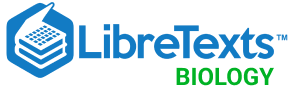
- school Campus Bookshelves
- menu_book Bookshelves
- perm_media Learning Objects
- login Login
- how_to_reg Request Instructor Account
- hub Instructor Commons
- Download Page (PDF)
- Download Full Book (PDF)
- Periodic Table
- Physics Constants
- Scientific Calculator
- Reference & Cite
- Tools expand_more
- Readability
selected template will load here
This action is not available.
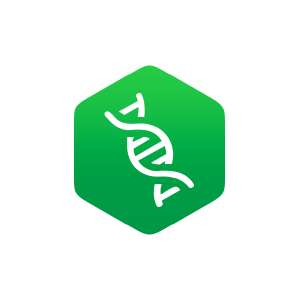
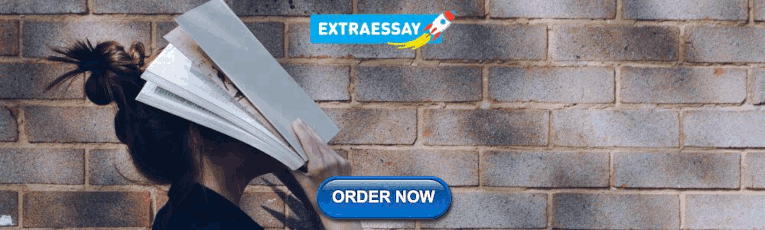
3.1: Discovery of Photosynthesis
- Last updated
- Save as PDF
- Page ID 17983
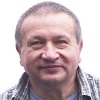
- Alexey Shipunov
- Minot State University
The history of the studies done on photosynthesis dates back into the 17th century with Jan Baptist van Helmont. He rejected the ancient idea that plants take most of their biomass from the soil. For the proof, he performed willow tree experiment. He started with a willow tree of 2.27 kg. Over 5 years, it grew to 67.7 kg. However, the weight of the soil only decreased by 57 grams. Van Helmont came to the conclusion that plants must take most of their weight from water. He did not know about gases.
Joseph Priestley ran a series of experiments in 1772 (Figure \(\PageIndex{1}\)). He tested a mouse, a candle, and a sprig of mint under hermetically sealed (no air can go in or out) jar. He first observed that a mouse and a candle behave very similarly when covered, in that they both “spend” the air. However, when a plant is placed with either the candle or mouse, the plant “revives” the air for both.
Further ideas were brought about in the late 1700’s. Jan Ingenhousz and Jean Senebier found that the air is only reviving in the day time and that CO\(_2\) is assembled by plants. Antoin-Laurent Lavoiser found that “revived air” is a separate gas, oxygen.
But what is the oxygen “maker”? There are many pigments in plants, and all accept and reflect some parts of rainbow. To identify the culprit, Thomas Engelmann ran an experiment (Figure \(\PageIndex{2}\)) using a crystal prism. He found that Spirogyra algae produce oxygen mostly in the blue and red parts of the spectrum. This was a huge find. It tells that the key photosynthetic pigment should accept blue and red rays, and thus reflect green rays. Blue-green chlorophyll best fits this description.
Another important fact was discovered by Frederick Blackman in 1905. He found that if light intensity is low, the increase of temperature actually has very little effect on the rate of photosynthesis (Figure \(\PageIndex{3}\)). However, the reverse is not exactly true, and light is able to intensify
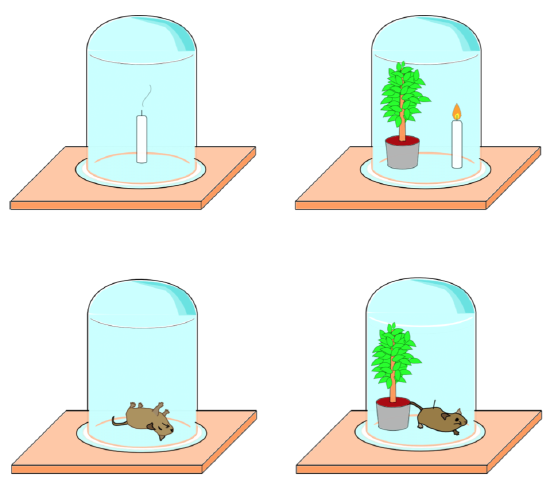
photosynthesis even when it is cold.
This could not happen if light and temperature are absolutely independent factors. If temperature and light are components of the chain, light was first (“ignition”) and temperature was second. This ultimately shows that photosynthesis has two stages . The first is a light stage . This stage relates to the intensity of the light. The second stage is the enzymatic (light-independent) stage which relates more with the temperature. Light reactions depend on the amount of light and water; they produce oxygen and energy in the form of ATP. Enzymatic reactions depend on carbon dioxide and water; they take energy from the light reactions and produce carbohydrates. Sometimes, enzymatic stage is called “dark” but it is not correct because in darkness, plant will run out of light-stage ATP almost immediately. Only some C\(_4\)-related processes (see below) could run at night.
Since water molecules are spent on light stage to make oxygen and at the same time are accumulating (see below), one of the best “equations” describing photosynthesis as a whole is \[{CO}_2 + {H}_2{O} + {light} \rightarrow {carbohydrates} + H_2O + O_2\]
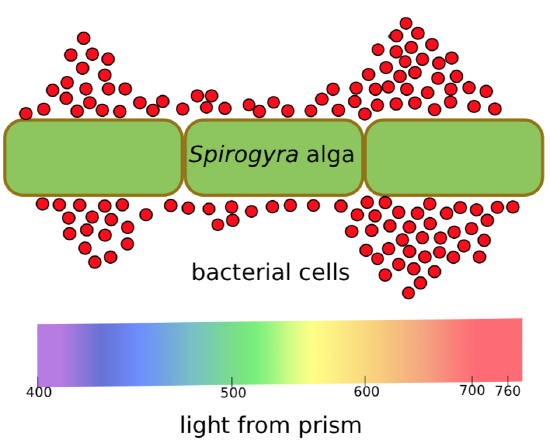
- Search Menu
- Advance Articles
- Collections
- Focus Collections
- Browse by cover
- High-Impact Research
- Author Guidelines
- Quick and Simple Author Support
- Focus Issues Call for Papers
- Submission Site
- Open Access Options
- Self-Archiving Policy
- Why Publish with Us?
- About Plant Physiology
- Editorial Board
- Advertising & Corporate Services
- Journals on Oxford Academic
- Books on Oxford Academic
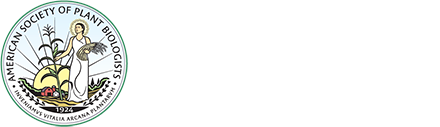
Article Contents
Origins of photosynthesis, photosynthetic pigments, reaction centers, electron transport chains, antenna systems, carbon fixation pathways, transition to oxygenic photosynthesis, literature cited.
- < Previous
Early Evolution of Photosynthesis
This work was supported by the Exobiology Program from the U.S. National Aeronautics and Space Administration (grant no. NNX08AP62G).
E-mail [email protected] .
www.plantphysiol.org/cgi/doi/10.1104/pp.110.161687
- Article contents
- Figures & tables
- Supplementary Data
Robert E. Blankenship, Early Evolution of Photosynthesis, Plant Physiology , Volume 154, Issue 2, October 2010, Pages 434–438, https://doi.org/10.1104/pp.110.161687
- Permissions Icon Permissions
Photosynthesis is the only significant solar energy storage process on Earth and is the source of all of our food and most of our energy resources. An understanding of the origin and evolution of photosynthesis is therefore of substantial interest, as it may help to explain inefficiencies in the process and point the way to attempts to improve various aspects for agricultural and energy applications.
A wealth of evidence indicates that photosynthesis is an ancient process that originated not long after the origin of life and has evolved via a complex path to produce the distribution of types of photosynthetic organisms and metabolisms that are found today ( Blankenship, 2002 ; Björn and Govindjee, 2009 ). Figure 1 shows an evolutionary tree of life based on small-subunit rRNA analysis. Of the three domains of life, Bacteria, Archaea, and Eukarya, chlorophyll-based photosynthesis has only been found in the bacterial and eukaryotic domains. The ability to do photosynthesis is widely distributed throughout the bacterial domain in six different phyla, with no apparent pattern of evolution. Photosynthetic phyla include the cyanobacteria, proteobacteria (purple bacteria), green sulfur bacteria (GSB), firmicutes (heliobacteria), filamentous anoxygenic phototrophs (FAPs, also often called the green nonsulfur bacteria), and acidobacteria ( Raymond, 2008 ). In some cases (cyanobacteria and GSB), essentially all members of the phylum are phototrop2hic, while in the others, in particular the proteobacteria, the vast majority of species are not phototrophic.
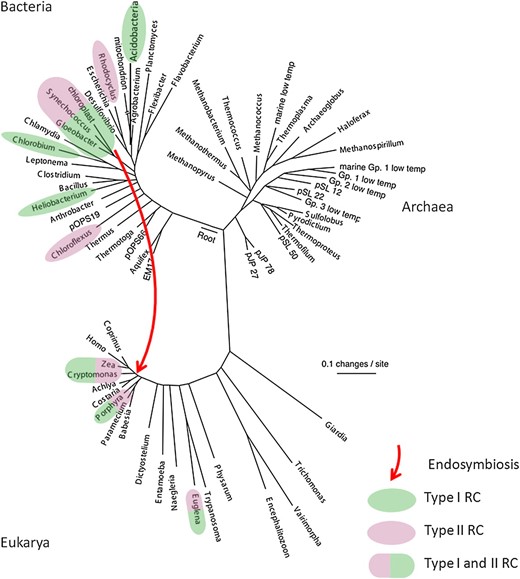
Small subunit rRNA evolutionary tree of life. Taxa that contain photosynthetic representatives are highlighted in color, with green highlighting indicating a type I RC, while purple highlighting indicates a type II RC. The red arrow indicates the endosymbiotic event that formed eukaryotic chloroplasts. Tree adapted from Pace (1997) .
Overwhelming evidence indicates that eukaryotic photosynthesis originated from endosymbiosis of cyanobacterial-like organisms, which ultimately became chloroplasts ( Margulis, 1992 ). So the evolutionary origin of photosynthesis is to be found in the bacterial domain. Significant evidence indicates that the current distribution of photosynthesis in bacteria is the result of substantial amounts of horizontal gene transfer, which has shuffled the genetic information that codes for various parts of the photosynthetic apparatus, so that no one simple branching diagram can accurately represent the evolution of photosynthesis ( Raymond et al., 2002 ). However, there are some patterns that can be discerned from detailed analysis of the various parts of the photosynthetic apparatus, so some conclusions can be drawn. In addition, the recent explosive growth of available genomic data on all types of photosynthetic organisms promises to permit substantially more progress in unraveling this complex evolutionary process.
While we often talk about the evolution of photosynthesis as if it were a concerted process, it is more useful to consider the evolution of various photosynthetic subsystems, which have clearly had distinct evolutionary trajectories. In this brief review we will discuss the evolution of photosynthetic pigments, reaction centers (RCs), light-harvesting (LH) antenna systems, electron transport pathways, and carbon fixation pathways. These subsystems clearly interact with each other, for example both the RCs and antenna systems utilize pigments, and the electron transport chains interact with both the RCs and the carbon fixation pathways. However, to a significant degree they can be considered as modules that can be analyzed individually.
We know very little about the earliest origins of photosynthesis. There have been numerous suggestions as to where and how the process originated, but there is no direct evidence to support any of the possible origins ( Olson and Blankenship, 2004 ). There is suggestive evidence that photosynthetic organisms were present approximately 3.2 to 3.5 billion years ago, in the form of stromatolites, layered structures similar to forms that are produced by some modern cyanobacteria, as well as numerous microfossils that have been interpreted as arising from phototrophs ( Des Marais, 2000 ). In all these cases, phototrophs are not certain to have been the source of the fossils, but are inferred from the morphology or geological context. There is also isotopic evidence for autotrophic carbon fixation at 3.7 to 3.8 billion years ago, although there is nothing that indicates that these organisms were photosynthetic. All of these claims for early photosynthesis are highly controversial and have engendered a great deal of spirited discussion in the literature ( Buick, 2008 ). Evidence for the timing of the origin of oxygenic photosynthesis and the rise of oxygen in the atmosphere is discussed below. The accumulated evidence suggests that photosynthesis began early in Earth’s history, but was probably not one of the earliest metabolisms and that the earliest forms of photosynthesis were anoxygenic, with oxygenic forms arising significantly later.
Chlorophylls are essential pigments for all phototrophic organisms. Chlorophylls are themselves the product of a long evolutionary development, and can possibly be used to help understand the evolution of other aspects of photosynthesis. Chlorophyll biosynthesis is a complex pathway with 17 or more steps ( Beale, 1999 ). The early part of the pathway is identical to heme biosynthesis in almost all steps and has clearly been recruited from that older pathway. The later steps include the insertion of magnesium and the elaboration of the ring system and its substituents. The earliest version of the pathway (and that used by most modern anoxygenic photosynthetic organisms) almost certainly was anaerobic, both not requiring and not tolerating the presence of O 2 . However, all modern oxygenic photosynthetic organisms now require O 2 as an oxidant at several steps in the pathway. This has been explained in terms of gene replacement of the genes coding for the enzymes at these steps, with the result that the overall pathway is unchanged but the enzymes at key steps are completely different in different groups of phototrophs ( Raymond and Blankenship, 2004 ).
A key concept in using chlorophyll biosynthesis pathways to infer the evolution of photosynthesis is the Granick hypothesis, which states that the biosynthetic pathway of chlorophyll recapitulates the evolutionary sequence ( Granick, 1965 ). This is an appealing idea and probably at least partly true. However, in some cases, in particular the situation of chlorophyll and bacteriochlorophyll, it has been argued that the strict version of the Granick hypothesis is misleading and other interpretations are more likely ( Blankenship, 2002 ; Blankenship et al., 2007 ).
All photosynthetic organisms contain carotenoids, which are essential for photoprotection, usually also function as accessory pigments, and in many cases serve as key regulatory molecules. Carotenoids, unlike chlorophylls, are also found in many other types of organisms, so their evolutionary history may reflect many other functions in addition to photosynthesis ( Sandman, 2009 ).
The RC complex is at the heart of photosynthesis; so much attention has been paid to understand the evolution of RCs. A wealth of evidence, including structural, spectroscopic, thermodynamic, and molecular sequence analysis, clearly segregates all known RCs into two types of complexes, called type I and type II ( Blankenship, 2002 ). Anoxygenic phototrophs have just one type, either type I or II, while all oxygenic phototrophs have one of each type. The primary distinguishing feature of the two types of RCs are the early electron acceptor cofactors, which are FeS centers in type I RCs and pheophytin/quinone complexes in type II RCs. The distribution of RC types on the tree of life is shown in Figure 1 and a comparative electron transport diagram that compares the different RCs in different types of organisms is shown in Figure 2 , with type I RCs color coded green and type II RCs color coded purple.
