical impacts of environmental factors on wheat grain development and protein synthesis. J. Cereal Sci. 38 , 133–146 (2003).
Beatty, P. H. et al . Nitrogen use efficiencies of spring barley grown under varying nitrogen conditions in the field and growth chamber. Ann. Bot. 105 , 1171–82 (2010).
Garcia del Moral, L., Ramos, J. & Recalde, L. Relationships between vegetative growth, grain yield and grain protein content in six winter barley cultivars. Can. J. plant Sci. 532 , 523–532 (1985).
Kichey, T., Hirel, B., Heumez, E., Dubois, F. & Le Gouis, J. In winter wheat (Triticum aestivum L.), post-anthesis nitrogen uptake and remobilisation to the grain correlates with agronomic traits and nitrogen physiological markers. F. Crop. Res. 102 , 22–32 (2007).
Taulemesse, F., Gouis, J. L., Gouache, D., Gibon, Y. & Allard, V. Bread wheat (Triticum aestivum L.) grain protein concentration is related to early post-flowering nitrate uptake under putative control of plant satiety level. PLoS One 11 , 1–21 (2016).
Gastal, F. & Lemaire, G. N uptake and distribution in crops: an agronomical and ecophysiological perspective. J. Exp. Bot. 53 , 789–799 (2002).
Justes, E., Mary, B., Meynard, J.-M., Machet, J.-M. & Thelier-Huche, L. Determination of a Critical Nitrogen Dilution Curve for Winter Wheat Crops. Ann. Bot. 74 , 397–407 (1994).
Makino, A. Photosynthesis, grain yield, and nitrogen utilization in rice and wheat. Plant Physiol. 155 , 125–9 (2011).
Jamieson, P. D., Martin, R. J. & Francis, G. S. Drought influences on grain yield of barley, wheat, and maize. New Zeal . J . Crop . Hortic. Sci. 23 , 55–66 (1995).
Fischer, R. A., Aguilar, I. & Laing, D. R. Post-anthesis sink size in a high-yielding dwarf wheat: Yield response to grain number. Aust. J. Agric. Res. 28 , 165–175 (1977).
Maidl, F., Sticksel, E., Retzer, F. & Frschbeck, G. Effect of Varied N-fertilization on Yield Formation of Winter Wheat under Particular Consideration of Mainstems and Tillers. J. Agron. Crop Sci. 180 , 15–22 (1998).
Derkx, A. P., Orford, S., Griffiths, S., Foulkes, M. J. & Hawkesford, M. J. Identification of differentially senescing mutants of wheat and impacts on yield, biomass and nitrogen partitioning. J. Integr. Plant Biol. 54 , 555–66 (2012).
Cai, S. et al . Grain protein content variation and its association analysis in barley. BMC Plant Biol. 13 , 35 (2013).
Gali, V. J. & Brown, C. G. Assisting decision-making in Queensland barley production through chance constrained programming. Aust. J. Agric. Resour. Econ. 44 , 269–287 (2000).
Branlard, G. & Dardevet, M. Diversity of grain proteins and bread wheat quality: I. Correlation between Gliadin Bands and Flour quality characteristics. J. Cereal Sci. 3 , 329–343 (1985).
Xu, Y. et al . Mapping QTLs for yield and nitrogen-related traits in wheat: influence of nitrogen and phosphorus fertilization on QTL expression. Theor. Appl. Genet. 127 , 59–72 (2014).
Barraclough, P. B. et al . Nitrogen efficiency of wheat: Genotypic and environmental variation and prospects for improvement. Eur. J. Agron. 33 , 1–11 (2010).
Sosulski, F. W. & Imafidon, G. I. Amino acid composition and nitrogen-to-protein conversion factors for animal and plant foods. J. Agric. Food Chem. 38 , 1351–1356 (1990).
Guillon, F. et al . A comprehensive overview of grain development in Brachypodium distachyon variety Bd21. J. Exp. Bot. 63 , 739–55 (2012).
Larré, C. et al . Brachypodium distachyon grain: identification and subcellular localization of storage proteins. J. Exp. Bot. 61 , 1771–83 (2010).
Martre, P., Porter, J. R., Jamieson, P. D. & Triboï, E. Modeling grain nitrogen accumulation and protein composition to understand the sink/source regulations of nitrogen remobilization for wheat. Plant Physiol. 133 , 1959–67 (2003).
Wuest, S. B. & Cassman, K. G. Fertilizer-Nitrogen Use Efficiency of Irrigated Wheat: I. Uptake Efficiency of Preplant versus Late-Season Application. Agron. J. 84 , 682–688 (1992).
Shewry, P. R. Improving the protein content and composition of cereal grain. J. Cereal Sci. 46 , 239–250 (2007).
Shewry, P. R., Tatham, A. S. & Halford, N. G. Nutritional control of storage protein synthesis in developing grain of wheat and barley. Plant Growth Regul. 34 , 105–111 (2001).
Altenbach, S. B. et al . Differential effects of a post-anthesis fertilizer regimen on the wheat flour proteome determined by quantitative 2-DE. Proteome Sci. 9 , 1–13 (2011).
Žilić, S., Barać, M., Pešić, M., Dodig, D. & Ignjatović-Micić, D. Characterization of proteins from grain of different bread and durum wheat genotypes. Int. J. Mol. Sci. 12 , 5878–5894 (2011).
Salon, C. et al . In Plant Metabolic Networks: Methods and Protocols . 1090, 335–346 (2014).
Ta, C. T. & Weiland, R. T. Nitrogen Partitioning in Maize during Ear Development. Crop Sci. 32 , 443 (1992).
Triboi, E. & Triboi-Blondel, A. M. Productivity and grain or seed composition: A new approach to an old problem. Eur. J. Agron. 16 , 163–186 (2002).
Taulemesse, F., Le Gouis, J., Gouache, D., Gibon, Y. & Allard, V. Post-Flowering Nitrate Uptake in Wheat Is Controlled by N Status at Flowering, with a Putative Major Role of Root Nitrate Transporter NRT2.1. PLoS One 10 , e0120291 (2015).
Worland, B., Robinson, N., Jordan, D., Schmidt, S. & Godwin, I. Post-anthesis nitrate uptake is critical to yield and grain protein content in Sorghum bicolor. J. Plant Physiol. 216 , 118–124 (2017).
Borrell, A. K. & Hammer, G. L. Nitrogen dynamics and the physiological basis of stay-green in Sorghum. Crop Sci. 40 , 1295–1307 (2000).
Rajcan, I. & Tollenaar, M. Source:sink ratio and leaf senescence in maize: I. Dry matter accumulation and partitioning during grain filling. F. Crop. Res. 60 , 245–253 (1999).
Barneix, A. J. Physiology and biochemistry of source-regulated protein accumulation in the wheat grain. J. Plant Physiol. 164 , 581–90 (2007).
Gooding, M. J., Gregory, P. J., Ford, K. E. & Ruske, R. E. Recovery of nitrogen from different sources following applications to winter wheat at and after anthesis. F. Crop. Res. 100 , 143–154 (2007).
Papakosta, D. K. & Gagianas, a. a. Nitrogen and Dry Matter Accumulation, Remobilization, and Losses for Mediterranean Wheat during Grain Filling. Agron. J. 83 , 864 (1991).
Bancal, P. Decorrelating source and sink determinism of nitrogen remobilization during grain filling in wheat. Ann. Bot. 103 , 1315–24 (2009).
Schjoerring, J. K., Bock, J. G. H., Gammelvind, L., Jensen, C. R. & Mogensen, V. O. Nitrogen incorporation and remobilization in different shoot components of field-grown winter oilseed rape (Brassica napus L.) as affected by rate of nitrogen application and irrigation. Plant Soil 177 , 255–264 (1995).
Siddiqi, M. Y., Glass, A. D. M., Ruth, T. J. & Rufty, T. W. Studies of the Uptake of Nitrate in Barley I. Kinetics of 13NO3- Influx. 93 , 1426–1432 (1990).
Siddiqi, M. Y., Glass, A. D. M., Ruth, T. J. & Fernando, M. Studies of the Regulation of Nitrate Influx by Barley Seedlings Using 13NO 3 − . Plant Physiol . 806–813 (1989).
Cai, C. et al . Regulation of the high-affinity nitrate transport system in wheat roots by exogenous abscisic acid and glutamine. J. Integr. Plant Biol. 49 , 1719–1725 (2007).
Le Deunff, E. & Malagoli, P. An updated model for nitrate uptake modelling in plants. I. Functional component: cross-combination of flow–force interpretation of nitrate uptake isotherms, and environmental and in planta regulation of nitrate influx. Ann. Bot. 113 , 991–1005 (2014).
Forde, B. & Clarkson, D. Nitrate and Ammonium Nutrition of Plants: Physiological and Molecular Perspectives. Adv . Bot . Res ., https://doi.org/10.1016/S0065-2296(08)60226-8 (1999).
Kalinata, P. & Nair, T. V. Variability In Kinetics Of Nitrate Uptake In Wheat Genotypes. Indian J. Plant Physiol. 6 , 411–413 (2001).
Trčkova, M., Stehno, Z. & Raimanová, I. Nitrate uptake and N allocation in Triticum aestivum L. and Triticum durum Desf. seedlings. Plant Soil Environ. 52 , 88–96 (2006).
Das, R., Jain, V., Aravind, S., Barman, M. & Srivastava, G. C. Kinetics Of Nitrate Uptake System In Wheat Genotypes. Indian J. Plant Physiol. 11 , 160–165 (2006).
CAS Google Scholar
He, M. et al . Leaf nitrogen and phosphorus of temperate desert plants in response to climate and soil nutrient availability. Sci. Rep. 4 , 6932 (2014).
Vogel, J. P. & Hill, T. High-efficiency Agrobacterium-mediated transformation of Brachypodium distachyon inbred line Bd21-3. Plant Cell Rep. 27 , 471–8 (2008).
Glass, A. D. M., Shaff, J. E. & Kochian, L. V. Studies of the Uptake of Nitrate in Barley IV. Electrophysiology. 456–463 (1992).
Pang, J., Milroy, S. P., Rebetzke, G. J. & Palta, J. A. The influence of shoot and root size on nitrogen uptake in wheat is affected by nitrate affinity in the roots during early growth. Funct. Plant Biol. 42 , 1179–1189 (2015).
Min, X., Siddiqi, M. Y., Guy, R. D., Glass, A. D. M. & Kronzucker, H. J. A comparative kinetic analysis of nitrate and ammonium influx in two early-successional tree species of temperate and boreal forest ecosystems. Plant, Cell Environ. 23 , 321–328 (2000).
Feng, H. et al . Spatial expression and regulation of rice high-affinity nitrate transporters by nitrogen and carbon status. J. Exp. Bot. 62 , 2319–2332 (2011).
Castle, S. & Randall, P. Effects of Sulfur Deficiency on the Synthesis and Accumulation of Proteins in the Developing Wheat Seed. Aust. J. Plant Physiol. 14 , 503 (1987).
Delhon, P., Gojon, A., Tillard, P. & Passama, L. Diurnal regulation of N03 uptake in soybean plants I. Changes in N03 influx, efflux, and N utilization in the plant during the day/night cycle. J. Exp. Bot. 46 , 1585–1594 (1995).