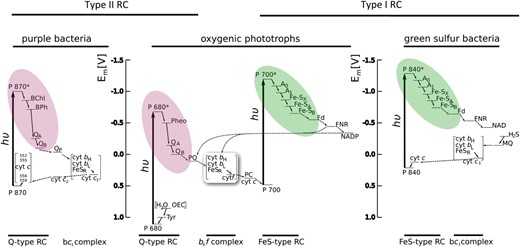
Electron transport diagram indicating the types or RCs and electron transport pathways found in different groups of photosynthetic organisms. The color coding is the same as for Figure 1 and highlights the electron acceptor portion of the RC. Figure courtesy of Martin Hohmann-Marriott.
Further analysis strongly suggests that all RCs have evolved from a single common ancestor and have a similar protein and cofactor structure. This is clearly seen when structural overlays of both type I and II RCs are made, showing a remarkably conserved three-dimensional protein and cofactor structure, despite only minimal residual sequence identity ( Sadekar et al., 2006 ). These comparisons have been used to derive structure-based evolutionary trees that do not rely on sequence alignments. Figure 3 shows a schematic evolutionary tree of RCs that is derived from this sort of analysis. It proposes that the earliest RC was intermediate between type I and II (type 1.5) and that multiple gene duplications have given rise to the heterodimeric (two related yet distinct proteins that form the core of the RC) complexes that are found in most modern RCs.
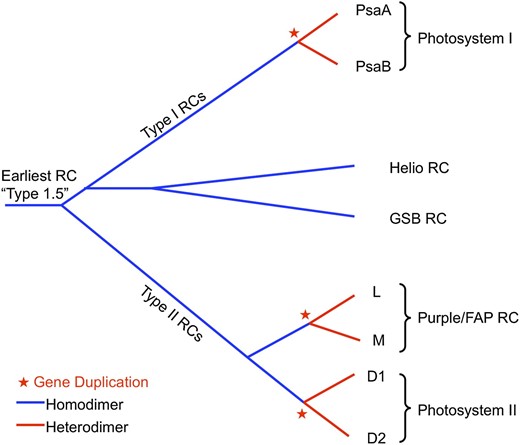
Schematic evolutionary tree showing the development of the different types of RC complexes in different types of photosynthetic organisms. This tree is based on structural comparisons of RCs by Sadekar et al. (2006) . Blue color coding indicates protein homodimer, while red indicates protein heterodimer complexes. Red stars indicate gene duplication events that led to heterodimeric RCs. Helio, Heliobacteria; GSB, green sulfur bacteria; FAP, filamentous anoxygenic phototroph.
A second important issue that relates to RC evolution is the question of how both type I and II RCs came to be in cyanobacteria, while all other photosynthetic prokaryotes have only a single RC. The various proposals that have been made to explain this fact can all be divided into either fusion or selective loss scenarios or variants thereof ( Blankenship et al., 2007 ). In the fusion hypothesis, the two types of RCs develop separately in anoxygenic photosynthetic bacteria and are then brought together by a fusion of two organisms, which subsequently developed the ability to oxidize water. In the selective loss hypothesis, the two types of RCs both evolved in an ancestral organism and then loss of one or the other RC gave rise to the organisms with just one RC, while the ability to oxidize water was added later. Both scenarios have proponents, and it is not yet possible to choose between them.
The primary photochemistry and several of the early secondary electron transfer reactions take place within the RC complex. However, additional electron transfer processes are necessary before the process of energy storage is complete. These include the cytochrome bc 1 and b 6 f complexes. These complexes oxidize quinols produced by photochemistry in type II RCs or via cyclic processes in type I RCs and pumps protons across the membrane that in turn contribute to the proton motive force that is used to make ATP. All phototrophic organisms have a cytochrome bc 1 or b 6 f complex of generally similar architecture, with the exception of the FAP phylum of anoxygenic phototrophs ( Yanyushin et al., 2005 ). This group contains instead a completely different type of complex that is called alternative complex III. The evolutionary origin of this complex is not yet clear. While the cytochrome bc 1 and b 6 f complexes are similar in many ways, the cytochrome c 1 and f subunits are very different and are almost certainly of distinct evolutionary origin ( Baniulis et al., 2008 ).
All photosynthetic organisms contain a light-gathering antenna system, which functions to collect excitations and transfer them to the RC where the excited state energy is used to drive photochemistry ( Green and Parson, 2003 ). While the presence of an antenna is universal, the structure of the antenna complexes and even the types of pigments used in them is remarkably varied in different types of photosynthetic organisms. This very strongly suggests that the antenna complexes have been invented multiple times during the course of evolution to adapt organisms to particular photic environments. So while evolutionary relationships are clear among some categories of antennas, such as the LH1 and LH2 complexes of purple bacteria and the LHCI and LHCII complexes of eukaryotic chloroplasts, it is not possible to relate these broad categories of antennas to each other in any meaningful way. This is in contrast to the RCs, where all available evidence clearly points to a single origin that has subsequently undergone a complex evolutionary development.
Most phototrophic organisms are capable of photoautotrophic metabolism, in which inorganic substrates such as water, H 2 S, CO 2 , or HCO 3 − are utilized along with light energy to produce organic carbon compounds and oxidized donor species. However, there are some groups of phototrophs that cannot carry out photoautotrophic metabolism and there are at least three entirely separate autotrophic carbon fixation pathways that are found in different types of organisms ( Thauer, 2007 ). By far the dominant carbon fixation pathway is the Calvin-Benson cycle, which is found in all oxygenic photosynthetic organisms, and also in most purple bacteria. The GSB use the reverse tricarboxylic acid cycle, and many of the FAPs use the 3-hydroxypropionate cycle ( Zarzycki et al., 2009 ). The Gram-positive heliobacteria lack any known autotrophic carbon fixation pathway and usually grow photoheterotrophically ( Asao and Madigan, 2010 ). Similarly, the aerobic anoxygenic phototrophs, which are closely related to the purple bacteria, lack any apparent ability to fix inorganic carbon. In the latter case, it seems most likely that the ancestor of this group contained the Calvin-Benson cycle but lost the genes because of their obligate aerobic lifestyle ( Swingley et al., 2007 ).
The carbon fixation machinery is thus similar to the antennas, in that several entirely separate solutions have been adopted by different classes of phototrophic organisms. This would be consistent with the idea that the earliest phototrophs were photoheterotrophic, using light to assimilate organic carbon, instead of being photoautotrophic. The ability to fix inorganic carbon was then added to the metabolism somewhat later during the course of evolution, possibly borrowing carbon fixation pathways that had developed earlier in autotrophic nonphotosynthetic organisms.
Perhaps the most widely discussed yet poorly understood event in the evolution of photosynthesis is the invention of the ability to use water as an electron donor, producing O 2 as a waste product and giving rise to what is now called oxygenic photosynthesis. The production of O 2 and its subsequent accumulation in the atmosphere forever changed the Earth and permitted the development of advanced life that utilized the O 2 during aerobic respiration. Several lines of geochemical evidence indicate that free O 2 began to accumulate in the atmosphere by 2.4 billion years ago, although the ability to do oxygenic photosynthesis probably began somewhat earlier ( Buick, 2008 ). In order for O 2 to accumulate, it is necessary that both the biological machinery needed to produce it has evolved, but also the reduced carbon produced must be buried by geological processes, which are controlled by geological processes such as plate tectonics and the buildup of continents. So the buildup of O 2 in the atmosphere represents a coming together of the biology that gives rise to O 2 production and the geology that permits O 2 to accumulate.
Oxygen is produced by PSII in the oxygen evolving center, which contains a tetranuclear manganese complex. The evolutionary origin of the oxygen evolving center has long been a mystery. Several sources have been suggested, but so far no convincing evidence has been found to resolve this issue ( Raymond and Blankenship, 2008 ). The possibility that functional intermediate stages existed that connect the anoxygenic type II RCs to PSII seems likely ( Blankenship and Hartman, 1998 ).
The process of photosynthesis originated early in Earth’s history, and has evolved to its current mechanistic diversity and phylogenetic distribution by a complex, nonlinear process. Current evidence suggests that the earliest photosynthetic organisms were anoxygenic, that all photosynthetic RCs have been derived from a single source, and that antenna systems and carbon fixation pathways have been invented multiple times.
Asao M Madigan MT ( 2010 ) Taxonomy, phylogeny, and ecology of the heliobacteria . Photosynth Res 104 : 103 – 111
Google Scholar
Baniulis D Yamashita E Zhang H Hasan SS Cramer WA ( 2008 ) Structure-function of the cytochrome b 6 f complex . Photochem Photobiol 84 : 1349 – 1358
Beale S ( 1999 ) Enzymes of chlorophyll biosynthesis . Photosynth Res 60 : 43 – 73
Björn LO Govindjee ( 2009 ) The evolution of photosynthesis and chloroplasts . Curr Sci 96 : 1466 – 1474
Blankenship RE ( 2002 ) Molecular Mechanisms of Photosynthesis . Blackwell Science, Oxford
Blankenship RE Hartman H ( 1998 ) The origin and evolution of oxygenic photosynthesis . Trends Biochem Sci 23 : 94 – 97
Blankenship RE Sadekar S Raymond J ( 2007 ) The evolutionary transition from anoxygenic to oxygenic photosynthesis . In Falkowski P Knoll AN , eds , Evolution of Aquatic Photoautotrophs . Academic Press , New York , pp 21–35
Buick R ( 2008 ) When did oxygenic photosynthesis evolve? Philos Trans R Soc Lond B Biol Sci 363 : 2731 – 2743
Des Marais DJ ( 2000 ) Evolution: When did photosynthesis emerge on Earth? Science 289 : 1703 – 1705
Granick S ( 1965 ) Evolution of heme and chlorophyll . In Bryson G Vogel HJ , eds , Evolving Genes and Proteins . Academic Press , New York , pp 67–88
Green BR Parson WW eds ( 2003 ) Light-Harvesting Antennas . Kluwer, Dordrecht , The Netherlands
Margulis L ( 1992 ) Symbiosis in Cell Evolution . WH Freeman , San Francisco
Olson JM Blankenship RE ( 2004 ) Thinking about the evolution of photosynthesis . Photosynth Res 80 : 373 – 386
Pace NR ( 1997 ) A molecular view of microbial diversity and the biosphere . Science 276 : 734 – 740
Raymond J ( 2008 ) Coloring in the tree of life . Trends Microbiol 16 : 41 – 43
Raymond J Blankenship RE ( 2004 ) Biosynthetic pathways, gene replacement and the antiquity of life . Geobiology 2 : 199 – 203
Raymond J Blankenship RE ( 2008 ) The origin of the oxygen-evolving complex . Coord Chem Rev 252 : 377 – 383
Raymond J Zhaxybayeva O Gerdes S Gogarten JP Blankenship RE ( 2002 ) Whole genome analysis of photosynthetic prokaryotes . Science 298 : 1616 – 1620
Sadekar S Raymond J Blankenship RE ( 2006 ) Conservation of distantly related membrane proteins: photosynthetic reaction centers share a common structural core . Mol Biol Evol 23 : 2001 – 2007
Sandman G ( 2009 ) Evolution of carotenoid desaturation: the complication of a simple pathway . Arch Biochem Biophys 483 : 169 – 174
Swingley WD Gholba S Mastrian SD Matthies HJ Hao J Ramos H Acharya CR Conrad AL Taylor HL Dejesa LC et al. ( 2007 ) The complete genome sequence of Roseobacter denitrificans reveals a mixotrophic rather than photosynthetic metabolism . J Bacteriol 189 : 683 – 690
Thauer RK ( 2007 ) A fifth pathway of carbon metabolism . Science 318 : 1732 – 1733
Yanyushin MF del Rosario M Brune DC Blankenship RE ( 2005 ) A new class of bacterial membrane oxidoreductases . Biochemistry 44 : 10037 – 10045
Zarzycki J Brecht V Muller M Fuchs G ( 2009 ) Identifying the missing steps of the autotrophic 3-hydroxypropionate CO 2 fixation cycle in Chloroflexus aurantiacus . Proc Natl Acad Sci USA 106 : 21317 – 21322
Author notes
Email alerts, citing articles via.
- Recommend to Your Librarian
- Advertising & Corporate Services
- Awards & Funding
- Plant Science Today
- Plant Biology Meeting
- Meeting Management Services
- Plant Science Research Weekly
- Taproot: A Plantae Podcast
Affiliations
- Online ISSN 1532-2548
- Print ISSN 0032-0889
- Copyright © 2024 American Society of Plant Biologists
- About Oxford Academic
- Publish journals with us
- University press partners
- What we publish
- New features
- Open access
- Institutional account management
- Rights and permissions
- Get help with access
- Accessibility
- Advertising
- Media enquiries
- Oxford University Press
- Oxford Languages
- University of Oxford
Oxford University Press is a department of the University of Oxford. It furthers the University's objective of excellence in research, scholarship, and education by publishing worldwide
- Copyright © 2024 Oxford University Press
- Cookie settings
- Cookie policy
- Privacy policy
- Legal notice
This Feature Is Available To Subscribers Only
Sign In or Create an Account
This PDF is available to Subscribers Only
For full access to this pdf, sign in to an existing account, or purchase an annual subscription.

- GPU Computing
- Cloud Computing
- Lattice Microbes
- Atomic Resolution Brownian Dynamics
- VND (Neuronal)
Bringing Physics To Life
Bringing computing to life, picture and movie galleries, tcb group members, publications, historical series: photosynthesis, unraveling photosynthesis step by step:, four decades of research in theoretical and computational biophysics, by lisa pollack, early influences on klaus schulten.
“Today you think it's exciting, but in those days it was considered the most boring thing you could do, to study molecules,” remarks Klaus Schulten on his decision in 1969 to pursue a PhD in molecular physics. “That was done in the 1930s, and even then it was boring.” But Schulten had a master plan, and he surmised molecular physics was the right field to pursue in graduate school, never tempted by the discoveries promised in the hot new field of particle physics. He wanted to study biology, but specifically at the level of atoms and molecules, and he imagined that physics would give him the tools he needed to accomplish this.

From a very early age Schulten was fascinated by biology. A voracious reader in all areas of science, he discovered, around age twelve, Du und das Leben (You and Life), a book by the eminent biologist Karl von Frisch, and the contents captivated him. “He was a great biologist who very early on appreciated the molecular basis of living systems. So he brought the two together and wrote on what life is, but that it comes from the molecules.” In fact, there was one particular chapter that caught his attention, about how cells employ photosynthesis to make ATP, the molecule that fuels life. “I felt that was my calling,” Schulten says; “I wanted to understand how life forms use energy to make ATP and then run many processes with it.”
Through high school Schulten continued to read on his own about science, finding very little stimulating on it in the classroom. In fact, because he liked to challenge his teachers with the things he taught himself, he was given the nickname “Professor” by his classmates. “When I got my degree at the high school, the teachers asked me, what do you want to study, and I said I want to study it all: chemistry, physics, mathematics, and biology. And that, of course, was further proof for them that I was totally crazy.”
When Schulten was in his early twenties, studying for his diploma at the University of Münster, he often walked in its famed botanical garden with fellow students. “I discussed at length with my friends what I wanted to do with my life, and that is exactly what I do today.” But what he wanted to do didn't exist at the timehe wanted to look inside living cells, at the level of electrons and atoms, to understand how they functioned; and he wanted to do it theoretically instead of experimentally.
The story charted in the following pages will reveal how a life's calling was realized over the course of forty-plus years. In its beginning stages, a tapestry of people in Schulten's early adulthood, when woven together, led to a familiarity and then a fascination with photosynthesis. Despite this fascination, Schulten at one point had to wait ten years before he could do the kinds of calculations he deemed worthy of publication: “What I really like about the photosynthesis is it goes from the molecule, even the electron, to the whole cell. And that is basically what I always wanted to do; that is why I stuck with it for a long time.”
Instead of plants, Schulten chose to study purple bacteria, which embody the simplest form of photosynthesis. Bacteria emerged around 3.5 billion years ago on the earth; photosynthesis, an ancient process, arose sometime thereafter, although no firm evidence exists to prove its exact origins. While many associate it with plants, photosynthesis most likely originated in bacteria, and photosynthesis is found in many different phyla of bacteria today, purple bacteria being one of them. The purple bacteria are a gift for scientists studying photosynthesis, as the machine or unit that runs photosynthesis in these bacteria is simpler than the ones in plants.

Even in its least complicated incarnation, Schulten never dreamt that he could understand thoroughly this phenomenon when he started the field in the 1970s. But forty years of dedication has paid off. “At the end, today,” summarizes Schulten, “I think we are pretty close to really going full circle and understanding everything.” This history documents how the pieces all fell together and, after setbacks and dry-spells, resulted in an impressive compendium of work by many scientists from different fields who worked with Schulten on a topic so key for life on earth.
Graduate School at Harvard: The Invisible States
When Schulten finished his diploma in physics at Münster, he carefully considered his next step, one that would bring him closer to his childhood dream. As a youth he had a mathematical mind and a love for living systems. At the school aquarium he wanted to understand the fish and the plants, and why they were alive. “I was never interested in just a feather, or in a bone,” he recounts about his youth. “It was more like this living entity that all comes together, that's what interested me.” When deciding on graduate schools, he asked around and the only person at the time who combined physics with living systems was Martin Karplus at Harvard.
Formally trained as a theoretical chemist, having worked with Linus Pauling at Cal Tech in graduate school, Martin Karplus spent many years doing chemistry-related research before he returned to his first love, biology. In the fall of 1969 he took a leave of absence from his position as a chemistry professor at Harvard to visit the Weizmann Institute in Israel to uncover how the tools of a theoretical chemist could be applied to biological problems. He came back from the institute with a host of problems in biology to study, his stay a success.
Around this same time, Klaus Schulten decided he wanted to work with Karplus on his PhD, for he knew Karplus combined mathematics, physics, chemistry and biology in his research, a long-held goal for Schulten. With a fellowship from the Volkswagen Foundation, Schulten began his studies, and would eventually receive a degree in chemical physics.
One of the areas of study Karplus chose to pursue after his stay in Israel was the role of retinal in vision. Retinal is related to the carotenoids, for basically half a carotenoid molecule is vitamin A, and when oxidized vitamin A becomes retinal. Retinal also is one example of a polyene, a type of chemical compound with multiple carbon-carbon bonds. Polyenes play key roles in light absorption in vision as well as in photosynthesis. Studying polyenes would become a major focus of the Karplus group at Harvard.