Miranda, K. M., Espey, M. G. & Wink, D. A. A Rapid, Simple Spectrophotometric Method for Simultaneous Detection of Nitrate and Nitrite. Nitric Oxide 5 , 62–71 (2001).
Ferrario-Méry, S. et al . Physiological characterisation of Arabidopsis mutants affected in the expression of the putative regulatory protein PII. Planta 223 , 28–39 (2005).
Rosen, H. A modified ninhydrin colorimetric analysis for amino acids. Arch. Biochem. Biophys. 67 , 10–15 (1957).
Guillon, F. et al . Brachypodium distachyon grain: characterization of endosperm cell walls. J. Exp. Bot. 62 , 1001–15 (2011).
Hong, S.-Y., Seo, P. J., Yang, M.-S., Xiang, F. & Park, C.-M. Exploring valid reference genes for gene expression studies in Brachypodium distachyon by real-time PCR. BMC Plant Biol. 8 , 112 (2008).
Rieu, I. & Powers, S. J. Real-Time Quantitative RT-PCR: Design, Calculations, and Statistics. Plant Cell Online 21 , 1031–1033 (2009).
Hothorn, T., Hornik, K., van de Wiel, M. A. & Zeileis, A. Implementing a Class of Permutation Tests: The coin Package. J. Stat. Softw. Novemb. 28 , 2–23 (2008).
Bretz, F., Hothorn, T. & Westfall, P. In Multiple Comparisons Using R 202, https://doi.org/10.1201/9781420010909-f (Chapman and Hall/CRC, 2011).
Hervé, M. RVAideMemoire: Testing and Plotting Procedures for Biostatistics. Available at, https://cran.r-project.org/package=RVAideMemoire (2017).
Download references
Acknowledgements
We thank Anne Marmagne for 15 N, N and C analyses, Gwendal Cueff for advices on protein analysis, and Joel Talbotec and Michel Burtin for help on plant culture. This work benefited from the support of the LabEx Saclay Plant Sciences-SPS (ANR-10-LABX-0040-SPS).
Author information
L. C. David and T. Girin contributed equally.
Authors and Affiliations
Institut Jean-Pierre Bourgin, INRA, AgroParisTech, CNRS, Université Paris-Saclay, 78000, Versailles, France
L. C. David, T. Girin, E. Fleurisson, E. Phommabouth, A. Mahfoudhi, S. Citerne, P. Berquin, F. Daniel-Vedele, A. Krapp & S. Ferrario-Méry
You can also search for this author in PubMed Google Scholar
Contributions
L.C.D., T.G., F.D.V., A.K. and S.F.M. conceived the experiments. L.C.D., T.G., E.F., E.P., A.M., S.C., P.B. and S.F.M. conducted the experiments. L.C.D., T.G. and S.F.M. analyzed the results. T.G. and S.F.M. wrote the manuscript. All authors reviewed the manuscript.
Corresponding author
Correspondence to T. Girin .
Ethics declarations
Competing interests.
The authors declare no competing interests.
Additional information
Publisher’s note: Springer Nature remains neutral with regard to jurisdictional claims in published maps and institutional affiliations.
Supplementary information
Rights and permissions.
Open Access This article is licensed under a Creative Commons Attribution 4.0 International License, which permits use, sharing, adaptation, distribution and reproduction in any medium or format, as long as you give appropriate credit to the original author(s) and the source, provide a link to the Creative Commons license, and indicate if changes were made. The images or other third party material in this article are included in the article’s Creative Commons license, unless indicated otherwise in a credit line to the material. If material is not included in the article’s Creative Commons license and your intended use is not permitted by statutory regulation or exceeds the permitted use, you will need to obtain permission directly from the copyright holder. To view a copy of this license, visit http://creativecommons.org/licenses/by/4.0/ .
Reprints and permissions
About this article
Cite this article.
David, L.C., Girin, T., Fleurisson, E. et al. Developmental and physiological responses of Brachypodium distachyon to fluctuating nitrogen availability. Sci Rep 9 , 3824 (2019). https://doi.org/10.1038/s41598-019-40569-8
Download citation
Received : 08 May 2018
Accepted : 12 February 2019
Published : 07 March 2019
DOI : https://doi.org/10.1038/s41598-019-40569-8
Share this article
Anyone you share the following link with will be able to read this content:
Sorry, a shareable link is not currently available for this article.
Provided by the Springer Nature SharedIt content-sharing initiative
This article is cited by
Nitric oxide synthase expression in pseudomonas koreensis mme3 improves plant growth promotion traits.
- María M. Labarthe
- Guillermo A. Maroniche
- Cecilia M. Creus
Applied Microbiology and Biotechnology (2024)
Enriched root bacterial microbiome in invaded vs native ranges of the model grass allotetraploid Brachypodium hybridum
- Brooke Pickett
- Chelsea J. Carey
- Emma L. Aronson
Biological Invasions (2022)
Impact of geomagnetic disturbances on power transformers: risk assessment of extreme events and data availability
- Patrik Hilber
- Ebrahim Shayesteh
Life Cycle Reliability and Safety Engineering (2022)
Spatial analysis of the root system coupled to microbial community inoculation shed light on rhizosphere bacterial community assembly
- Shaodong Wei
- Samuel Jacquiod
- Søren Johannes Sørensen
Biology and Fertility of Soils (2021)
By submitting a comment you agree to abide by our Terms and Community Guidelines . If you find something abusive or that does not comply with our terms or guidelines please flag it as inappropriate.
Quick links
- Explore articles by subject
- Guide to authors
- Editorial policies
Sign up for the Nature Briefing newsletter — what matters in science, free to your inbox daily.


An official website of the United States government
The .gov means it’s official. Federal government websites often end in .gov or .mil. Before sharing sensitive information, make sure you’re on a federal government site.
The site is secure. The https:// ensures that you are connecting to the official website and that any information you provide is encrypted and transmitted securely.
- Publications
- Account settings
Preview improvements coming to the PMC website in October 2024. Learn More or Try it out now .
- Advanced Search
- Journal List
- Front Plant Sci
Improving the effects of drought priming against post-anthesis drought stress in wheat ( Triticum aestivum L.) using nitrogen
Attiq ullah.
1 Key Laboratory of Crop Physiology, Ecology and Production Management, Nanjing Agricultural University, Nanjing, China
Zhongwei Tian
Muhammad abid.
2 Department of Soil Conservation, Narowal, Pakistan
Anab Khanzada
Muhammad zeeshan.
3 Key Laboratory of Crop Cultivation and Tillage, Agricultural College of Guangxi University, Nanning, China
Chuanjiao Sun
Associated data.
The original contributions presented in this study are included in the article/supplementary material, further inquiries can be directed to the corresponding author.
Water and nitrogen (N) deficiencies are the major limitations to crop production, particularly when they occur simultaneously. By supporting metabolism, even when tissue water capacity is lower, nitrogen and priming may reduce drought pressure on plants. Therefore, the current study investigates the impact of nitrogen and priming on wheat to minimize post-anthesis drought stress. Plant morphology, physiology, and biochemical changes were observed before, during, and after stress at the post-anthesis stage. The plants were exposed to three water levels, i.e., well watering (WW), water deficit (WD), and priming at jointing and water deficit (PJWD) at the post-anthesis stage, and two different nitrogen levels, i.e., N180 (N1) and N300 (N2). Nitrogen was applied in three splits, namely, sowing, jointing, and booting stages. The results showed that the photosynthesis of plants with N1 was significantly reduced under drought stress. Moreover, drought stress affected chlorophyll (Chl) fluorescence and water-related parameters (osmotic potential, leaf water potential, and relative water content), grain filling duration (GFD), and grain yield. In contrast, PJWD couple with high nitrogen treatment (N300 kg ha –1 ) induced the antioxidant activity of peroxidase (37.5%), superoxide dismutase (29.64%), and catalase (65.66%) in flag leaves, whereas the levels of hydrogen peroxide (H 2 O 2 ) and superoxide anion radical (O 2 – ) declined by 58.56 and 66.64%, respectively. However, during the drought period, the primed plants under high nitrogen treatment (N300 kg ha –1 ) maintained higher Chl content, leaf water potential, and lowered lipid peroxidation (61%) (related to higher activities of ascorbate peroxidase and superoxide dismutase). Plants under high nitrogen treatment (N300 kg ha –1 ) showed deferred senescence, improved GFD, and grain yield. Consequently, the research showed that high nitrogen dose (N300 kg ha –1 ) played a synergistic role in enhancing the drought tolerance effects of priming under post-anthesis drought stress in wheat.
Introduction
Plant growth and development are greatly associated with the available resources, such as nitrogen, water, and light. Studies have shown that water and nitrogen deficiencies alter numerous morphological and physiological processes in plants ( Wang et al., 2021 ). Water scarcity is viewed as the most limiting factor to plant development and yield ( Siddiqui et al., 2015 ; Jabborova et al., 2021 ). Wheat ( Triticum aestivum L.) is considered to be the largest cultivated cereal crop in the world and is extremely sensitive to drought stress, which regularly occurs at the post-anthesis stage and leads to significant yield losses ( Cossani and Reynolds, 2012 ; Wang et al., 2014a ). Drought stress induced during the post-anthesis stage of the wheat crop either halts grain filling or causes the grain to remain completely unfilled ( Dhanda and Sethi, 2002 ), as grain filling is predominantly a connected source–sink relationship ( Thakur et al., 2010 ). In cereals, photosynthesis of the flag leaf and inflorescence during post-anthesis plays a crucial role in grain development ( Murchie et al., 2002 ). Therefore, improving drought tolerance during post-anthesis in wheat is crucial for sustaining food security in the present global climate scenario.
The recent global climate events are continuously impacting agriculture, threatening global food security. Drought stress is the primary factor affecting plant metabolism and disturbing the photosynthesis machinery ( Ahmad S. et al., 2021 ; Nardino et al., 2022 ). In the process, drought stress reduces the stomata conductance to restrict water losses during evapo-transpiration and reduces the CO 2 in the grass ( Gaikwad et al., 2020 ), subsequently disturbing photosystem II (Fv/Fm) ( Grassi and Magnani, 2005 ). In fact, the effects of drought on wheat crops are multilayered, such as turgor loss, osmotic imbalance, and shrinkage in cell volume, as well as reduced membrane stability and accumulation of ROS ( Batra et al., 2014 ; Liu et al., 2015 ; Ahmad A. et al., 2021 ). Moreover, the failure of plants to scavenge ROS induces oxidative stress, which leads to membrane lipid peroxidation, protein oxidation, hormonal imbalance, and finally, cell death ( Niu et al., 2013 ; Liu et al., 2015 ). Drought tolerance is a complex quantitative trait ( Wu et al., 2022 ), and plants deploy multiple strategies to combat drought ( Ahmad et al., 2019 ). These include osmoprotectants, enzymatic and non-enzymatic antioxidants, biomarkers, and secondary metabolism-related enzymes ( Zeeshan et al., 2020 ; Siddiqui et al., 2021 ; Salam et al., 2022 ).