Karplus, in his various jobs over the years, always appreciated the fresh perspectives he gained from talking to the other colleagues at his institution. One of the fuels that fed Karplus's polyene research came from interactions with a new assistant professor in chemistry, Bryan Kohler, whose experiments intrigued Karplus. With his graduate student Bruce Hudson, Kohler had found that when they shined light on certain polyenes and looked at the resulting excitation bands, there was a little excitation they couldn't explain. The wisdom at the time held that there was some dirt in the solution they couldn't clean away, which was responsible for this weak absorption. “So then they cleaned and cleaned but it didn't go away,” recalls Schulten about this mystery. “And they went to my advisor, Karplus, and asked him if there is a chance that there is some electronic excitation there. I was just a new student of Karplus's, and so when you have a new student you also have a new victim.”
Karplus and Schulten decided to employ the so-called electron correlation effect to see what would happen if they excited a pair of electrons in the polyene in a coordinated manner. “You might say if you lift two electrons up that's crazy,” says Schulten about this theoretical approach. “That costs twice as much energy, but that's not necessarily so. And because the electrons might do it in such a coordinated way that the excitation is actually lower in energy than moving just a single one, that is what I was going to try out.”
Schulten started this work in the fall of 1971, and actually stayed in Boston over the subsequent winter break to investigate this new problem and prove the existence of forbidden, low-lying states. He vividly remembers a snowstorm during that time, which almost paralyzed the region; Schulten was barely able to get out and buy food. But that was not his greatest concern: “I was always worried that Kohler and Hudson would come through the door any moment and tell me, 'Oh, sorry, we found a better cleaning agent and now the effect is gone.'”
Because it was winter break, Schulten had the office and computer center all to himself. “I worked there day and night,” Schulten recalls. “Basically I remember that I only stopped working when my hand got cramped from holding the pen, because I had to derive all these quantum mechanical rules that describe the system.”
Schulten was very careful, however, and even did the calculations two or three times to make sure he made no mistakes. His diligence paid off; when Karplus returned from break, Schulten showed him proof of the mysterious low-lying states. “These other states were not seen because they are what physicists would call magnetic,” explains Schulten. “They actually involve the spins, and spins are basically magnetic moments.” His work revealed that polyenes have certain triplet-triplet excitations responsible for the feeble excitations the experimentalists witnessed. (A triplet has two electrons whose total spin adds to unity.) “If you take two triplet excitations in the polyene and add them up,” spells out Schulten, “you are getting an excitation energy that is still lower than the lowest excitation that is seen in an optical transition.”
Publishing this new finding, however, was not straightforward. Other researchers claimed that what Kohler and Hudson saw was a well-known “dirt effect” and not something real. They actually claimed the dirt was in the window of the cuvette and not in the polyenes themselves. Schulten, a rookie in computational biology at that point, summarizes how the paper was likely received by some in the scientific community: “Do you know what foolish thing Karplus did? He wrote a paper on this dirt effect.” But Schulten and Karplus persevered and eventually published their findings. Schulten's understanding of these polyene excitations would later inform his photosynthesis research.
While Schulten was applying techniques of theoretical chemistry and physics to biological problems in his formal studies, his life outside the classroom at Harvard was enriching his mind just as much, and helping to shape his future research interests. He loved to spend free time in the biology labs of George Wald and Ruth Hubbard, a power couple on campus. “Several times they threatened to kick me out,” recalls Schulten, “because I was too arrogant. I was a physicist, and so I always thought I was so much smarter than all the biologists.” George Wald received the Nobel Prize in 1967 for having discovered that retinal is used by all species with eyesight. Vision, like photosynthesis, is a process involving the interaction of biomolecules with light and Schulten learned about retinal and the carotenoids from Wald. “He was a real big influence on me,” Schulten muses; “I was interested in vision but then I knew of course that these kind of molecules also play a role in photosynthesis.”
The Boston area around Harvard was a vibrant environment, especially for young scientists. While living there Schulten also heard about some work on carotenoids by the Tufts biochemist Norman Krinsky. Known later as the father of modern carotenoid research, one of the many things Krinsky worked on was studying the protective role of carotenoids. Although carotenoids are pigments that absorb light, Schulten heard about research that proposed another role for the carotenoids: as quenchers of excited oxygen. Krinsky and many others working in the field in the 1960s and 1970s found that the length of carotenoids was related to their protective function, and that mutant strains with shorter-than-normal lengths would not exhibit protection for the bacteria; that is, bacteria with shorter carotenoids would die when exposed to light. Schulten grew fascinated upon hearing about this other role for carotenoids, as protectors of living organisms.
Max Planck Institute for Biophysical Chemistry
Schulten got to work straightaway. “I figured out where it comes from,” he explains. “And I also figured out that you could verify it by doing the reaction with and without magnetic fields.” What Schulten essentially discovered is that a magnetic field can influence a chemical reaction, a new physical effect. He immediately published an initial, qualitative paper suggesting the effect, and then, teaming up with his wife, Zan Luthey-Schulten, and Hans-Joachim Werner, a student in the group that Schulten was mentoring, they presented the full quantitative theory of the effect in two papers published in 1977.
Because the computing facilities at Göttingen were not stellar, the three had to use sophisticated mathematical tricks for this mammoth feat. “We achieved a numerical solution,” recounts Schulten, “of the problem of how two molecules that transfer electrons between them, and carry their unpaired electron spins that move around and back, how they can react with each other in a solvent.”
Around 2010 Schulten found himself refereeing a paper for Nature by a well-known physics group, and was surprised to discover that they presented exactly the same computations that the three theorists at the Max Planck Institute had painstakingly carried out over three decades earlier, without the benefit of sophisticated computers. As Schulten sees it, “something that people were proud of in 2010 we did in 1976.” But Schulten and his collaborators were not satisfied with just detailing the qualitative theory behind this new magnetic field effect. They began to think about all the ways it could be applied.
In electron transfer, an electron moves from one place to another, a seemingly simple process. But Weller and collaborators had worked for many years fine-tuning a system of donor and acceptor molecules that was optimal for making measurements in the laboratory. While Weller's pet system, pyrene and dimethylaniline, was a chemical system, the scientists who worked for Weller at the institute were fully aware that electron transfer was an important process in biological systems as well. Since Schulten knew about electron transfer in biological systems, he started thinking about application of the new magnetic field effect to biological systems, ones beyond the chemical systems favored in Weller's group. “Basically, when you have a new physical effect,” notes Schulten, “like this magnetic field effect, you very often can use it as a measuring stick.” But what kind of measuring stick is optimal? “The measuring sticks that you usually are looking for are ones that observe processes that are too miniscule in terms of size, and too fast in terms of time, to observe easily in experiment.” In other words, all one needed to do was put the magnetic field on the system, then take it away, and see if there was a different outcome.
Electron transfer processes were known to be involved in photosynthesis. Being in an electron transfer group, Schulten says that in the back of everybody's minds was an awareness of just how important electron transfer was to photosynthesis. So in 1978 Schulten and Werner, along with their director, Albert Weller, published a paper suggesting that the new effect could be used as a ruler to characterize in more detail the electron transfer that occurs in photosynthesis. The goal was to use the magnetic field effect to understand better the electron transfer in photosynthesis.
There were many reasons why Schulten already knew enough about electron transfer in photosynthesis to suggest using the magnetic field effect as a yardstick in his 1978 paper. The electron transfer in photosynthesis occurred in a protein called the photosynthetic reaction center, which may be thought of as the heart of the photosynthetic unit. When plants and bacteria absorb energy from the sun, this energy is used by the reaction center to transfer an electron. The workings of the reaction center were not fully understood because the structure of this protein was not known in the late 1970s when Schulten was in Göttingen. “So people actually did very well,” recalls Schulten, “to conclude, from certain optical properties and from electron transfer rates, what the structure looked like. So it was amazingly good actually, but it was not firm.”
One of the groups that did, however, study these electron transfer reactions, despite the uncertainty about the reaction center structure, was located in the Netherlands, in Leiden. Schulten knew them and their work quite well. Led by Arnold Hoff, they studied magnetic resonance in photosynthesis, and Schulten was impressed by how far they got without knowing the exact structure of the reaction center. When Schulten published the 1978 paper suggesting that the new magnetic field effect be used as a yardstick to characterize electron transfer in photosynthesis, Albert Weller, his boss, was one of the co-authors. Referring to this paper, Arnold Hoff told Schulten that Hoff should have been an author on the publication too. “Now Hoff didn't do anything,” recounts Schulten, “so he didn't have a reason to be an author of this paper. But he said that Weller had even less of a reason.” Schulten did not buy this argument, as he felt that Weller ran a great institute that inspired and enriched him as a scientist. “People ignore a little bit the role that the big guys play,” says Schulten. “They not only give you a seat to sit on, they also give you ideas. They sell the whole of science by saying why it is important, and what will be done in the next few years. If they wouldn't do that, one couldn't do one's work.”

While Schulten knew about the Leiden group and their work, another reason he was aware of current photosynthesis research he attributes to being in Germany. “Science has strong local aspects,” he explains, “so that certain feats of science are done more in one country than another, for example. And photosynthesis was studied a lot in Germany.” One of those hubs was located in the Technical University of Berlin, at the Max-Volmer Institute for Physical Chemistry, which was really put on the map by Horst Witt. Schulten visited the institute often and learned a great deal about Witt's efforts, which focused on explaining oxygen-generating photosynthesis. “He was very bold,” Schulten notes. “He did it actually before one knew much, but he really struggled hard to give a picture of what could be involved. And that really also gave me a broader picture of photosynthesis as a biological process that involves many different steps and many different proteins. That motivated me to understand how living cells actually combine many steps to have a good outcome.”
Besides the groups in Berlin and Leiden, Göttingen also shaped Schulten's views on the complexity of biological processes that would later inform his own photosynthesis work. One of those Göttingen influences was a man who worked there at the institute, Manfred Eigen. In 1967 Eigen received the Nobel Prize in chemistry for having studied very fast reactions. Although trained in physics and chemistry, his attention turned to biological processes later in his career, especially to the origin of life. “Here we have the molecules,” Schulten says of Eigen's views on biological organization, “and here we have the whole cell: surviving, producing enough energy for its survival, and doing so many levels of reactions. And to begin to understand the challenges involved in organizing all those levels was really the program of Eigen's intellectual life in a way.” This influenced Schulten to always consider biological organization in a living cell.
While Schulten went to lectures by Eigen in Göttingen, he also participated in the famous annual gathering known as the Winter Seminar, in Klosters, Switzerland. There Schulten learned to ski during the days and listened to cutting-edge talks at night. Started in 1965, this gathering first encompassed only coworkers at the institute. In an online video, Manfred Eigen discusses how the Winter Seminar originated: “We should go away from the lab, because in the lab you have your daily life here and you should really go to some nice silent place. And since there are so many meetings in summertime, you say, let's have a winter seminar and let's discuss there our problems. And if we have time left, let's go skiing.” Later the seminar grew in popularity and attracted an international following; Eigen estimates that between 30 and 50 Nobel Prize winners attended in the course of its run over several decades. The seminar was unique, as it purposely did not require an abstract and manuscript and completely finished work, as most meetings did at the time. “In other words,” continues Eigen in a second video, “people are uninhibited to talk about things because they are not pinned down. They can speculate in the seminar, and that makes it lively.” Being near a force like Manfred Eigen, who wanted to understand biological complexity, encouraged Schulten to think of photosynthesis as an overall process that, although it involves many steps, should be viewed as a unit.
Göttingen also brought Schulten's attention to a critical equation by physical chemist Theodor Förster, via his supervisor Albert Weller. Schulten knew about the various accomplishments of German Theodor Förster because Weller had worked with Förster for many years studying protolytic reactions in the 1950s. From 1946 to 1949 Förster published three papers that described how an excited molecule transfers its excitation to an acceptor molecule. Soon becoming known as Förster Resonance Energy Transfer, or FRET, this mechanism now made it possible to describe how one chlorophyll transfers its excitation to a nearby chlorophyll during photosynthesis. “Förster made a tremendous contribution to science with it, way beyond photosynthesis,” remarks Schulten on this equation. “His formula and his idea are used today all over the world in many laboratories.”
While the accomplishments of Förster and his famous equation came to Schulten naturally through Weller's lineage to Förster, Schulten is quick to point out that Förster was not the only one in the 1940s to wonder at the mechanism of excitation transfer between pigments in photosynthesis. The famous physicist Robert Oppenheimer, known for his work on the Manhattan Project during World War II, published in the minutes of the American Physical Society a brief abstract in 1941 entitled “Internal Conversion in Photosynthesis,” right before he got caught up with the war effort. In the abstract he addresses excitation transfer from certain pigments (namely, fluorescent dyes) to chlorophylls in photosynthetic algae, suggesting the transfer is a large-scale model of internal conversion of gamma rays. After the war, in a 1950 paper with William Arnold, the pair elaborated on the mechanism of excitation transfer between chlorophyll and phycocyanin, a pigment used as a dye. William Arnold had a background in physics and biology and had worked on experimental aspects of photosynthesis since the 1930s. Arnold had done some work to show that light absorbed by the pigment phycocyanin was transferred to chlorophylls instead of directly reducing carbon dioxide. When he told Oppenheimer about this finding, they set out to explain the mechanism of excitation transfer. While the pair discussed this idea of excitation transfer as internal conversion sometime around 1939 or 1940, they did not get around to publishing this explicitly until 1950, according to Arnold's autobiographical article.
But why is the Oppenheimer and Arnold work still relatively unknown? Schulten notes that their abstract approach was not particularly helpful for biologists. “Förster was more of a physical chemist, not a physicist, and he had a much better way of explaining what he learned,” says Schulten, about the difference between Förster's and Oppenheimer's strategies. “In particular Förster described very carefully the elementary act of sharing the excitation between two chlorophylls. Oppenheimer knew that, too, but then he immediately averaged over hundreds of them. But what was really very important later was to know the pair behavior.”
Although Schulten published his first paper ever on photosynthesis in 1978 while at the Max Planck Institute, his next foray into the field would come only after a ten-year hiatus. But the serendipitous opportunity that presented itself a decade later would prove a treasure trove for a computational biophysicist like Schulten. The reason for the hiatus was because Schulten realized that for his theoretical purposes, the data was just not therethe structure of the reaction center was still unknown. “One could have done more,” says Schulten, “but it would have always been questioned.”
Living Among Experimentalists
From 1974 to 1980 Schulten worked in Göttingen, and although he only published one paper, among many, on photosynthesis, his time at the Max Planck Institute was critical to his future work in the field. “Göttingen was the place where I learned the most and where my scientific direction was shaped most,” Schulten notes. “And that was clearly due to the fact that I lived among experimentalists.”
Schulten was surrounded by 40 or 50 experimentalists who worked in his group. Schulten calls himself a mathematician by ability, who has a love for life sciences, but acknowledges that, back in the 1970s, it was tricky to apply mathematics to a new field like biology; there was much resistance and uncertainty. Schulten realized that to tackle this precarious task of introducing mathematics to biology he would need to work on solid ground and become thoroughly familiar with the experimental side. His time in Göttingen provided just that opportunity.
First, his experimentalist coworkers questioned Schulten's pure theoretical approach, which only made him improve his methods; there were also lots of good-natured jokes about him being a theoretician. Second, Schulten figured out how to apply a critical eye to experiment. “You need to know what are they doing, how are they doing it, who are the good people, and who are the not-so-good,” Schulten remarks. “So somehow you need to develop a working relationship.” Third, he learned about key directions in the field and heard about cutting-edge experiments while at the institute. In short, his time in Göttingen was not only productive in terms of research, but also because he made many contacts, with experimentalists in particular.
A Task Labeled Impossible
In 1980 Schulten's formative time at the Max Planck Institute came to an end, as permanent positions there were few. He took a job 245 miles away in Bavaria, as a professor in the physics department of the Technical University of Munich. He was still eight years away from revisiting photosynthesis in his own work, but as the decade wore on he had a front row seat to some cutting edge research that would eventually bring him back to this topic.

Southwest of the city center of Munich lies the town of Martinsried and in it is located the Max Planck Institute for Biochemistry. When Schulten moved to Munich in 1980 he began hearing about a fascinating project that was going on at the institute. A young biochemist there in Martinsried was trying to crystallize a membrane protein. Despite the claim in contemporary biochemistry textbooks that it was impossible to form crystals from this kind of protein, Hartmut Michel believed otherwise; an accidental observation had given him hope. Up to that point the proteins that had been crystallized were all solvated in water; since the surface of these proteins are electrically polar, they interact strongly with each other and are likely to form crystals. But membrane proteins live in lipid membranes, which are hydrophobic, and these proteins are largely nonpolar and so these membrane proteins don't interact strongly with each other.
In 1978 Hartmut Michel noticed by chance that the bacteriorhodopsin he was using in an experiment formed glass-like bodies when put in the freezer. At the time he was a post-doc in Dieter Oesterhelt's group in Würzburg, Germany, and Oesterhelt had discovered bacteriorhodopsin, an integral membrane protein, and proposed its function in the early 1970s. With the observation in the freezer, and with Oesterhelt's enthusiastic support, Michel spent the next year trying to form three-dimensional crystals of bacteriorhodopsin. While he was successful in finally obtaining the crystals, he discovered that they were not suitable for structural analysis in X-ray diffraction. Around this time Michel moved with Oesterhelt to Martinsried, where Oesterhelt became a director at the Max Planck Institute there.
But Hartmut Michel was not discouraged by his setbacks with bacteriorhodopsin. He decided to find more suitable membrane proteins and try and crystallize them instead. He chose to work with reaction center proteins from two purple bacteria, and also chlorophylls from spinach. He knew that these protein complexes were said to be part of crystalline arrays in their native environment. Using detergents, and techniques acquired from the bacteriorhodopsin work, in July 1981 Michel finally formed three-dimensional crystals of the reaction center protein of purple bacteria Rhodopseudomonas viridis. But the big test would be whether or not the reaction center crystals were of suitable quality for X-ray diffraction. In September of that year, working with Wolfram Bode, Michel found his crystals diffracted beautifully. Three years after Michel had accidentally observed crystalline-like membrane proteins in a freezer, he finally succeeded in producing high-quality crystals of a membrane protein, a task purported to be impossible. Michel points to 1981 as one of the best years of his life.
Once Hartmut Michel had his high-quality crystals, his next step was to find experts in X-ray crystallography in order to then elucidate the structure of the reaction center. Another group at the institute in Martinsried, led by Robert Huber, specialized in X-ray crystallography of proteins. In the spring of 1982 Michel gave a talk to Huber's group about his membrane protein crystallization work, seeking collaborators. Out of this seminar emerged a colleague for the project: Johann “Hans” Deisenhofer. Having joined Huber in 1971 as a PhD student, and then later converting that to a permanent position in the group, Deisenhofer did not hesitate for long to team up with Michel on the project, even though the reaction center was the largest protein at that time whose structure had yet to be determined and it was not clear that standard methods of structure determination would be suitable for the crystal.
Working at a rapid pace, and with input and support along the way from Robert Huber and Dieter Oesterhelt, the pair managed to solve the complete structure by 1985, helped by the following scientists: Kunio Miki, Otto Epp, Karl Weyer, and Heidi Gruenberg. By 1987 they had refined the structure at 2.3 Å resolution. Following their success, Hartmut Michel moved to Frankfurt in October 1987 to lead a group at the Max Planck Institute for Biophysics, and Hans Deisenhofer went to Dallas, Texas for a position at the Howard Hughes Medical Institute in March of 1988. In the fall of 1988 the two scientists, along with Robert Huber, would win the Nobel Prize in chemistry for determining the three-dimensional structure of the reaction center.
A Bonanza for a Computational Biophysicist
During his time in Munich, Schulten got to know Hartmut Michel and Hans Deisenhofer, and was always eager to hear about their progress on the reaction center over the course of the decade. In fact, Schulten soon realized that with the elucidation of the structure of a reaction center, many new calculations could be done that would have otherwise been impossible before the structure was solved. The reaction center solution had two major effects on Schulten's professional life. First, he returned to the field of photosynthesis, which had fascinated him from boyhood through graduate school. While he briefly touched on this topic in Göttingen, there he also learned about many of the major players at the forefront of photosynthesis research. "THE REACTION CENTER SOLUTION HAD TWO MAJOR EFFECTS ON SCHULTEN'S PROFESSIONAL LIFE." He was, therefore, in a prime position by 1987 to begin his own research on photosynthesis when his colleagues finally determined the reaction center structure. And the second major effect the structure determination had on him involved his long-term goals for working with large biomolecules. Since the size of the reaction center protein was so big, Schulten initiated an audacious plan to acquire a computer powerful enough to handle such a massive protein. While the building of a homemade parallel supercomputer was ultimately influenced by solution of the reaction center, that story is told in detail elsewhere (see this URL for a history of NAMD). Suffice it to say that it led to a software product that would help Schulten realize his dream of describing a living cell via its constituent molecules.
Meanwhile, with the reaction center structure now in hand, in the late 1980s Schulten, working with Michel and Deisenhofer, began a series of molecular dynamics calculations on just a small section of the protein with his graduate student in Munich, Herbert Treutlein. They published a series of papers in 1988 on their molecular dynamics calculations. But one paper, written by Schulten, Deisenhofer and Michel, remained never to be published, a victim of fame. The referee report rejected the paper outright, claiming in this instance that one had to be super critical of high-profile scientists because otherwise the readership would easily believe everything the three famous scientists had written. Schulten is pretty sure this even happened before Michel and Deisenhofer received the Nobel Prize.
In the same year that he jump-started his return to photosynthesis by publishing computational papers that utilized the new reaction center structure, Schulten took a job at the University of Illinois at Urbana-Champaign. Soon after that, in 1989, Schulten founded the Theoretical Biophysics Group there at the Beckman Institute for Advanced Science and Technology, an interdisciplinary center at the university. Eventually the group at Beckman became known as the Theoretical and Computational Biophysics Group. It is through this group that Schulten and collaborators were able to start to piece together the many steps in photosynthesis and begin to understand it on a grand scale
Sir Anthony Leggett and Lord Rayleigh
One of the first projects Schulten embarked on at Beckman was an examination of electron transfer and its coupling to protein motion in the reaction center. This work culled from advances made by one of Schulten's colleagues, as well as one-hundred-year-old mathematics set forth by another famous physicist and Nobel Prize winner. During electron transfer in the reaction center, the electron is affected energetically by how the rest of the protein moves. The atoms in the protein vibrate due to thermal motion, and as the charges on the protein move around, the electrons exhibit slightly different quantum behavior. The reaction center protein has roughly 10,000 atoms, each vibrating in three directions, which means 30,000 vibrations. With a minimum of two parameters needed to describe the electron coupling, the result is 60,000 parameters to keep track of. To characterize this quantum system, Schulten and his graduate student Dong Xu had to dig deep.
A colleague of Schulten's in the physics department, and a next-door neighbor as well since 1988, is Sir Anthony Leggett. In 2003 Leggett would win the Nobel Prize in physics for his work explaining the theory behind superfluidity in helium-3. In 2004 Queen Elizabeth II knighted the London native for his discoveries. After his work in the 1970s that would earn him the Nobel Prize, one of the fields Leggett turned to was quantum mechanics under thermal conditions. Schulten recalls that among the many papers he published related to quantum mechanics, Leggett wrote a huge, comprehensive review article. “It was known in our physics department as the telephone book,” says Schulten, “because when it was a manuscript it was as fat as a telephone book when he sent it to the journal.”
Leggett's work on how quantum systems are affected by thermal disorder gave Dong Xu and Schulten the framework for describing how electrons in principle are coupled to the protein motion. They realized they did not explicitly need to know the 60,000 parameters, but instead could use certain functions that characterized how an electron is being affected by the vibrational motion. Additionally, Dong Xu did some detective work and hit on the mathematics derived by Lord Rayleigh in the late 1800s to describe how sound is generated; Rayleigh's equations worked out how the air, a totally disordered thermal bath, can still be imprinted with systematic coherent behavior to produce sound. “That kind of mathematics could actually be applied to the problem in the reaction center,” notes Schulten. “To understand the quantum motion of electrons, which is also coherent like sound, was the goal. The electron motions are actually being affected by the vibrations in the protein that act pretty much like the atoms in the air, statistically speaking.” Schulten is particularly proud of the elegant mathematical steps published in their papers on this topic.
After completing the electron transfer work, the mid-1990s arrived and brought an experimental collaborator from Schulten's past back into the picture. Little did Schulten realize that one inebriated evening would lead to one of the most highly cited papers in his whole career.
Intrepid Spirit Needed