Previous studies indicated that pre-stress priming had a positive effect on plants by activating faster and more proficient defense mechanisms against the subsequent stress circumstances ( Abid et al., 2016b ; Wang et al., 2018 ). Pre-stress priming, which is known as “stress memory” ( Wang et al., 2018 ), could increase stress tolerance ( Abid et al., 2016b ). Previous experiments showed that induction of drought priming at the vegetative stage and/or pre-anthesis stage enhanced heat and/or drought tolerance during the grain filling stage in Triticum aestivum L. ( Wang et al., 2014a , b ) and enhanced freezing tolerance in wheat at the jointing stage ( Li et al., 2015 ). Similarly, high-temperature stress at the post-anthesis stage was relieved in response to drought priming in wheat, as evident by enhanced photosynthetic activity and mitigation of oxidative stress ( Zhang et al., 2016 ). Moreover, higher net photosynthesis along with dry biomass and grain yield was observed in response to the multiple water-logging priming induced at three different growth stages in wheat ( Li et al., 2011 ). Therefore, inducing priming at different growth stages against the corresponding stresses occurring during post-anthesis was found to be an effective tool for stress mitigation. But it should be noted that drought stress levels change during the vegetative development period of wheat, and therefore the effects of drought priming against post-anthesis drought stress require further investigation. Additionally, previous studies did not investigate the use of high nitrogen fertilizer along with pre-stress priming to enhance drought tolerance.
Nitrogen is a significant underlying part of nucleic acids, proteins, chlorophyll, rubisco, and some hormones, and can decrease drought stress damage by maintaining metabolic activities ( Zhang et al., 2007 ; Ata-Ul-Karim et al., 2016 ). Abid et al. (2016a) suggested that proper nitrogen supply can improve plant drought tolerance in wheat. Studies reported that the utilization of nitrogen fertilizer relieves the negative impact of drought stress ( Saneoka et al., 2004 ; Yang et al., 2012 ). Morgan (1986) suggested that nitrogen-fertilized wheat plants respond faster to drought stress by closing stomata and reducing net photosynthesis. Shabbir et al. (2016) found that a combined foliar spray of additional nitrogen affected the addition of osmoprotectants and the activities of nitrogen assimilation and antioxidant enzymes, thereby improving wheat yield and drought tolerance. Moreover, water limitation and low nitrogen supply are the main limiting factors for wheat yield. It is widely reported that both factors affect leaf–water relationship, chlorophyll fluorescence, and photosynthetic processes, thereby limiting the rate of plant development, inducing early senescence, and shortening the GFD period, thus causing limited grain weight and poor crop productivity ( Madani et al., 2010 ; Mobasser et al., 2014 ). However, Li et al. (2011) revealed that under drought stress, applying nitrogen prevents different grass species from changing their physiological functions. These varying outcomes may be accredited to differences in particular environments, species characteristics, drought stress levels, nitrogen, timing, or different growth phases of the crops. Even under constant water and nitrogen levels, the response of plants to drought stress varies at various phases of crop development ( Ernst et al., 2010 ; Shi et al., 2014 ). In addition, despite its significance, very few studies can completely compare the effects of different levels of drought stress on grain yield traits at different vegetative stages of wheat. Furthermore, the tandem use of high nitrogen fertilizer and priming to understand the plant ecophysiological mechanisms of drought resistance has not been explored. Therefore, an appropriate estimation of the effects of drought priming against post-anthesis drought stress using nitrogen on the physiological activities and yield attributes will provide significant insight into the cultivation of wheat under adverse conditions ( Teixeira et al., 2014 ).
In this study, we hypothesized that nitrogen application and pre-anthesis drought priming might lessen the effects of post-anthesis drought stress in wheat. It was further hypothesized that an appropriate increase in nitrogen application in water deficit conditions would enhance the drought tolerance of the wheat crops.
Materials and methods
Experimental design.
To assess the combined effects of nitrogen and pre-drought priming on the wheat plants during post-anthesis drought stress, a rain cover pot experiment was conducted at the Pailou Experimental Research Station of Nanjing Agricultural University, China (32°040 N, 118°760 E), during the growing period of 2018–2019. Plants were sown at a shallow depth of 3–5 cm in plastic pots, i.e., 25 cm high and 22 cm in diameter, and filled with 10 kg of clay loam air-dried soil (bulk density; 1.29 g cm –3 ), sieved with a 0.5-mm mesh, uniformly mixed, and placed in a shade house under natural light conditions. Meanwhile, at the time of pot filling, 0.5 g of P 2 O 5 and 1.1 g of K 2 O per pot were mixed with soil. Two wheat varieties, i.e., Yangmai-158 (medium gluten) and Yangmai-22 (low gluten), were seeded as experimental materials. Prior to sowing, seeds were disinfected with 1% (v/v) H 2 O 2 and then washed three times using ddH 2 O. Fourteen seeds of each variety were sown in each pot and were arranged in a completely randomized design (CRD) with a factorial arrangement. The experimental design is presented in Figure 1 . At emergence, seven uniform seedlings were left after thinning in all pots for subsequent experimental measurements and analysis. The details of the atmospheric temperature of the experimental area are given in Table 1 .

Experimental plan for the study: drought priming (P) was applied at jointing (J) by exposing the plants of two wheat cultivars (Yangmai-158 and Yangmai-22) to moderate drought stress at 55–60% field capacity (FC) for 10 days followed by re-watering. Meanwhile, non-priming plants (WW) were subjected to continued watering at 75–80% FC. At the post-anthesis stage, moderate drought stress at 55–60% FC was applied from 7 to 14 days after post-anthesis followed by re-watering. The treatments during post-anthesis drought stress were assigned as PJWD priming at jointing + post-anthesis drought stress, WD no priming at jointing + post-anthesis drought stress, and WW no priming at jointing + no post-anthesis drought stress. After priming at jointing and application of post-anthesis drought stress treatments, the plants were re-watered directly at 75%–80% FC for recuperation.
Average temperatures during growing seasons of 2018–2019.
Treatments and applications
The experiment consisted of two wheat varieties, i.e., Yangmai-158 (YM-158) and Yangmai-22 (YM-22), and three water levels, i.e., well watering (WW), water deficit (WD), and priming at jointing and water deficit (PJWD) at post-anthesis stage. The experiment included two different nitrogen levels, i.e., N180 kg ha –1 (N1) and N300 kg ha –1 (N2). There were 12 treatments and 33 pots as replicates per treatment (2 variety* 3 water level* 2 nitrogen* 33 replications = 396 pots in total). Drought priming was induced at the jointing stage, and irrigation of pots was suspended until a moderate drought stress level from 55 to 60% field capacity (FC) was reached. This moderate drought stress level was maintained for 10 days as priming by compensating for water lost every day. Meanwhile, the control pots were kept on irrigation under well-watered conditions. After the completion of priming, the pots were re-watered to the level of control pots (78–80% FC) until the application ensured post-anthesis drought stress. Seven days after the anthesis appeared, plants were exposed to drought stress (at 30–40% field capacity) and re-watered to 78–80% FC till harvesting after the complication of treatment duration. The soil moisture content of each treatment was measured daily using an HH2 Moisture Meter (Delta-T Devices, Cambridge, United Kingdom). For N treatment, nitrogen fertilizer in the form of urea nitrogen [CO(NH 2 ) 2 ] was applied in three splits: 50% N of each level was applied at the time of sowing, another 30% N at jointing, and another 20% at booting. The quantity of water required for pot irrigation was determined using the procedure suggested by a previous study ( Abid et al., 2016a ).
where W is the quantity of water irrigation, H is the depth of soil, A is the vicinity of pot, Y is the bulk density of the soil, FC1 is the top edge of the needed soil FC, and FC0 is the real soil FC before irrigation.
Plant selection and trait measurement
At the post-anthesis stage, the uniform tillers/spikes from all treatments were tagged at the same time for testing and measurements. The topmost fully expended flag leaves were sampled from the three chosen pots (considered as one replication) of each treatment 1 day before the beginning of drought stress (0 DS), 5 days after drought stress (5 DS), and 5 and 10 days after re-watering (5 DRW and 10 DRW) for the determination of physiological and biochemical parameters. Plants in each pot were utilized only once per sample at each time point and then were discarded. All plant samplings and photosynthesis measurements were performed between 9:00 a.m. and 11:00 a.m. local time. The samples collected for protein assay and other related parameters were kept at –80°C until further determination.
Osmotic potential, leaf water potential, and leaf relative water content
The flag was selected for measurement of leaf relative water content (LRWC) from each treatment in three replications as indicated by a previous study ( Barrs and Weatherley, 1962 ). The LRWC equation is as follows:
where FW indicates the fresh weight of leaf, TW indicates the fresh weight of turgid dipped leaves in distal water after drenching in the darkness for 24 h, and DW indicates the dry weight of leaves after dehydration at 75°C to a stable weight (oven drying).
The osmotic potential was resolved by following the technique of a previous study ( Ribaut and Pilet, 1991 ). The novel completely enlarged leaf was set in the nitrogen fluid at a defrosted point (25°C), and the cell sap liquid was separated by compacting the leaf in a syringe. The osmotic concentration was estimated using the fume osmometer pressure (Wescor Vapor 5600, United States) and determined using the van’t Hoff condition.
The leaves were immobilized in the fixed cover of the sample holder, and pressure was applied until liquid flowed from the uncovered end of the leaf stalk. At this point, careful perusing was noted, which demonstrated that water was held inside the leaf with negative power, expressed as –MPa.
Leaf gas exchange measurements
At the post-flowering stage, IRGA Li-COR portable photosynthesis system (LI-6400 Inc., Lincoln, NE, United States) was used to measure gas exchange measurements in the flag leaf. Photosynthesis was measured at 1,000 μmol photo m –2 s –1 at 25°C and 400 μmol mol –1 CO 2 (Ca). The light saturation net CO 2 assimilation rate (Pn) and stomatal conductivity ( gs ) were determined.
Chlorophyll fluorescence measurements
The measurements of chlorophyll fluorescence were performed using a modulated fluorimeter (FMS2; Hansatech, King’s Lynn, Norfolk, United Kingdom). Prior to measurements, the topmost fully expanded leaf that was uniformly oriented and fully exposed to light was kept in the dark for 30 min, and then exposed to light (∼1,000 m mol photons m –2 s –1 ) to record the ground state fluorescence (F0) and the maximum fluorescence (Fm). At this point, the steady-state fluorescence value (Fs) and maximum fluorescence (Fm’) of the light adaptation level were measured. Next, the actinic light was turned off, and the leaf was illuminated with far-red light for 3 s to determine the minimum fluorescence in the light-adapted state (F0’). Based on these standards, according to the recommendations from Maxwell and Johnson (2000) , the fluorescence was determined using the maximum efficiency of PSII (Fv/Fm) and the effective quantum yield of PSII (ΦPSII).
Chlorophyll and soluble protein content measurements
A handheld SPAD 502 Chl meter (Soil Plant Analysis Development; Minolta, Japan) was utilized to measure the chlorophyll content (SPAD) of flag leaves of each treatment. The SPAD readings were taken from five flag leaves of each pot, averaged, and considered as one replication. Similarly, three replications were taken per treatment, and the averaged values were taken as the chlorophyll content.
To quantify the total soluble protein content, fresh leaves (0.5 g) were ground in sodium phosphate solution (50 mM, pH 7.0). The amalgam was centrifuged at 4,000 × g at 4°C for 10 min. In order to measure the soluble proteins, bovine serum albumin was used to normalize the sample supernatant, and the absorbance value was recorded spectroscopically at 595 nm.
Superoxide production, malondialdehyde content, hydrogen peroxide content, and antioxidant enzyme activity
The O 2 – content was estimated according to the method suggested by Sui et al. (2007) . The H 2 O 2 and malondialdehyde (MDA) contents were determined according to the method by Zheng et al. (2009) . The activity of superoxide dismutase (SOD, EC 1.15.1.1) was assessed as suggested by Yang et al. (2007) , and the activity of peroxidase (POD, EC 1.11.1.7) was determined according to the method by Zheng et al. (2009) . The catalase activity (CAT, EC 1.11.1.6) was assayed using the method of Tan et al. (2008) .
Flag leaf area and weight, grain filling duration, and yield component determination
At the post-anthesis stage, the LI-3000 area meter (Li-Cor. Inc., Lincoln, NE, United States) was used to measure the green flag leaf area during drought stress treatment (WD). The specific leaf weight was calculated as follows:
where WL is the flag leaf weight and AL is the flag leaf area.
When half of the spikes under the treatment had reached grain maturity, the grain filling duration (GFD) (the number of days from blossoming to grain development) was recorded. At maturity, three pots were haphazardly chosen from every treatment to ascertain the number of tillers with spikes, the number of grains/spikes, spike length, the weight of 1,000 grains, grain yield/pot, plant biomass, and drought index (DI). The DI was determined as the proportion of grain yield under drought stress (YD) and grain yield under WW development (YW).
Statistical analysis
An analysis of variance (ANOVA) was performed by utilizing the general linear model (GLM) program to see the effects of various nitrogen levels, priming, and post-flowering drought stress on the physiological, morphological, and enzymatic exercises. The mean values for treatments were compared with the least significant difference (LSD t -test was applied to test the treatment differences.) with a probability of 0.05 (SPSS Inc., Chicago, IL, United States). The figures were drawn using Sigma Plot 10.
Leaf water potential, osmotic potential, and leaf relative water content
The leaf water potential (Ψw), osmotic potential (Ψs), and LRWC of flag leaves were measured at the post-anthesis stage and presented in Figures 2A–F . A decreasing trend of these parameters was observed from 12 to 14 days after anthesis (DAA) in both cultivars. The maximum value (less negative) of Ψw and Ψs was recorded in WW, PJWD, and WD under N2 supplementation. The Ψw, Ψs, and LRWC showed a gradual decrease in drought-stressed plants. Moreover, there was a significant difference in Ψw, Ψs, and LRWC in both N levels dose-dependently and WW, and there was also a significant difference between treatments under PJWD and WD conditions. The Ψw, Ψs, and LRWC values declined further under N1 compared to N2. The effect of drought stress decreased with higher nitrogen levels. For instance, N2 showed high performance as compared to N1 in WW, PJWD, and WD. Furthermore, under the post-anthesis drought stress, Ψw, Ψs, and LRWC were less reduced in primed plants when compared to the non-primed plants in both cultivars. After re-watering, the Ψw, Ψs, and LRWC recovered differently under different water and N levels. The drought-stressed plants showed better recovery in Ψw, Ψs, and LRWC under N2 when compared with N1.

Effects of pre-drought priming on leaf water potential (Ψw), osmatic potential (Ψs), and leaf relative water content (LRWC) in response to post-anthesis drought stress under two nitrogen (N) rates (N1,180 and N2,300) in Yangmai-158 (A,C,E) and Yangmai-22 (B,D,F) wheat cultivars. Treatments: WW (control), no priming at jointing + no post-anthesis drought stress; PJWD, priming at jointing + post-anthesis drought stress; and WD, no priming at jointing + post-anthesis drought stress. Pre-drought priming was completed during the jointing stage, and post-anthesis drought stress was applied from 7 to 14 DAA. The horizontal axis shows the time course of the sampling: 1 day before drought stress (0DS), 5 days after drought stress (5DS), 5 and 10 days after re-watering (5DRW and 10DRW). Each vertical bar above the mean values indicates the standard error of three replicates ( n = 3) by using three-way ANOVA at P < 0.05.
Gas exchange parameters
The level of photosynthesis was maximum from 12 to 14 DAA in both cultivars, and then it started to decrease gradually after 14 days post-anthesis. Analysis showed that the photosynthesis rate (Pn) and stomatal conductance (gs) were reduced under post-anthesis WD and PJWD treatments relative to the WW treatment. However, under WD, the photosynthesis (Pn), and stomatal conductance (gs) were reduced relative to PJWD in YM-158 and YM-22 varieties. Lower Pn and gs were observed in WW, WD, and PJWD conditions under N1 compared to N2 ( Figures 3A–D ). The Pn and gs were influenced more under drought stress than priming treatments when compared to WW, depending on the N level. After re-watering, the PJWD plants showed higher recovery than the WD plants in both cultivars. Furthermore, slow recovery was observed under N1 compared to N2. The results indicated that higher nitrogen and priming have positive effects on Pn and gs under drought conditions.

Effects of pre-drought priming on net photosynthetic rate (Pn) and stomatal conductance (gs) in response to post-anthesis drought stress under two nitrogen (N) rates (N1,180 and N2, 300) in Yangmai-158 (A,C) and Yangmai-22 (B,D) wheat cultivars. Treatments: WW (control), no priming at jointing + no post-anthesis drought stress; PJWD, priming at jointing + post-anthesis drought stress; and WD, no priming at jointing + post-anthesis drought stress. Pre-drought priming was completed during the jointing stage, and post-anthesis drought stress was applied from 7 to 14 days after anthesis. The horizontal axis shows the time course of the sampling: 1 day before drought stress (0DS), 5 days after drought stress (5DS), and 5 and 10 days after re-watering (5DRW and 10DRW). Each vertical bar above the mean value indicates the standard error of three replicates ( n = 3) by using three-way ANOVA at P < 0.05.
The decline in Fv/Fm and Φ PSII values started from 12 to 14 DAA. There was a significant alteration in Fv/Fm and Φ PSII rates in N1 and N2 under WW, WD, and PJWD conditions. Drought-stressed WD significantly decreased the Fv/Fm and Φ PSII rates of flag leaves, but PJWD in both cultivars maintained higher Fv/Fm and Φ PSII values than WD. On the other hand, N2 supplement plants showed better stability and reduced effects of drought stress on these parameters than N1 ( Figures 4A–D ). The Fv/Fm and Φ PSII rates in drought-stressed plants also varied from the rates of WW under drought stress and N treatments. The decline in Fv/Fm and Φ PSII was more prominent in WD under N1 compared to N2. After re-watering, the value of Fv/Fm and Φ PSII increased. The primed plants showed higher recovery rates than the non-primed plants in both varieties, and N2 recovered quicker than N1.

Effects of pre-drought priming on the quantum efficiency of the photochemical reaction in PSII (Fv/Fm) and the quantum yield of the PSII electron transport (ΦPSII), in response to post-anthesis drought stress under two nitrogen (N) rates (N1,180 and N2, 300) in Yangmai-158 (A,C) and Yangmai-22 (B,D) wheat cultivars. Treatments: WW (control), no priming at jointing + no post-anthesis drought stress; PJWD, priming at jointing + post-anthesis drought stress; and WD, no priming at jointing + post-anthesis drought stress. Pre-drought priming was completed during the jointing stage, and post-anthesis drought stress was applied from 7 to 14 days after anthesis. The horizontal axis shows the time course of the sampling: 1 day before drought stress (0DS), 5 days after of drought stress (5DS), and 5 and 10 days after re-watering (5DRW and 10DRW). Each vertical bar above the mean value indicates the standard error of three replicates ( n = 3) by using three-way ANOVA at P < 0.05.
The Chl and soluble protein content showed significant differences in all three water levels and N doses ( Figures 5A–D ). The content of Chl and soluble protein was significantly affected under the WD treatments depending on the strength of WD and N application doses. WD and PJWD significantly decreased the value of Chl and soluble protein in both wheat cultivars compared with WW. The plants under N1 showed a lesser value of Chl and soluble protein content compared to N2. Chlorophyll and soluble protein content under N2 showed more stability in WW, WD, and PJWD treatments compared to N1. After re-watering, the recovery of Chl and soluble protein was slower in N1 than in N2.

Effects of pre-drought priming on chlorophyll (SPAD value) and soluble protein content in response to post-anthesis drought stress under two nitrogen (N) rates (N1,180 and N2, 300) in Yangmai-158 (A,C) and Yangmai-22 (B,D) wheat cultivars. Treatments: WW (control), no priming at jointing + no post-anthesis drought stress; PJWD, priming at jointing + post-anthesis drought stress; and WD, no priming at jointing + post-anthesis drought stress. Pre-drought priming was completed during the jointing stage, and post-anthesis drought stress was applied from 7 to 14 days after anthesis. The horizontal axis shows the time course of the sampling: 1 days before drought stress (0DS), 5 days after drought stress (5DS), and 5 and 10 days after re-watering (5DRW and 10DRW). Each vertical bar above the mean value indicates the standard error of three replicates ( n = 3) by using three-way ANOVA at P < 0.05.
Superoxide, hydrogen peroxide, and malondialdehyde content
The O 2 – , H 2 O 2 , and MDA content increased rapidly in drought stress and in priming plants when compared to the WW plants at the post-anthesis stage. Significant differences in the concentrations of O 2 – , H 2 O 2 , and MDA were observed among the N levels in the WW, WD, and PJWD conditions dose-dependently. However, the concentration of these parameters decreased with increasing N levels in the WD and PJWD treatments. Therefore, in N2, the magnitude of O 2 – , H 2 O 2 , and MDA increment was less than N1 ( Figures 6A–F ). Compared to drought stress treatment, the primed plants showed less accumulation of O 2 – , H 2 O 2 , and MDA. Similarly, cultivar YM-158 showed higher content of O 2 – , H 2 O 2 , and MDA than that of YM-22, which suggested that YM-158 is more sensitive to drought than YM-22. After re-watering, the accumulation of O 2 – , H 2 O 2 , and MDA decreased, particularly in the primed and N2 plants, when compared with drought-stressed and N1-supplemented plants.

Effects of pre-drought priming on malondialdehyde (MDA), hydrogen peroxide (H 2 O 2 ), and superoxide anion radical (O 2 – ) content in response to post-anthesis drought stress under two nitrogen (N) rates (N1, 180 and N2, 300) in Yangmai-158 (A,C,E) and Yangmai-22 (B,D,F) wheat cultivars. Treatments: WW (control), no priming at jointing + no post-anthesis drought stress; PJWD, priming at jointing + post-anthesis drought stress; and WD, no priming at jointing + post-anthesis drought stress. Pre-drought priming was completed during the jointing stage, and post-anthesis drought stress was applied from 7 to 14 days after anthesis. The horizontal axis shows the time course of the sampling: 1 day before drought stress (0DS), 5 days after drought stress (5DS), and 5 and 10 days after re-watering (5DRW and 10DRW). Each vertical bar above the mean value indicates the standard error of three replicates ( n = 3) by using three-way ANOVA at P < 0.05.