Sometime around 1994 Hartmut Michel, now in Frankfurt, was visiting the Schulten group at Beckman, invited by Schulten to give a lecture. At a party Schulten hosted during the visit, Schulten and Michel got a bit inebriated and thus a bit emboldened, and decided to solve the structure of a light-harvesting complex Michel had been working on, even though a key piece of information was missing. Up to that point various light-harvesting proteins had been crystallized, but none explicitly solved. The two scientists made a deal: Michel's group would hand over their diffraction data, and Schulten's group would have a certain amount of time to solve the protein structure with Michel's diffraction data.
When Hartmut Michel's group began work on crystallizing LH2, another researcher at the University of Glasgow began similar determination of the structure of LH2 as well, but for a different bacterium. The British scientist, Richard Cogdell, worked on structure determination for the species Rhodopseudomonas acidophila. On the other hand, Hartmut Michel had chosen to work on LH2 of the bacterium Rhodospirillum molischianum, a decision that would have profound consequences.
Since this was such a tricky problem to solve, Schulten put a big group of people on it: three graduate students and a new post-doc, chemist Xiche Hu. Over the course of several months, as the intricacy and complexity of the problem grew and grew, everyone would drop out of the project except Xiche Hu, the others daunted by what was known as the phase problem.
When diffraction data are generated from a crystal, only two pieces of data can be extracted from the experiment. The third piece of data, missing but necessary for elucidating the structure, is the phase angles of the reflections. One way to solve this is to grow the crystal with heavy metals that are bound to particular places in the protein and through geometrical constructions determine the phase. But the protein Rs. molischianum was so modular that the experimentalists in Michel's group could not co-crystallize with heavy metals successfully. So it fell to Xiche Hu and Klaus Schulten alone to come up with a way to conquer the phase problem.
For Schulten and Hu, two blows would soon befall them as Hu worked diligently on the phase problem. First, they learned that Richard Cogdell and his collaborators succeeded in solving the structure of Rps. acidophila, and would soon publish in Nature . The light-harvesting protein that Hu and Schulten were working on turned out to be the more difficult one to solve. And second, since the allotted months had passed and Hu did not have a structure, the problem reverted back to Hartmut Michel's group. Although this relinquishment was a blow to the scientists in Urbana, Xiche Hu was philosophical about it. “In some sense they had the right to do it,” Hu remarks, “because in principle they didn't need us to solve the structure.”
Although Xiche Hu had to teach himself the entire field of crystallography to work on the light-harvesting complex, he had learned quite a few things during his limited time working with the diffraction data. So when Hartmut Michel was unable to solve the LH2 structure, he approached Schulten to rejoin the project. Xiche Hu was ready to revisit the challenge. “Xiche Hu came every morning to my office,” recalls Schulten, “and put his arm around my shoulder and said, 'Klaus, we are going to make it.' So he, not the postdoctoral advisor, was the confidence generator.”
To conquer the problem, Hu and Schulten first generated a structure that they hoped was similar to the real structure, which they called a “search model,” and which they based on the Rps. acidophila structure that had already been determined by Cogdell's group. The experimentalists in Michel's group were experts in reading diffraction data, and surmised that the structure of the light-harvesting complex was a ring made of eight identical subunits, which effectively reduced the problem to one-eighth of its original complexity. So Hu and Schulten only needed to solve the structure for one subunit in the protein, and they could utilize Cogdell's structure as a guide. In essence Hu and Schulten used a computational step to generate search models that were homologous to the unknown structure so they could determine the phases. Schulten likens the procedure to iterating with Newton's Method: if the answer diverges, one can clearly see the initial guess was far off and so one starts over with a different guess. Hu says that what finally solved the structure was exploiting the symmetry aspects of the protein. The structure they computed is shown at left.

Schulten calls the moment when he saw the structure of the light-harvesting protein one of the peak highlights of his entire career. He realized the significance of this work immediately. His reaction, however, was not quite the same as his competitor, Richard Cogdell's. “So Cogdell tells me, every time I see him: Oh Klaus, I'll never forget when I saw the structure at first, I was just weeping,” recounts Schulten. Although the two teams were racing to solve their respective structure first, the team headed by Michel and Schulten published about a year later than Cogdell. But this publication, in May of 1996 in the journal Structure, remains one of Schulten's most highly cited papers, with 821 citations as of July 2012.
Confrontation in Spain
Around 1995 Schulten went to Spain for a meeting, and gave a talk about the computational methodology Xiche Hu and Schulten were using to approach the problem of solving the structure. Basically Schulten was talking about the role the theorist could play in X-ray crystallography, a field usually reserved for experimentalists. In the audience was crystallographer Robert Huber, who had won the Nobel Prize in 1988 for his role in determining the structure of the reaction center protein. “Huber shot up afterwards, after my lecture,” recounts Schulten, “and said this is the greatest rubbish he ever heard, totally undoable and really stupid.”
Schulten thought this was way too aggressive, and he was not the only one. “There was a coffee break,” continues Schulten, “and after the coffee break, several colleagues stood up and they said: We have to comment on this, because the exchange you heard is making a very bad example for our young people.” These scientists basically conveyed that to not try new approaches, like the one Schulten was advocating, is exactly against the fabric of science. They were not dissuaded by the fact that Huber was a Nobel laureate.
Huber was privy to the whole exchange that followed the coffee break. He eventually stood up and apologized. “He said he would only be too happy if theory would replace experiment,” Schulten recalls. But Schulten recognized that Huber did not actually mean it. This whole incident was only one of many factors that contributed to Schulten's doubts about the success of the project to determine the light-harvesting protein's structure. In the end, however, his doubts were put to rest. And the structure determination was only the beginning of one of the most impressive projects ever achieved in his group.
From Structural Biology to Quantum Physics
While he was studying for his diploma at the University of Frankfurt in Germany in the early 1990s, Thorsten Ritz heard Klaus Schulten give a seminar there about a possible mechanism for the magnetic compass in birds. Captivated by the talk, since it pointed out one of the ways in which quantum mechanics plays a role in biology, Ritz made sure to talk to Schulten while he was briefly doing research at the Center for Complex Systems Research in Urbana. Ritz decided to do dissertation research with Schulten in Urbana while getting his PhD from the University of Ulm. As Schulten did not have a candidate molecule yet for the avian magnetic compass, Ritz began his studies on photosynthesis instead.
In a similar vein, Ana Damjanović was an undergraduate student in physics at Belgrade University when she met Schulten at a conference in Europe. Impressed by his research, she went to the University of Illinois at Urbana-Champaign specifically to get her PhD in Schulten's group. Shortly after her arrival, she would team up with Ritz and begin work on the quantum physics of photosynthesis.
One of the first things the trio would immediately uncover was how quantum coherence assists light harvesting. In the two light-harvesting proteins, LH1 and LH2, chlorophyll-like molecules (technically called bacteriochlorophylls in purple bacteria) are packed closely together in a ring shape, as seen in the picture of LH2 for example. To transfer the energy harvested by the chlorophylls, the individual chlorophylls team up and transfer the excitation not randomly but in a pool. They share their excitation in a very ordered, or so to speak, “coherent” way; it is as though they are humming one tune together as opposed to each playing unique parts in an orchestra. With quantum coherence, the system of pigments could reach very far and fast to transfer the excitation.
In the 1940s Arnold and Oppenheimer had wondered why so many chlorophylls were necessary in photosynthesis and exactly how they all worked together. In the late 1990s, Schulten together with Hu, Ritz and Damjanović elucidated the physics underlying the structures of the light-harvesting proteins, namely that the arrangement of a group of tightly-interacting chlorophylls in fact act together to make themselves more efficient through quantum coherence.
Return to Polyenes
The light-harvesting pigments are not only made of chlorophyll-like molecules that absorb sunlight. They also consist of carotenoids, which are pigments that also absorb light, usually in the blue range, and are popularly known for their nutritional value in foods such as tomatoes and sweet potatoes. The other main highlight of the collaboration between Damjanović and Ritz was clarification of the role that carotenoids play in light harvesting. Basically they found that the carotenoids have to use tricks in order to transfer the excitation to the chlorophylls.
In this study of carotenoids, a trip to Japan for an unrelated reason rekindled Schulten's interest in his Harvard work from the early 1970s on polyenes. Carotenoids are related to polyenes, as both share an underlying structure of conjugated double bonds, and both polyenes and carotenoids have low-lying, optically forbidden states. While Schulten was in Japan in the late 1990s for a conference on visual receptors, he met Yasushi Koyama, an experimentalist who had studied the forbidden states in polyenes. Koyama was keen to collaborate with Schulten and, in order to get to know him better, asked Schulten if he would like to take a drive to an earthquake museum. The Kobe earthquake rocked Japan in 1995, and a memorial museum commemorated the devastation.
During the trip to the earthquake museum, Koyama revealed he was aware of the work Schulten and Karplus did in the early 1970s to prove that polyenes had optically forbidden states, and that he was very eager to team up with Schulten's group for a paper. Schulten and Koyama, together with the team of Ritz and Damjanović, elaborated on the mechanism that made carotenoids sometimes couple efficiently to chlorophylls to transfer excitation when the coupling was expected to be poor. They suggested in a 2000 paper that some photosynthetic systems have a type of symmetry breaking in their carotenoids that augments the excitation transfer efficiency.

This period of time in Schulten's group, from about 1995 to 2000, still stands out as one of the most impressive eras of achievement in Schulten's mind. It started with Xiche Hu and his relentless efforts to solve the structure of LH2, and then ended with Thorsten Ritz and Ana Damjanović. The pair basically sorted out the key physical characteristics that nature had designed to effectively absorb sunlight, to keep it for a short while and transfer it effectively to other pigment subsystems in the so-called photosynthetic light harvesting system.
When asked why the team of Hu, Ritz, and Damjanović was able to unravel the physics behind light-harvesting in such a compact amount of time, Damjanović points to three reasons: the team worked extremely hard, she and Thorsten Ritz helped each other cooperatively, and when stuck, Damjanović took full advantage of the many resources available. “There was usually someone around who knew how to use the software or programs that I needed to use,” Damjanović clarifies, “If I got stuck somewhere, I would go and ask them for help.” This way Damjanović avoided gridlock in the research, by promptly seeking help from scientists at the University of Illinois, and sometimes even researchers at other institutions.
Schulten also weighed in on why the team of Ritz and Damjanović made remarkable achievements. Xiche Hu's work on the light-harvesting protein was the first time in Schulten's group that someone had worked on structural biology. “This structure determination was very new for us; but particularly relative to the structural biology, we were really excellent quantum biologists,” notes Schulten. This second hat that the team wore, of quantum physicists, was a natural one for Schulten's group to embrace since they could draw on years of experience, and this resulted in explanation of the quantum physics behind light harvesting in photosynthesis in the near-record time of about three years.
From Molecule to Cellular Compartment

When Schulten moved to Illinois in 1988 he eventually met two scientists there at the university who also worked on photosynthesis: Antony Crofts and Colin Wraight, both from the School of Molecular and Cellular Biology. Schulten even published a photosynthesis paper in 1999 with Crofts. When Colin Wraight invited Schulten to a dinner in honor of a visitor from Sheffield, Schulten and the guest from England struck up a conversation about their respective work in photosynthesis. Neil Hunter, an experimentalist, was at that point in time imaging a structure called a chromatophore that was central to photosynthetic function in purple bacteria. Always on the lookout for collaborators, Schulten and Hunter decided to join forces to model the chromatophore.
Melih Sener, with a PhD in theoretical high-energy physics, started working on modeling the chromatophore in 2005. While high-energy physics and biophysics may seem like disparate fields, Sener had a clear objective for making the switch. “It's a little bit like the history of Don Quixote,” Sener explains. “He read knightly stories and attacked windmills. And I read the stories of all the heroes of nineteenth- and twentieth-century physics and when I realized that most windmills were already overtaken in high-energy physics, I decided that the most interesting problems are then in biological physics.”
A chromatophore is a complete cellular compartment that's part of a bacterial photosynthetic membrane. “It's essentially a machine that captures sunlight and converts it to chemically stored energy in the form of ATP, and it does so by a number of cooperating proteins,” Sener says. Similar to an organelle in eukaryotes, in bacteria this chromatophore is termed a pseudo-organelle. The chromatophore that Sener began working on is found in the purple bacterium Rhodobacter sphaeroides, contains several hundred proteins, and has a spherical shape that is in vesicle format, although not all chromatophores are spherical. One of the main tasks that Sener faced in the modeling project he light-heartedly compares to the forensics of road kill.
“The way these things are imaged is that you take the vesicle, and then squeeze it flat on a mica surface. Then you can scan it with an atomic force microscope,” explains Sener. With projection algorithms borrowed from geography, Sener deployed computing tools on a flattened patch to reconstruct what it might have looked like when spherical. In fact, Sener utilized experimental data from many sources, not only atomic force microscopy, to reconstruct the chromatophore. He cites as a major challenge the accrual and utilization of decades of experimental data. He culled from X-ray crystallography, NMR, electron microscopy, spectroscopic data, and, of course, the atomic force microscopy images from Neil Hunter's lab. Creation of the model, as shown above, is still an ongoing project for Sener in Schulten's group. For example, missing components need to be filled in, but experimental collaborators are working on some of these issues. To the best of his knowledge, however, this is most likely the first attempt to combine so much data into one structural, organelle-like model.
But this generation of the three-dimensional blueprint of the chromatophore was not the only goal. Sener removed the cap of the forensic scientist and replaced it with the cap of the physicist. His objective: use the tools of quantum physics to model light harvesting for the entire chromatophore. This stage, as well, presented multi-layered challenges to Schulten and Sener. One of them was the separation of scales, time scales as well as length scales. They wanted to describe the many processes involved in converting absorbed energy to ATP, but each step involved different time scales. For example, the first processes of light absorption and excitation transfer are femtoseconds to picoseconds, while the later processes of chemical transformations climb toward microseconds and milliseconds. Length scales also varied from describing electronic states distributed over a single molecule to as large as over the entire vesicle, 50 to 70 nanometers. “So naturally,” summarizes Sener, “you can't use one formulation, one language, one computational framework” when working with disparate scales.
The overall goal of this second stage of the chromatophore project is to compute the rate of ATP production as a function of incoming light intensity. “This required us to look at the chromatophore as a complete machine, where multiple processes communicate with one another,” Sener remarks. “And that's probably going to keep us busy for some time.”
Membrane Curvature
While Sener and his many experimental collaborators were keeping busy modeling the chromatophore, Schulten put other group members on the project of self-assembly of photosynthetic membranes. As shown in the graphic above, the chromatophore consists of light-harvesting proteins (LH2 in green, and LH1 in blue with the reaction center in red) among its many components. Schulten had the idea that these light-harvesting proteins, which have a functional role within the chromatophore, may also help to shape the structure itself as the proteins of the chromatophore aggregate to form membrane indentations.
With papers published in 2008 and 2009, molecular dynamics calculations on three different bacteria showed that aggregates of LH2 complexes do seem to induce curvature, which is consistent with the curved chromatophore membrane structures seen in certain kinds or purple bacteria. These various calculations encompassed between 880,000 and 1.7 million atoms. The scientists who worked on the curvature properties in these two publications include Danielle Chandler and Jen Hsin.
While Hsin and Chandler were pursuing the study of membrane curvature in 2008, a string of lucky coincidences occurred that would enable Hsin to elaborate what is known as the core complex in atomic detail. A burgeoning method in Schulten's group and some new experimental data materialized at exactly the right moment to further knowledge of the core complex.

Klaus Schulten's group has many areas of study, including software development as well as various fields of biophysics, such as magnetoreception, bionanotechnology, and of course, photosynthesis. But in 2005 he agreed to collaborate on a project with an experimentalist that promised a fascinating molecular machine to work with, and that would be a great test for the methodology and techniques his group has developed over the years to examine massive systems. What he and his students needed to do was combine data from X-ray crystallography with lower resolution data from the electron microscope, and the system in question was the cell's protein making factory: the ribosome. Essentially, to use an analogy, consider trying to understand a bird in flight. The X-ray crystallography data captures a crisp picture of a bird standing still on a wire, with the position of every claw, feather, and eye well known. But the electron microscopy image of a flying bird is more fuzzy, although captures the bird in the course of its function. What Schulten needed to do was apply all the crisp details of the motionless bird to the image of the bird in flight, therefore making it possible to explain flight, or the bird's function.
All the elements to coax the X-ray data into the electron microscopy image of the ribosome were at hand in Schulten's group; in fact his career was ready for it by 2006. He had in-house software, VMD and NAMD, which group members could exploit and alter, he had a mixture of software developers and research scientists who could offer support and expertise, he had a thirty-year history of working with experimentalists, his group at the Beckman Institute was a well-oiled machine after 20+ years, and he had energetic and talented graduate students who worked tirelessly on the ribosome. So, in a matter of about two years, his group had developed a method they introduced to the world in 2008, called Molecular Dynamics Flexible Fitting (MDFF). A detailed account of its creation and subsequent numerous applications can be found in this history of MDFF .
Jen Hsin had been studying the core complex, which, as seen in the image, is comprised of two reaction centers in green with light-harvesting complex I (LH1) wrapped around it in blue. With help from fellow graduate student James Gumbart, Hsin built a model of the core complex, which is considered a dimer since it has two distinct but similar regions. She used bits and pieces of proteins to make an atomic-level structure of the dimer. And when she ran simulations of the dimer in a patch of membrane, the system wouldn't stay flat, an effect seen in the bottom frame of the image. “We were puzzled by this result, as such geometry was unusual for integral membrane proteins,” recalls Hsin. “Until our collaborators informed us that they had just obtained a low-resolution electron microscopy map of the same protein complex showing that it is indeed bent, and even more drastically than we saw in the simulation.” The experimentalist who provided the electron microscopy data was Neil Hunter.
To further their understanding about how the large degree of bending they were seeing, now on two fronts, would affect membrane geometry, the task at hand required merging the atomistic models and simulations of Hsin's with the low-resolution map from Neil Hunter. At this time, the method Molecular Dynamics Flexible Fitting had just been developed in the Schulten group, which was exactly the method needed to merge atomic-level structures into less-resolved electron microscopy images. Coincidentally, all the pieces of the puzzle came together so perfectly to further the study of membrane curvature with MDFF. Hsin explains that the MDFF work, published in 2009, showed that “higher order structures of large, transmembrane protein complexes can force their membrane environment to adopt to their geometries.”
Membrane curvature research is still ongoing. Currently Danielle Chandler is continuing its study, with massive calculations that are pushing the limits of the simulation and visualization software produced in Schulten's group, namely NAMD and VMD. Her simulations encompass 20 million atoms, probably one of the largest molecular dynamics calculations in the world on a biological system to date. Membrane curvature is not the only physical property of study. “We're also interested in things like protein-lipid interactions, lipid mobility, etc.,” Chandler says.
The purple bacterium in question is Rhodospirillum photometricum , chosen because of the availability of atomic force microscopy data on the structure. But all this data presents major challenges for the researcher. One of the steps of this molecular dynamics simulation involves gathering coordinates for 20 million atoms, but the software VMD can only tackle a few million atoms. “John Stone, the developer of VMD in Schulten's group, had to invent a new file format to handle it,” continues Chandler. “The new format is a binary file formatso you can't open it in a text editor to look at it, but it can handle an arbitrarily large amount of data.” While the coordinates of Rsp. photometricum take up approximately 1.1 gigabytes, a trajectory file from a molecular dynamics run is about 4 terabytes large, and has to be stored on external hard drives. This calculation is already running on the Blue Waters supercomputer located at the University of Illinois at the National Center for Supercomputing Applications (NCSA). Completion of these massive computations will only bring the Schulten group closer to its goal of predicting the behavior of entire life forms from just their constituent atoms.
The Role of Quantum Coherence, Revisited
The final stage in this history of photosynthesis ends with a look at what the group at Beckman is doing most recently on quantum coherence in photosynthetic purple bacteria. In 1999 Schulten and his graduate students Ana Damjanović and Thorsten Ritz investigated how quantum coherence manifests in excitation transfer in the purple bacteria; at the close of this first decade of the new millennium Schulten returned to this topic, but in a much more comprehensive and elegant manner.

Coherence, which is a quantum phenomenon mentioned above and first described in the work of Xiche Hu, Thorsten Ritz and Ana Damjanović, means that two things, like molecules in a pigment ring, are correlated. “So coherence is like taking two things and making them almost one,” Strümpfer explains, “where one directly affects the other one instantaneously. There's no time delay, there's no exchange of anything, it's just an instantaneous thing.” For example, chlorophylls in a ring of a light-harvesting protein are involved in sharing excitation. “When chlorophylls share the excitation between them,” Schulten further clarifies, “it's not like the excitation hops from one chlorophyll to the next and then totally forgets where it comes from. Rather it remembers where it comes from.” This is one manifestation of coherence in photosynthesis.
One of the things Strümpfer had to account for was the thermal environment. Very often physicists like to do their calculations at absolute zero to reduce the complexity of a difficult problem. But Strümpfer did not have that luxury with living systems. “You have to work at room temperature, or the temperatures that these organisms experience,” Strümpfer explains. “And consider everything in place there: the membrane, the water, and the proteins surrounding everything. You know that those components are fluctuating with thermal noise basically.”
While taking into account all the molecules in a protein, and the lipid membrane it is attached to, and the water that surrounds it, may seem like a daunting task, Strümpfer and Schulten used clever mathematics to circumvent the problem of including those millions of atoms in the surrounding environment. The scientists again turned to Sir Anthony Leggett, who has studied quantum mechanics in a thermal environment. The elements of the surroundings, like water molecules or the lipids of a membrane, vibrate with a certain set of frequencies; Strümpfer used a distribution of frequencies that reflected the surrounding pieces and coupled the pigments he was studying to those frequencies. Strümpfer and Schulten basically took the ideas of Leggett and adapted them to their particular problem.
While Strümpfer had successfully unearthed a theory to explicate excitation transfer, implementation presented immediate challenges. Not only was the new theory hard to understand, writing code that ran fast enough was a hurdle. Strümpfer discovered that he had to educate himself very quickly about computer hardware and the nuances of writing efficient C++ code. This calculation required huge amounts of RAM, 200 gigabytes, and only one computer in the group met those conditions; but getting his code to take advantage of the huge memory was not straightforward. “I had to scramble and ask people all over the place how to do this. I needed to get it faster. I couldn't do the calculation unless I could get it done quickly.” Strümpfer eventually got his code working.
The graphic above shows a small segment of a photosynthetic apparatus, which includes a reaction center that is surrounded by an LH1 ring, and close by are three LH2 rings. The LH1 ring basically acts as a system of antennae, feeding excitation to the reaction center. But in the dark habitats that these purple bacteria live infor example, in the bottom of pondsmore feeder systems evolved, namely LH2s, to channel additional excitation energy to the LH1 and then to the reaction center. In the work Strümpfer and Schulten did on excitation transfer, they looked at transfer in two distinct forms: how excitation travels within a ring, and how excitation travels from one ring to another. And they found some surprising results.