Activities of anti-oxidative enzymes
The POD, SOD, and CAT activities were significantly reduced under drought stress and priming treatments compared to the WW treatment ( Figures 7A–F ). There were significant differences in SOD, POD, and CAT activities between different N doses and water levels. In the WD condition, the POD, SOD, and CAT showed significant differences. In contrast, nitrogen treatment dose-dependently increased the activities of SOD, POD, and CAT in primed plants under drought stress treatment. After re-watering, the N2 plants recovered quickly when compared to N1 plants. The maximum value of all of these parameters was observed in the control treatment with N2. Under post-anthesis drought stress, the SOD, POD, and CAT activities slightly declined in primed plants when compared to the non-primed plants in both cultivars.

Effects of pre-drought priming on catalase (CAT), peroxidase (POD), and superoxide dismutase (SOD) content in response to post-anthesis drought stress under two nitrogen (N) rates (N1, 180 and N2, 300) in Yangmai-158 (A,C,E) and Yangmai-22 (B,D,F) wheat cultivars. Treatments: WW (control), no priming at jointing + no post-anthesis drought stress; PJWD, priming at jointing + post-anthesis drought stress; and WD, no priming at jointing + post-anthesis drought stress. Pre-drought priming was completed during the jointing stage, and post-anthesis drought stress was applied from 7 to 14 days after anthesis. The horizontal axis shows the time course of the sampling: 1 day before drought stress (0DS), 5 days after drought stress (5DS), and 5 and 10 days after re-watering (5DRW and 10DRW). Each vertical bar above the mean value indicates the standard error of three replicates ( n = 3) by using three-way ANOVA at P < 0.05.
Flag leaf area and specific leaf weight
In the post-flowering stage, during the drought stress period, the flag leaf area and specific leaf weight of YM-22 were higher than those of YM-158 under WW, WD, and PJWD conditions ( Figures 8A,B ). The drought stress decreased flag leaf area and weight, while nitrogen and priming improved the flag leaf area and weight. The minimum flag leaf area and weight were observed in N1 under the WD condition. The maximum flag leaf area was recorded in WW with N2 in both cultivars. PJWD enhanced the flag leaf area and specific leaf weight more than WD. These outcomes showed that pre-anthesis PJWD treatment could bring a superior change in leaf morphology under post-anthesis WD conditions, supporting the accumulation of photosynthetic products.

Effects of pre-drought priming on flag leaf area and specific leaf weight in response to post-anthesis drought stress under two nitrogen (N) rates (N1, 180 and N2, 300) in Yangmai-158 and Yangmai-22 (A) , and Yangmai-158 and Yangmai-22 (B) wheat cultivars. Treatments: WW, (control) no priming at jointing + no post-anthesis drought stress; PJWD, priming at jointing + post-anthesis drought stress; and WD, no priming at jointing + post-anthesis drought stress. Pre-drought priming was completed during the jointing stage, and post-anthesis drought stress was applied from 7 to 14 days after anthesis. Each vertical bar above the mean value indicates the standard error of three replicates ( n = 3) by using three-way ANOVA at P < 0.05.
Grain filling duration, grain yield characteristics, and related parameters
The drought stress treatment produced significant changes in GFD, spikes pot –1, grains spike –1 , spike length, biomass pot –1 , thousand-grain weight, and grain yield pot –1 ( Table 2 ). However, drought priming lessened the loss caused by post-anthesis drought stress. Low N aggravated the effects of drought stress on these attributes. The level of grain yield reduction increased with increasing drought and N insufficiency. Drought-primed plants indicated less reduction in grain yield when compared to the non-primed plants in both cultivars under normal water conditions. Similarly, the grain yield reduced less under N2, which resulted in a more prominent drought index (DI) for drought stress treatments. Considerably higher DI was noted in N2 (PJWD) and lower DI was seen in N1 (WD). The impacts of drought stress treatments and N amount on grain yield were assigned to their remarkably similar effects on spikes pot –1 , grains spike –1 , spike length, biomass pot –1 , and thousand-grain weight under N1 drought stress conditions. The water level and N showed significant effects on the spikes pot –1 . N2 showed improved results compared to N1. Under WW, the number of grains spike –1 was better than WD and PJWD, whereas the number of grains spike –1 increased with an increasing level of nitrogen. Spike length significantly decreased as a response to PJWD and WD, and nitrogen content also affected the spike length. The thousand-grain weight was also influenced by drought stress and nitrogen level. However, the response to nitrogen was different than the water level and increased the thousand-grain weight. Furthermore, biomass at maturity decreased significantly due to post-anthesis drought stress and priming when compared to WW treatment, and its reduction was also greater in N1 compared to N2. Therefore, it can be said that a high dose of nitrogen N2 and priming before anthesis can reduce the impact of post-anthesis drought stress and increase drought resistance in plants.
Effects of pre-drought priming and nitrogen on grain yield and its components under post-anthesis drought stress in Yangmai-158 (YM-158) and Yangmai-22 (YM-22) wheat cultivars.
ns, non-significant and “*”, “**”, significant difference at 0.05 and 0.01 levels of probability, respectively. The different lowercase letters within the table showed significant differences at p-value 0.05. N, Nitrogen; WL, water level; V, variety.
The ability of the wheat plant to counteract drought stress in the post-anthesis stage varied with the variation in its treatments with priming and N level ( Abid et al., 2016a ). The results of the present study showed that drought stress reduced plant growth, while higher N application and pre-anthesis moderate drought stress (termed as priming) eased the negative effects of drought stress. In addition, under drought stress, stomatal conductance was decreased, which led to reduced photosynthesis when compared with other treatments. LRWC is the main characteristic that shows plant water level, and when LRWC drops, it causes stomatal closure and affects the availability of CO 2 during photosynthesis under water deficit conditions ( Moshelion et al., 2015 ). In this study, higher Ψw, Ψs, and LRWC values were observed under higher N application and pre-anthesis drought priming in response to drought stress when compared with non-primed plants, indicating that priming and N application trigger leaf activity that enables plants to continue normal functioning under drought stress conditions and helps plants to recover quickly once the drought is over, as demonstrated by the re-watering of the primed plants. Wang et al. (2014a , b) observed higher LRWC and stomatal conductance in the primed plants than in non-primed plants under drought stress conditions in wheat. Similarly, higher N application increased the stomatal conductance and leaf characteristics relative to low N application in drought-stressed plants, suggesting that under low N conditions, the leaf water potential would be decreased, which limits cell growth. As previously mentioned, reduced Ψw under abiotic stresses is the major source of decreased accumulation of dry matter and grain yield ( Gepstein and Glick, 2013 ). Our result showed that under drought conditions, the osmotic potential and LRWC in primed plants in both varieties were higher than those in the non-primed plants. These findings confirmed that drought-primed plants could maintain good water status by better controlling the osmotic potential to cope with drought stress after flowering.
In this study, the decrease in Chl content under drought stress might be the reason for the decline of the photochemical activity of chloroplast, which ultimately reduces photosynthetic activity ( Moshelion et al., 2015 ). However, compared with the non-primed plants and N1 supplementation, the primed and N2-supplemented plants restored the photochemical activities to some extent. It is worth noting that the obvious effect of limited N supply on the droughted plants is the decrease of the Chl and Rubisco contents ( Abid et al., 2016a ). As we know from the previous research, the larger part of N is stored in enzymes, such as Rubisco (which is the main source of the N recycling), and is a necessary component of enzymes involved in the photosynthetic process ( Abid et al., 2016a ). Previously, similar effects of nitrogen limitation on Rubisco concentration and photosynthesis were pointed out in rose plants by Gonzalez-Real and Baille (2000) , as well as in rice and wheat plants ( Makino, 2011 ) . Under severe drought stress, the carboxylation efficiency of Rubisco was incredibly reduced, and Rubisco acts as an oxygenase rather than a carboxylase, where it can reduce the carbon assimilation of photosynthesis ( Makino, 2011 ). N is the main part of Chl and proteins, influencing the entire metabolism of plants under drought stress. Grassi and Magnani, (2005) found that adequate N content partially restored the photosynthetic biochemistry compared to limited N availability. Previous studies have reported that when the drought stress level intensifies, it reduces the leaf area, which subsequently affects photosynthetic pigmentation and reduces stomatal conductance and transpiration rate ( Ahmad et al., 2022 ). In this manner, supporting the plant’s water status and stomatal opening under high N is important to maintaining the conductance of leaf to CO 2 , photosynthetic responses, and electron transfer ( Liu et al., 2013 ).
MDA is the main product of lipid peroxidation, which reflects the degree of damage to the plant photosynthetic functions under adverse conditions ( Zeeshan et al., 2020 , 2021 ). Severe drought stress under low nitrogen application causes excessive accumulation of MDA. The excessive accumulation of MDA triggers early leaf senescence, causes degradation of chlorophyll and macromolecules, and increases cell membrane permeability, which eventually largely affect the development of grains ( Zeeshan et al., 2020 ). We reported higher MDA and ROS accumulation in response to N1-supplemented and droughted plants (DS) when compared with N2-supplemented and primed plants (PJWD) under the same conditions. The strict control of ROS and MDA production and accumulation is to plant defense mechanisms and is a necessary phenomenon under unfavorable conditions because the excessive accumulation of these cellular toxicants can cause cell death, thereby decreasing plant growth and development ( Bieker and Zentgraf, 2013 ).
In order to cope with oxidative damage and keep a balance between the production and scavenging of reactive oxygen species and restoration of metabolic homeostasis, plants have to evolve a well-established antioxidant enzyme system ( Najafi et al., 2021 ; Salam et al., 2022 ). A study has found a strong correlation between oxidative stress caused by drought and the activities of antioxidant enzymes ( Adrees et al., 2020 ). The antioxidative enzyme system of plants, mainly comprising SOD, POD, and CAT, has a prominent role in the mitigation of oxidative stress ( Szalai et al., 2009 ). Our study reported elevated activity of SOD, POD, and CAT in flag leaves in response to the PJWD and higher application of N (N2) over non-primed plants supplemented with limited N (N1). These results suggest that the observed increase in SOD and POD activities would compensate for the accumulation of ROS. In addition, the increase in CAT activity would lead to scavenging the H 2 O 2 , thereby reducing oxidative damage to the membrane. SOD is a key enzyme for the scavenging of reactive oxygen species and conversion of O 2 − to H 2 O 2 . Finally, CAT and POD subsequently scavenge the H 2 O 2 produced due to dismutation and convert it into H 2 O and O 2 using ascorbate in the process ( Khan et al., 2020 ). This study recommends that priming and high doses of N can increase the antioxidant capacity after flowering to reduce the damage caused due to oxidative stress, which can be advantageous in improving the physiological activity of plant leaves. Under higher nitrogen application, the higher ROS detoxification by antioxidant systems in drought-stressed plants may help in protecting photosynthesis. Wang et al. (2014a , b) found higher antioxidant activities and lower accumulation of ROS and MDA in pre-stress primed plants over the non-primed plants under droughted conditions in wheat at different growth stages. Furthermore, Saneoka et al. (2004) observed that N nutrition promotes the drought tolerance of bent grass by stopping cell membrane damage, reducing MDA accumulation, and improving osmoregulation in Agrostis palustris Huds.
Under the droughted condition, crops alter their phenophases: they trigger early anthesis and early maturity in order to avoid stressful conditions, and this alteration is considered vital for maintaining higher grain yield, particularly in cereal ( Chaves et al., 2002 ). This alteration could shift the post-anthesis stage for suitable conditions for leaf photosynthesis and grain filling in wheat ( Fan et al., 2017 ). In the current study, GFD was reduced under the WD condition relative to other treatments, which in turn decreased the grain weight. Previously, Lv et al. (2021) mentioned that in wheat crops, both grain filling rate (GFR) and GFD have a direct impact on grain weight. Similarly, Wu et al. (2011) observed that during the vegetative growth stage, the drought stress alone and/or limited N supply significantly reduced the grain weight and hindered the yield. In the current study, upon supplementation with high N treatment (N2), plants produced more grains and increased plant green canopy, resulting in elevated photosynthetic activity and a high carbon accumulation. On the other hand, droughted plants (WD) under low nitrogen supplementation produced fewer grains and a smaller canopy, resulting in decreased photosynthetic activity. According to an estimation, about 30–50% of the photosynthetic products required for wheat grain filling are delivered through the current photosynthesis during grain filling, so the “keep green” feature can extend the duration of its availability ( Inoue et al., 2004 ; Abid et al., 2016c ). In addition, the ability of the PJWD plants to maintain a higher photosynthetic rate and chlorophyll content may be the ultimate cause for the increase in grain filling rate and duration, and ultimately higher grain yield as compared to the WD plants. The grain filling rate and duration are the key factors that determine the final grain weight, and the final grain weight is crucial for final grain yield ( Altenbach, 2012 ). Therefore, compared with WD plants at post-anthesis, the PJWD plants maintained a higher sink strength, had satisfactory translocation, and received grain reserves, confirming the successful development of grains, and limiting their severe dry weight and grain yield decline.
Furthermore, the PJWD plants efficiently prevented water loss in grains under drought conditions, which might avoid the vascular physiochemical gap within the grain. As the endosperm and embryo dehydration could limit the metabolism of obtained assimilates, the hydraulic response of the developing grain to drought could have a direct impact on filling quality ( Egli and TeKrony, 1997 ; Gupta et al., 2011 ). Under unfavorable environmental conditions, particularly at the post-anthesis stage, plant physiological activities can be reduced and affect the grain yield. Therefore, to face the unfavorable conditions and reduce yield losses, the application of N and priming are needed before post-anthesis drought stress. A higher application of N (N2) along with PJWD plants produced notably higher grain yield and yield-related parameters when compared to remaining treatments except WW. Taken together, the tandem application of higher N with pre-anthesis drought priming can reduce the drought stress on the wheat plants at the post-anthesis stage and can improve the yield of wheat in response to drought stress.
The results of this study indicated that wheat plants pre-exposed to moderate drought stress (priming) and an adequate supply of nitrogen have reduced adverse effects of drought stress after anthesis. Nitrogen and priming had significant effects on wheat’s physiological activities and grain yield. The study found that high nitrogen and priming before anthesis induced greater photosynthetic capacity by expanding the green flag leaf area, elevating the chlorophyll and soluble protein content, increasing sink strength, and increasing the GFD, thereby increasing the grain weight and yield. Furthermore, high nitrogen and priming also increased antioxidant capacity and reduced oxidative stress and MDA accumulation. Drought priming in the early growth stages (especially during the jointing period) highlighted the importance of drought timing and was confirmed to be the best approach to trigger the plants to initiate an effective tolerance mechanism against drought stress. Agriculture will face severe water shortage in the future, and hence to face the challenge of water scarcity, we should not only rely on breeding new cultivars that can tolerate water scarcity, but also invent crop management techniques that can increase crop potential to achieve sustainable crop production.
Data availability statement
Author contributions.
AU, ZT, and TD conceived and organized the experiment. AU conducted the experiment, collected the samples, analyzed the data, and wrote the draft. LX, KL, CS, AK, and YJ helped in plant sampling and data analysis. MA and MZ critically revised the manuscript. TD acquired the funding. All authors contributed to the article and approved the submitted version.
Acknowledgments
We appreciate and acknowledge the effort of K. G. Daniels from Cell Design Institute, Department of Cellular and Molecular Pharmacology, University of California, San Francisco, CA 94158, United States, for revising and proofreading this manuscript.
This work was supported by the National Key R&D Program of China (grant no. 2017YFD0301204) and the National Natural Science Foundation of China (grant nos. 31471443 and 31501262). We also acknowledge the support from the Jiangsu Collaborative Innovation Center for Modern Crop Production (JCIC-MCP).
Conflict of interest
The authors declare that the research was conducted in the absence of any commercial or financial relationships that could be construed as a potential conflict of interest.
Publisher’s note
All claims expressed in this article are solely those of the authors and do not necessarily represent those of their affiliated organizations, or those of the publisher, the editors and the reviewers. Any product that may be evaluated in this article, or claim that may be made by its manufacturer, is not guaranteed or endorsed by the publisher.
- Abid M., Tian Z., Ata-Ul-Karim S. T., Liu Y., Cui Y., Zahoor R., et al. (2016b). Improved tolerance to post-anthesis drought stress by pre-drought priming at vegetative stages in drought-tolerant and-sensitive wheat cultivars. Plant Physiol. Biochem. 106 218–227. 10.1016/j.plaphy.2016.05.003 [ PubMed ] [ CrossRef ] [ Google Scholar ]
- Abid M., Tian Z., Ata-Ul-Karim S. T., Cui Y., Liu Y., Zahoor R., et al. (2016a). Nitrogen nutrition improves the potential of wheat ( Triticum aestivum L.) to alleviate the effects of drought stress during vegetative growth periods. Front. Plant Sci. 7 : 981 . 10.3389/fpls.2016.00981 [ PMC free article ] [ PubMed ] [ CrossRef ] [ Google Scholar ]
- Abid M., Tian Z., Ata-Ul-Karim S. T., Wang F., Liu Y., Zahoor R., et al. (2016c). Adaptation to and recovery from drought stress at vegetative stages in wheat ( Triticum aestivum ) cultivars. Funct. Plant Biol 43 1159–1169. 10.1071/FP16150 [ PubMed ] [ CrossRef ] [ Google Scholar ]
- Adrees M., Khan Z. S., Ali S., Hafeez M., Khalid S., ur Rehman M. Z., et al. (2020). Simultaneous mitigation of cadmium and drought stress in wheat by soil application of iron nanoparticles. Chemosphere 238 : 124681 . 10.1016/j.chemosphere.2019.124681 [ PubMed ] [ CrossRef ] [ Google Scholar ]
- Ahmad A., Aslam Z., Naz M., Hussain S., Javed T., Aslam S., et al. (2021). Exogenous salicylic acid-induced drought stress tolerance in wheat ( Triticum aestivum L.) grown under hydroponic culture. PLoS One 16 : e0260556 . 10.1371/journal.pone.0260556 [ PMC free article ] [ PubMed ] [ CrossRef ] [ Google Scholar ] Retracted
- Ahmad S., Kamran M., Ding R., Meng X., Wang H., Ahmad I., et al. (2019). Exogenous melatonin confers drought stress by promoting plant growth, photosynthetic capacity and antioxidant defense system of maize seedlings. PeerJ 7 : e7793 . 10.7717/peerj.7793 [ PMC free article ] [ PubMed ] [ CrossRef ] [ Google Scholar ]
- Ahmad S., Muhammad I., Wang G. Y., Zeeshan M., Yang L., Ali I., et al. (2021). Ameliorative effect of melatonin improves drought tolerance by regulating growth, photosynthetic traits and leaf ultrastructure of maize seedlings. BMC Plant Biol 21 : 368 . 10.1186/s12870-021-03160-w [ PMC free article ] [ PubMed ] [ CrossRef ] [ Google Scholar ]
- Ahmad S., Wang G. Y., Muhammad I., Chi Y. X., Zeeshan M., Nasar J., et al. (2022). Interactive effects of melatonin and nitrogen improve drought tolerance of maize seedlings by regulating growth and physiochemical attributes. Antioxidants 11 : 359 . 10.3390/antiox11020359 [ PMC free article ] [ PubMed ] [ CrossRef ] [ Google Scholar ]
- Altenbach S. B. (2012). New insights into the effects of high temperature, drought and post-anthesis fertilizer on wheat grain development. J. Cereal Sci. 56 39–50. 10.1016/j.jcs.2011.12.012 [ CrossRef ] [ Google Scholar ]
- Ata-Ul-Karim S. T., Liu X., Lu Z., Yuan Z., Zhu Y., Cao W. (2016). In-season estimation of rice grain yield using critical nitrogen dilution curve. Field Crops Res. 195 1–8. 10.1016/j.fcr.2016.04.027 [ CrossRef ] [ Google Scholar ]
- Batra N. G., Sharma V., Kumari N. (2014). Drought-induced changes in chlorophyll fluorescence, photosynthetic pigments, and thylakoid membrane proteins of Vigna radiata . J. Plant Interact 9 712–721. [ Google Scholar ]
- Barrs H. D., Weatherley P. E. (1962). A re-examination of the relative turgidity technique for estimating water deficits in leaves. Aus. J. Biol. Sci 15 413–428. 10.1071/BI9620413 [ CrossRef ] [ Google Scholar ]
- Bieker S., Zentgraf U. (2013). Plant senescence and nitrogen mobilization and signaling. Senescence Senescence Relat. Disord. 104 53–83. 10.5772/54392 [ CrossRef ] [ Google Scholar ]
- Chaves M. M., Pereira J. S., Maroco J., Rodrigues M. L., Ricardo C. P., Osório M. L., et al. (2002). How plants cope with water stress in the field? Photosynthesis and growth. Ann. Bot. 89 907–916. 10.1093/aob/mcf105 [ PMC free article ] [ PubMed ] [ CrossRef ] [ Google Scholar ]
- Cossani C. M., Reynolds M. P. (2012). Physiological traits for improving heat tolerance in wheat. Plant Physiol. 160 1710–1718. 10.1104/pp.112.207753 [ PMC free article ] [ PubMed ] [ CrossRef ] [ Google Scholar ]
- Dhanda S. S., Sethi G. S. (2002). Tolerance to drought stress among selected Indian wheat cultivars. J. Agri. Sci. 139 319–326. 10.1017/S0021859602002526 [ CrossRef ] [ Google Scholar ]
- Egli D. B., TeKrony D. M. (1997). Species differences in seed water status during seed maturation and germination. Seed Sci. Res. 7 3–12. 10.1017/S0960258500003305 [ CrossRef ] [ Google Scholar ]
- Ernst L., Goodger J. Q., Alvarez S., Marsh E. L., Berla B., Lockhart E., et al. (2010). Sulphate as a xylem-borne chemical signal precedes the expression of ABA biosynthetic genes in maize roots. J. Exp. Bot. 61 3395–3405. 10.1093/jxb/erq160 [ PubMed ] [ CrossRef ] [ Google Scholar ]
- Fan Y., Tian Z., Yan Y., Hu C., Abid M., Jiang D., et al. (2017). Winter night-warming improves post-anthesis physiological activities and sink strength in relation to grain filling in winter wheat ( Triticum aestivum L.). Front. Plant Sci. 8 : 992 . 10.3389/fpls.2017.00992 [ PMC free article ] [ PubMed ] [ CrossRef ] [ Google Scholar ]
- Gaikwad P. N., Sidhu G. S., Gahukar S. J., Kharade S. J., Chavan R. S., Bhojane P. N. A. (2020). Review: advances in draught stress tolerance in wheat ( Triticum aestivum L.). Int. J. Curr. Microbiol. App. Sci. 9 2873–2884. 10.20546/ijcmas.2020.905.330 [ CrossRef ] [ Google Scholar ]
- Gepstein S., Glick B.R. (2013) Strategies to ameliorate abiotic stress-induced plant senescence. Plant Molecul. Biol. 82 623–633. 10.1007/s11103-013-0038-z [ PubMed ] [ CrossRef ] [ Google Scholar ]
- Grassi G., Magnani F. (2005). Stomatal, mesophyll conductance and biochemical limitations to photosynthesis as affected by drought and leaf ontogeny in ash and oak trees. Plant Cell Environ. 28 834–849. [ Google Scholar ]
- Gonzalez-Real M. M., Baille A. (2000). Changes in leaf photosynthetic parameters with leaf position and nitrogen content within a rose plant canopy (Rosa hybrida). Plant Cell Environ. 23 351–363. [ Google Scholar ]
- Gupta A. K., Kaur K., Kaur N. (2011). Stem reserve mobilization and sink activity in wheat under drought conditions. Amer. J. Plant Sci. 2 : 70 . 10.4236/ajps.2011.21010 [ CrossRef ] [ Google Scholar ]
- Inoue T., Inanaga S., Sugimoto Y., El Siddig K. (2004). Contribution of pre-anthesis assimilates and current photosynthesis to grain yield, and their relationships to drought resistance in wheat cultivars grown under different soil moisture. Photosynthetica 42 99–104. [ Google Scholar ]
- Jabborova D., Annapurna K., Kakhramon D., Abdujalil N., Yuriy E., Asad S., et al. (2021). Co inoculation of rhizobacteria promotes growth, yield and nutrient contents in soybean and improves soil enzymes and nutrients under drought conditions. Sci. Rep. 11 : 22081 . 10.1038/s41598-021-01337-9 [ PMC free article ] [ PubMed ] [ CrossRef ] [ Google Scholar ]
- Khan I., Samrah A. A., Rizwana I., Muhammad R., Nosheen A., Humaira Y., et al. (2020). Effect of 24-Epibrassinolide on plant growth, antioxidants defense system and endogenous hormones in two wheat varieties under drought stress. Physiol. Planta 172 696–706. 10.1111/ppl.13237 [ PubMed ] [ CrossRef ] [ Google Scholar ]
- Li C., Jiang D., Wollenweber B., Li Y., Dai T., Cao W. (2011). Waterlogging pretreatment during vegetative growth improves tolerance to waterlogging after anthesis in wheat. Plant Sci. 180 672–678. 10.1016/j.plantsci.2011.01.009 [ PubMed ] [ CrossRef ] [ Google Scholar ]
- Li X., Pu H., Liu F., Zhou Q., Cai J., Dai T., et al. (2015). Winter wheat photosynthesis and grain yield responses to spring freeze. Agronom. J. 107 1002–1010. 10.2134/agronj14.0460 [ CrossRef ] [ Google Scholar ]
- Liu H., Sultanm M. A., Liu X. L., Zhang J., Yu F., Zhao H. X. (2015). Physiological and comparative proteomic analysis reveals different drought responses in roots and leaves of drought-tolerant wild wheat ( Triticum boeoticum ). PLoS One 10 : e0121852 . 10.1371/journal.pone.0121852 [ PMC free article ] [ PubMed ] [ CrossRef ] [ Google Scholar ]
- Liu X., Fan Y., Long J., Wei R., Kjelgren R., Gong C., et al. (2013). Effects of soil water and nitrogen availability on photosynthesis and water use efficiency of Robinia pseudoacacia seedlings. J. Environ. Sci. 25 585–595. 10.1016/S1001-0742(12)60081-3 [ PubMed ] [ CrossRef ] [ Google Scholar ]
- Lv X., Ding Y., Long M., Liang W., Gu X., Liu Y., et al. (2021). Effect of foliar application of various nitrogen forms on starch accumulation and grain filling of wheat ( Triticum aestivum L.) under drought stress. Front. Plant Sci. 12 : 463 . 10.3389/fpls.2021.645379 [ PMC free article ] [ PubMed ] [ CrossRef ] [ Google Scholar ]
- Madani A., Rad A. S., Pazoki A., Nourmohammadi G., Zarghami R. (2010). Wheat ( Triticum aestivum L.) grain filling and dry matter partitioning responses to source: sink modifications under postanthesis water and nitrogen deficiency. Acta Sci. Agron. 32 145–151. [ Google Scholar ]
- Makino A. (2011). Photosynthesis, grain yield, and nitrogen utilization in rice and wheat. Plant Physiol. 155 125–129. 10.1104/pp.110.165076 [ PMC free article ] [ PubMed ] [ CrossRef ] [ Google Scholar ]
- Maxwell K., Johnson G. N. (2000). Chlorophyll fluorescence—a practical guide. J. Exp. Bot. 51 659–668. 10.1093/jexbot/51.345.659 [ PubMed ] [ CrossRef ] [ Google Scholar ]
- Mobasser H. R., Mohammadi G. N., Abad H. H., Rigi K. (2014). Effect of application elements, water stress and variety on nutrients of grain wheat in Zahak region. Iran. JBES. 5 105–110. [ Google Scholar ]
- Morgan J. A. (1986). The effects of N nutrition on the water relations and gas exchange characteristics of wheat (Triticum aestivum L.). Plant Physiol. 80 52–58. 10.1104/pp.80.1.52 [ PMC free article ] [ PubMed ] [ CrossRef ] [ Google Scholar ]
- Moshelion M., Halperin O., Wallach R., Oren R. A., Way D. A. (2015). Role of aquaporins in determining transpiration and photosynthesis in water-stressed plants: crop water-use efficiency, growth and yield. Plant Cell Environ. 38 1785–1793. 10.1111/pce.12410 [ PubMed ] [ CrossRef ] [ Google Scholar ]
- Murchie E. H., Yang J., Hubbart S., Horton P., Peng S. (2002). Are there associations between grain-filling rate and photosynthesis in the flag leaves of field-grown rice? Environ. Exp. Bot. 53 2217–2224. 10.1093/jxb/erf064 [ PubMed ] [ CrossRef ] [ Google Scholar ]
- Najafi S., Nazari Nasi H., Tuncturk R., Tuncturk M., Sayyed R. Z., Amirnia R. (2021). Bio fertilizer application enhances drought stress tolerance and alters the antioxidant Enzymes in medicinal pumpkin ( Cucurbita pepo convar. pepo var. Styriaca ). Horticulturae 7 : 588 . [ Google Scholar ]
- Nardino M., Perin E. C., Aranha B. C., Carpes S. T., Fontoura B. H., de Sousa D. J. P., et al. (2022). Understanding drought response mechanisms in wheat and multi-trait selection. PloS one 17 : e0266368 . 10.1371/journal.pone.0266368 [ PMC free article ] [ PubMed ] [ CrossRef ] [ Google Scholar ]
- Niu Y., Wang Y., Li P., Zhang F., Liu H., Zheng G. (2013). Drought stress induces oxidative stress and the antioxidant defense system in ascorbate-deficient vtc1 mutants of Arabidopsis thaliana . Acta Physiol. Planta. 35 1189–1200. 10.1007/s11738-012-1158-9 [ CrossRef ] [ Google Scholar ]
- Ribaut J. M., Pilet P. E. (1991). Effects of water stress on growth, osmotic potential and abscisic acid content of maize roots. Physiol. Planta. 81 156–162. [ Google Scholar ]
- Salam A., Khan A. R., Liu L., Yang S., Azhar W., Ulhassan Z., et al. (2022). Seed priming with zinc oxide nanoparticles downplayed ultrastructural damage and improved photosynthetic apparatus in maize under cobalt stress. J. Hazard. Mat. 423 : 127021 . 10.1016/j.jhazmat.2021.127021 [ PubMed ] [ CrossRef ] [ Google Scholar ]
- Saneoka H., Moghaieb R. E., Premachandra G. S., Fujita K. (2004). Nitrogen nutrition and water stress effects on cell membrane stability and leaf water relations in Agrostis palustris Huds. Environ. Exp. Bot. 52 131–138. 10.1016/j.envexpbot.2004.01.011 [ CrossRef ] [ Google Scholar ]
- Shabbir R. N., Waraich E. A., Ali H., Nawaz F., Ashraf M. Y., Ahmad R., et al. (2016). Supplemental exogenous NPK application alters biochemical processes to improve yield and drought tolerance in wheat ( Triticum aestivum L.). Environ. Sci. Pollut. Res. 23 2651–2662. 10.1007/s11356-015-5452-0 [ PubMed ] [ CrossRef ] [ Google Scholar ]
- Shi J., Yasuor H., Yermiyahu U., Zuo Q., Ben-Gal A. (2014). Dynamic responses of wheat to drought and nitrogen stresses during re-watering cycles. Agri. Water Manage. 146 163–172. 10.1016/j.agwat.2014.08.006 [ CrossRef ] [ Google Scholar ]
- Siddiqui M. H., Mutahhar Y. Al-K, Al-Q, Mohammed A., Al-W, Mohamed H., Anil G., Hayssam M. A., et al. (2015). Response of different genotypes of faba bean plant to drought stress. Int. J. Mol. Sci. 16 10214–10227. 10.3390/ijms160510214 [ PMC free article ] [ PubMed ] [ CrossRef ] [ Google Scholar ]
- Siddiqui M. H., Nasir K. M., Soumya M., Saud A., Riyadh A. B., Abdullah A., et al. (2021). Hydrogen sulfide (H2S) and potassium (K+) synergistically induce drought stress tolerance through regulation of H+ ATPase activity, sugar metabolism, and antioxidative defense in tomato seedlings. Plant Cell Rep. 40 1543–1564. 10.1007/s00299-021-02731-3 [ PubMed ] [ CrossRef ] [ Google Scholar ]
- Sui N., Li M., Liu X. Y., Wang N., Fang W., Meng Q. W. (2007). Response of xanthophyll cycle and chloroplastic antioxidant enzymes to chilling stress in tomato over-expressing glycerol-3-phosphate acyltransferase gene. Photosynthetica 45 447–454. 10.1007/s11099-007-0074-5 [ CrossRef ] [ Google Scholar ]
- Szalai G., Kellõs T., Galiba G., Kocsy G. (2009). Glutathione as an antioxidant and regulatory molecule in plants under abiotic stress conditions. J. Plant Growth Regul. 28 66–80. [ Google Scholar ]
- Tan W., Liu J., Dai T., Jing Q., Cao W., Jiang D. (2008). Alterations in photosynthesis and antioxidant enzyme activity in winter wheat subjected to post-anthesis water-logging. Photosynthetica 46 21–27. 10.1007/s11099-008-0005-0 [ CrossRef ] [ Google Scholar ]
- Teixeira E. I., George M., Herreman T., Brown H., Fletcher A., Chakwizira E., et al. (2014). The impact of water and nitrogen limitation on maize biomass and resource-use efficiencies for radiation, water and nitrogen. Field Crops Res. 168 : 109 . 10.1016/j.fcr.2014.08.002 [ CrossRef ] [ Google Scholar ]
- Thakur P., Kumar S., Malik J. A., Berger J. D., Nayyar H. (2010). Cold stress effects on reproductive development in grain crops: an overview. Environ. Exp. Bot. 67 429–443. 10.1016/j.envexpbot.2009.09.004 [ CrossRef ] [ Google Scholar ]
- Wang G. Y., Hu Y. X., Liu Y. X., Ahmad S., Zhou X. B. (2021). Effects of supplement irrigation and nitrogen application levels on soil carbon–nitrogen content and yield of one-year double cropping maize in subtropical region. Water 13 : 1180 . 10.3390/w13091180 [ CrossRef ] [ Google Scholar ]
- Wang X., Vignjevic M., Liu F., Jacobsen S., Jiang D., Wollenweber B. (2014a). Drought priming at vegetative growth stages improves tolerance to drought and heat stresses occurring during grain filling in spring wheat. Plant Growth Regul. 75 677–687. 10.1007/s10725-014-9969-x [ CrossRef ] [ Google Scholar ]
- Wang X., Vignjevic M., Jiang D., Jacobsen S., Wollenweber B. (2014b). Improved tolerance to drought stress after anthesis due to priming before anthesis in wheat ( Triticum aestivum L.) var. Vinjett. J. Exp. Bot. 65 6441–6456. 10.1093/jxb/eru362 [ PMC free article ] [ PubMed ] [ CrossRef ] [ Google Scholar ]
- Wang X., Zhang X., Chen J., Wang X., Cai J., Zhou Q., et al. (2018). Parental drought-priming enhances tolerance to post-anthesis drought in offspring of wheat. Front. Plant Sci. 9 : 261 . 10.3389/fpls.2018.00261 [ PMC free article ] [ PubMed ] [ CrossRef ] [ Google Scholar ]
- Wu Y., Liu W., Li X., Li M., Zhang D., Hao Z., et al. (2011). Low-nitrogen stress tolerance and nitrogen agronomic efficiency among maize inbreds: comparison of multiple indices and evaluation of genetic variation. Euphytica 180 281–290. 10.1007/s10681-011-0409-y [ CrossRef ] [ Google Scholar ]
- Wu Y., Wang Y., Shi H., Hu H., Yi L., Hou J. (2022). Time-course transcriptome and WGCNA analysis revealed the drought response mechanism of two sunflower inbred lines. PLoS One 17 : e0265447 . 10.1371/journal.pone.0265447 [ PMC free article ] [ PubMed ] [ CrossRef ] [ Google Scholar ]
- Yang S. H., Wang L. J., Li S. H. (2007). Ultraviolet-B irradiation-induced freezing tolerance in relation to antioxidant system in winter wheat ( Triticum aestivum L.) leaves. Environ. Exp. Bot. 60 300–307. 10.1016/j.envexpbot.2006.12.003 [ CrossRef ] [ Google Scholar ]
- Yang Y., Guo J., Wang G., Yang L., Yang Y. (2012). Effects of drought and nitrogen addition on photosynthetic characteristics and resource allocation of Abies fabri seedlings in eastern Tibetan Plateau. New For. 43 505–518. 10.1007/s11056-011-9295-3 [ CrossRef ] [ Google Scholar ]
- Zeeshan M., Hu Y. X., Iqbal A., Salam A., Liu Y. X., Muhammad I., et al. (2021). Amelioration of AsV toxicity by concurrent application of ZnO-NPs and Se-NPs is associated with differential regulation of photosynthetic indexes, antioxidant pool and osmolytes content in soybean seedling. Ecotoxicol. Environ. Saf. 225 : 112738 . 10.1016/j.ecoenv.2021.112738 [ PubMed ] [ CrossRef ] [ Google Scholar ]
- Zeeshan M., Lu M., Sehar S., Holford P., Wu F. (2020). Comparison of biochemical, anatomical, morphological, and physiological responses to salinity stress in wheat and barley genotypes deferring in salinity tolerance. Agronomy 10 : 127 . 10.3390/agronomy10010127 [ CrossRef ] [ Google Scholar ]
- Zhang L. X., Li S. X., Zhang H., Liang Z. S. (2007). Nitrogen rates and water stress effects on production, lipid peroxidation and antioxidative enzyme activities in two maize (Zea mays L.) genotypes. J. Agronom. Crop Sci 193 387–397. [ Google Scholar ]
- Zhang X., Wang X., Zhong J., Zhou Q., Wang X., Cai J. (2016). Drought priming induces thermo-tolerance to post-anthesis high-temperature in offspring of winter wheat. Environ. Exp. Bot. 127 26–36. 10.3389/fpls.2016.00501 [ PMC free article ] [ PubMed ] [ CrossRef ] [ Google Scholar ]
- Zheng C., Jiang D., Liu F., Dai T., Jing Q., Cao W. (2009). Effects of salt and waterlogging stresses and their combination on leaf photosynthesis, chloroplast ATP synthesis, and antioxidant capacity in wheat. Plant Sci. 176 575–582. 10.1016/j.plantsci.2009.01.015 [ PubMed ] [ CrossRef ] [ Google Scholar ]
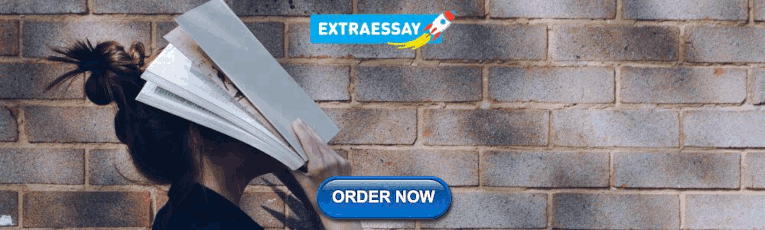
IMAGES
VIDEO
COMMENTS
anthesis: [noun] the action or period of opening of a flower.
Post-Anesthesia Care: Symptoms, Diagnosis, and Management provides a novel and common sense approach to the challenges faced by PACU clinicians. By focusing on the initial presenting symptoms, the book facilitates the development of a differential diagnosis and an appropriate plan of action. Section I covers the challenges of the postsurgical ...
Postanthesis definition: Occurring after the opening of a flower. .
Anthesis is the period during which a flower is fully open and functional. It may also refer to the onset of that period. The onset of anthesis is spectacular in some species. In Banksia species, for example, anthesis involves the extension of the style far beyond the upper perianth parts.
The term anthesis is derived from the Greek word anthos, meaning flower, and has been used in botanical literature to describe the flowering stage since at least the 18th century. The study of anthesis and its patterns has been important in the field of botany for understanding plant reproduction and the evolution of flowering plants.
Post-anthesis nitrogen uptake and translocation play critical roles in photosynthetic assimilation and grain filling. However, their effects on leaf stay-green characteristics, dry matter ...
Last days of anthesis: C. carduacea Anthesis was noted to last 5 days with C. carduacea. In the series for day 4-5 (anthesis) plus day 6 (post-anthesis; rightmost 2 images) the pappus lengthens until it completely envelops the style branches. The head shown is the same as the one above for the onset of anthesis.
postanthesis (not comparable) occurring after the opening of a flower.
Examples of how to use "anthesis" in a sentence from Cambridge Dictionary.
Botany. 1783-. The stage at which a flower is open, allowing fertilization to occur. Also: an instance of this. 1783. The Anthesis [Latin Anthesis] takes place, when the burnt Anthers scatter their bags of Dust upon the Stigma. translation of C. Linnaeus, Syst. Veg. (1785) vol. I. 10.
Anthesis definition: the period or act of expansion in flowers, especially the maturing of the stamens. See examples of ANTHESIS used in a sentence.
The highest post-anthesis N uptake was recorded in P × Wat (24 kg ha −1), which contributed 31% towards the total N uptake in grains. The uptake of pre- and post-anthesis N was lowest in P × Wya as compared to other groups. Both pre- or post-anthesis N uptake showed positive corelations with grain yield (Fig. 5).
In cereals, photosynthesis of the flag leaf and inflorescence during post-anthesis plays a crucial role in grain development (Murchie et al., 2002). Therefore, improving drought tolerance during post-anthesis in wheat is crucial for sustaining food security in the present global climate scenario.
The climate is cold, humid Mediterranean with mean annual maximum and minimum daily air temperatures of 20.2 and 9.5 ... Post-anthesis dry matter, N and P accumulation as the difference between dry matter, N or P content of the whole plant at anthesis and at physiological maturity. 2.
Post-anthesis dry matter accumulation under timely sown accounted for 62.5% (av. of 2 yrs.) of the grain yield, wherein it was 55.8% greater than the late sown crop. ... The higher rate of mean productivity (MP) and geometric mean productivity (GMP) ...
Post-anthesis nitrogen uptake and translocation play critical roles in photosynthetic assimilation and grain filling. However, their effects on leaf stay-green characteristics, dry matter accumulation, and translocation after anthesis remain unclear. ... It has been reported that a longer period of grain filling, which leads to higher ...
Moreover, greater N accumulation post-anthesis has a positive correlation with grain yield in rice, indicating that the post-anthesis N-accumulation plays an essential role in expanding the grain ...
Mean values of agronomic traits and canopy temperature among 15 wheat genotypes evaluated under non-stressed (NS), pre-anthesis (PrADS) and post-anthesis (PoADS) drought stress conditions in the field environment. ... In the present study, pre- and post-anthesis drought stress had a negligible effect on flowering and maturation times across the ...
Post-anthesis dry matter accumulation (PDMA) plays an important role in yield, but the differences and mechanisms of PDMA among rice varieties with different yield levels are still unclear. ... To ensure representativeness of the sampling, plants of nine hills with the mean stem number from each plot were sampled for measurements of stem and ...
The GNC was quite unaltered by pre- and post-anthesis N treatments (Fig. 3a), as it was statistically affected only by the post-anthesis LN treatment (−27%). In combination with the reduction of ...
Days after anthesis (DAA) and postharvest behavior were used as a novel integrative holistic approach to define the physiological and horticultural maturity of fruit and implement appropriate harvesting times for quality and nutraceutical content. ... The samples were analyzed in three dilutions taking as mean the final ORAC value as ...
The post-anthesis temperature of the two types of wheat showed the same rule under different sowing dates, and the rules were similar in different years. ( Table 1 and Table 2 ) With the postponement of the sowing date, the EAT of S1 was lower than that of S2-S6 by −1.9-57.1 °C d, 14.4-72.9 °C d, 32.6-90.7 °C d, 68.4-107.3 °C d ...
Experimental design. To assess the combined effects of nitrogen and pre-drought priming on the wheat plants during post-anthesis drought stress, a rain cover pot experiment was conducted at the Pailou Experimental Research Station of Nanjing Agricultural University, China (32°040 N, 118°760 E), during the growing period of 2018-2019.