In considering how excitation moves within a ring, say within an LH1 ring or within one LH2 ring, Strümpfer looked at exciting just one pigment molecule individually in a ring and then observed how it settled. “Suppose excitation starts at one place,” spells out Strümpfer, “and then while this is still excited, another one also becomes excited. You have both of them excited at the same time, and then if it's very strongly coupled, then they're coherently excited. So then they act as one unit now, instead of being two separate things.” Strümpfer goes on to note that the description he and Schulten like for this inter-ring coherent behavior is to consider it as waves sloshing back and forth, or running around in the ring. “This is something that I struggled with immensely, actually,” admits Strümpfer, “because we don't have things that behave like this in our reality, if you can imagine.”
Strümpfer and Schulten found that within a ring complex, coherence plays an important role; but the hopping from one ring to another, for example from one LH2 to a different LH2, is much easier to visualize and compute. Excitation hops from one ring to another, and its mathematics is given by probabilities, but the excitation is never in both rings at the same time. “So that makes it easier, first, to imagine, and then second, to actually model physically,” remarks Strümpfer.
One of the major questions people have asked Schulten and Strümpfer about this work is how does quantum coherence then play a role in light harvesting. And Strümpfer calls the answer one of the most surprising findings to come out of their comprehensive study. “The effect is that having quantum coherence within one ring dramatically improves the rate at which excitation can jump from one ring to the next ring,” he summarizes.
Schulten is proud of his group's efforts on coherence, which started in the late 1990s and continues on today with the work of Johan Strümpfer. But this current work on coherence draws from some 30-plus years of Schulten and collaborators laboring to understand the story of how nature has fashioned photosynthesis to make it an efficient and elegant process. And Schulten's objective still remains to uncover the many steps of photosynthesis and clearly explain the findings to the research community. “There was a lot of work done, methodological developments and theory and so on, but the goal was to explain how nature effectively harvests sunlight,” summarizes Schulten on his last 35 years. “It's maybe still not complete, but I figured it out to a large degree.” And without a doubt, for what remains to be done, Schulten's group will be at the forefront in the years to come.
After forty-plus years of studying photosynthesis, Klaus Schulten has pieced together the intervening processes that occur between the absorption of sunlight and the generation of ATP. He feels he has finally garnered an understanding to suit himself, about the full mechanism of the photosynthetic membrane. With solution of the entire structure of the chromatophore at hand, Schulten was at a point in his career where he could illustrate the function of this autonomous machine, which harbors many processes that interlock together to make photosynthesis work. He had also demystified the role of each type of protein that comprises the chromatophore. At this juncture in time (2014), he harkened back to his long-cherished goal of explaining a whole cell, and realized he could by now reach this goal, by at least explicating a living system at the organelle level. He could show the function of chromatophore and how its proteins worked together like a Swiss watch to convert sunlight into ATP. But what he wanted to do scientifically he could only do with a movie.
This is no Hollywood creation, however, with its myriad special effects and artists' renditions. Schulten had control of all 100 million atoms that make up the chromatophore, and each interlocking process illustrated is based on sound research conducted over decades by many scientists, including various members of Schulten's group. The movie starts with light harvesting and quantum coherence, proceeds to electron and proton transfer, and ends up with synthesis of ATP. The proteins critical to this array of processes are the reaction center, LH1 and LH2, the bc 1 complex, and finally ATP synthase. Within the context of the chromatophore, the movie accounts for all the interacting parts for an entire, multi-faceted description of a full biological machine. What is illustrated is the interdigitation of processes going on, not just one process or one protein, as is often how scientists have to study living systems.
This movie is a culmination of a life's career in the field of photosynthesis, based on over forty years of work. It also illustrates a driving principle for Klaus Schulten, the desire to understand biological organization, or how multiple proteins form societies, or associations and collaborations, and thereby make a cell become "alive." The movie indeed looks at one such society with over one hundred proteins enclosed in a membrane, encompassing roughly 100 million atoms. Get ready to see how this biological machine functions, based on decades of work at the level of atoms and molecules.
Photosynthesis Movie
The Photosynthetic Membrane of Purple Bacteria: A Clockwork of Proteins and Processes from TCBG on Vimeo .
Other Related Articles
Stephen Jay Gould. Planet of the Bacteria. Washington Post Horizon, 119:344, 1996.
Martin Karplus. Spinach on the Ceiling: A Theoretical Chemist's Return to Biology. The Annual Review of Biophysics and Biomolecular Structure, 35:1-47, 2006.
Klaus Schulten and Martin Karplus. On the origin of a low-lying forbidden transition in polyenes and related molecules. Chemical Physics Letters, 14:305-309, 1972.
Klaus Schulten, H. Staerk, Albert Weller, Hans-Joachim Werner, and B. Nickel. Magnetic field dependence of the geminate recombination of radical ion pairs in polar solvents. Zeitschrift für Physikalische Chemie , NF101:371-390, 1976.
Hans-Joachim Werner, Zan Schulten, and Klaus Schulten. Theory of the magnetic field modulated geminate recombination of radical ion pairs in polar solvents: Application to the pyrene-N,N-dimethylaniline system. Journal of Chemical Physics, 67:646-663, 1977.
Zan Schulten and Klaus Schulten. The generation, diffusion, spin motion, and recombination of radical pairs in solution in the nanosecond time domain. Journal of Chemical Physics, 66:4616-4634, 1977.
Hans-Joachim Werner, Klaus Schulten, and Albert Weller. Electron transfer and spin exchange contributing to the magnetic field dependence of the primary photochemical reaction of bacterial photosynthesis. Biochimica et Biophysica Acta, 502:255-268, 1978.
Web of Stories. “Manfred Eigen, 108, The winter seminars.” Accessed April 12, 2012. http://www.webofstories.com/play/52329?o=MS
Web of Stories. “Manfred Eigen, 109, Why the winter seminars are unique.” Accessed April 12, 2012. http://www.webofstories.com/play/52330
Theodor Förster. Zwischenmolekulare Energiewanderung und Fluoreszenz. Ann. Phys. (Leipzig). 2:55-75, 1948.
J. R. Oppenheimer. Internal Conversion in Photosynthesis. Phys. Rev., 60:158, 1941.
William Arnold and J. R. Oppenheimer. Internal conversions in the photosynthetic mechanism of blue-green algae. J. Gen. Physiol., 33:423-435, 1950.
William A. Arnold. Personal Perspective: Experiments. Photosynthesis Research, 27:73-82, 1991.
Nobelprize.org. “Hartmut Michel - Autobiography.” Accessed April 13, 2012. http://www.nobelprize.org/nobel_prizes/chemistry/laureates/1988/michel-autobio.html
Nobelprize.org. “Johann Deisenhofer - Autobiography.” Accessed April 12, 2012, http://www.nobelprize.org/nobel_prizes/chemistry/ laureates/1988/deisenhofer-autobio.html
Nobelprize.org. “Video Player: Interview with Johann Deisenhofer,” Accessed April 12, 2012, http://www.nobelprize.org/mediaplayer/index.php?id=893&view=1
Nobelprize.org. “Video Player: Interview with Hartmut Michel,” Accessed April 13, 2012, http://www.nobelprize.org/mediaplayer/index.php?id=413&view=1
Johann Deisenhofer and Hartmut Michel. The photosynthetic reaction centre from the purple bacterium Rhodopseudomonas viridis (Nobel Lecture). Angew. Chem. Int. Engl., 28:829-847, 1989.
Johann Deisenhofer and Hartmut Michel. The photosynthetic reaction centre from the purple bacterium Rhodopseudomonas viridis. Science, 245:1463-1473, 1989.
Herbert Treutlein, Christoph Niedermeier, Klaus Schulten, J. Deisenhofer, H. Michel, Axel Brünger, and Martin Karplus. Molecular dynamics simulation of the primary processes in the Photosynthetic reaction center of Rhodopseudomonas viridis. In A. Pullman et al., editors, Transport Through Membranes: Carriers, Channels and Pumps, pp. 513-525. Kluwer Academic Publishers, Dordrecht, 1988.
Herbert Treutlein, Klaus Schulten, Christoph Niedermeier, J. Deisenhofer, H. Michel, and D. Devault. Electrostatic control of electron transfer in the photosynthetic reaction center of Rhodopseudomonas viridis. In J. Breton and A. Verméglio, editors, The Photosynthetic Bacterial Reaction Center: Structure and Dynamics, volume 149 of NATO Science Series A: Life Sciences, pp. 369-377. Plenum, New York, 1988.
Dong Xu and Klaus Schulten. Coupling of protein motion to electron transfer in a photosynthetic reaction center: Investigating the low temperature behaviour in the framework of the spin-boson model. Chemical Physics, 182:91-117, 1994.
Juergen Koepke, Xiche Hu, Cornelia Muenke, Klaus Schulten, and Hartmut Michel. The crystal structure of the light harvesting complex II (B800-850) from Rhodospirillum molischianum. Structure, 4:581-597, 1996.
Ana Damjanović, Thorsten Ritz, and Klaus Schulten. Energy transfer between carotenoids and bacteriochlorophylls in a light harvesting protein. Physical Review E, 59:3293-3311, 1999.
Thorsten Ritz, Ana Damjanović, Klaus Schulten, Jian-Ping Zhang, and Yasushi Koyama. Efficient light harvesting through carotenoids. Photosynthesis Research, 66:125-144, 2000.
Melih K. Sener, John D. Olsen, C. Neil Hunter, and Klaus Schulten. Atomic level structural and functional model of a bacterial photosynthetic membrane vesicle. Proceedings of the National Academy of Sciences, USA, 104:15723-15728, 2007.
Danielle Chandler, Jen Hsin, Christopher B. Harrison, James Gumbart, and Klaus Schulten. Intrinsic curvature properties of photosynthetic proteins in chromatophores. Biophysical Journal, 95:2822-2836, 2008.
Jen Hsin, Danielle E. Chandler, James Gumbart, Christopher B. Harrison, Melih Sener, Johan Strümpfer, and Klaus Schulten. Self-assembly of photosynthetic membranes. ChemPhysChem, 11:1154-1159, 2010.
Jen Hsin, James Gumbart, Leonardo G. Trabuco, Elizabeth Villa, Pu Qian, C. Neil Hunter, and Klaus Schulten. Protein-induced membrane curvature investigated through molecular dynamics flexible fitting. Biophys. J. , 97:321-329, 2009.
Johan Strümpfer and Klaus Schulten. Light harvesting complex II B850 excitation dynamics. Journal of Chemical Physics, 131:225101, 2009.
Johan Strümpfer, Melih Sener, and Klaus Schulten. How quantum coherence assists photosynthetic light harvesting. Journal of Physical Chemistry Letters, 3:536-542, 2012.
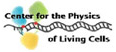

An official website of the United States government
The .gov means it’s official. Federal government websites often end in .gov or .mil. Before sharing sensitive information, make sure you’re on a federal government site.
The site is secure. The https:// ensures that you are connecting to the official website and that any information you provide is encrypted and transmitted securely.
- Publications
- Account settings
Preview improvements coming to the PMC website in October 2024. Learn More or Try it out now .
- Advanced Search
- Journal List
- Plant Physiol
- v.154(2); 2010 Oct

Early Evolution of Photosynthesis 1
Photosynthesis is the only significant solar energy storage process on Earth and is the source of all of our food and most of our energy resources. An understanding of the origin and evolution of photosynthesis is therefore of substantial interest, as it may help to explain inefficiencies in the process and point the way to attempts to improve various aspects for agricultural and energy applications.
A wealth of evidence indicates that photosynthesis is an ancient process that originated not long after the origin of life and has evolved via a complex path to produce the distribution of types of photosynthetic organisms and metabolisms that are found today ( Blankenship, 2002 ; Björn and Govindjee, 2009 ). Figure 1 shows an evolutionary tree of life based on small-subunit rRNA analysis. Of the three domains of life, Bacteria, Archaea, and Eukarya, chlorophyll-based photosynthesis has only been found in the bacterial and eukaryotic domains. The ability to do photosynthesis is widely distributed throughout the bacterial domain in six different phyla, with no apparent pattern of evolution. Photosynthetic phyla include the cyanobacteria, proteobacteria (purple bacteria), green sulfur bacteria (GSB), firmicutes (heliobacteria), filamentous anoxygenic phototrophs (FAPs, also often called the green nonsulfur bacteria), and acidobacteria ( Raymond, 2008 ). In some cases (cyanobacteria and GSB), essentially all members of the phylum are phototrop2hic, while in the others, in particular the proteobacteria, the vast majority of species are not phototrophic.

Small subunit rRNA evolutionary tree of life. Taxa that contain photosynthetic representatives are highlighted in color, with green highlighting indicating a type I RC, while purple highlighting indicates a type II RC. The red arrow indicates the endosymbiotic event that formed eukaryotic chloroplasts. Tree adapted from Pace (1997) .
Overwhelming evidence indicates that eukaryotic photosynthesis originated from endosymbiosis of cyanobacterial-like organisms, which ultimately became chloroplasts ( Margulis, 1992 ). So the evolutionary origin of photosynthesis is to be found in the bacterial domain. Significant evidence indicates that the current distribution of photosynthesis in bacteria is the result of substantial amounts of horizontal gene transfer, which has shuffled the genetic information that codes for various parts of the photosynthetic apparatus, so that no one simple branching diagram can accurately represent the evolution of photosynthesis ( Raymond et al., 2002 ). However, there are some patterns that can be discerned from detailed analysis of the various parts of the photosynthetic apparatus, so some conclusions can be drawn. In addition, the recent explosive growth of available genomic data on all types of photosynthetic organisms promises to permit substantially more progress in unraveling this complex evolutionary process.
While we often talk about the evolution of photosynthesis as if it were a concerted process, it is more useful to consider the evolution of various photosynthetic subsystems, which have clearly had distinct evolutionary trajectories. In this brief review we will discuss the evolution of photosynthetic pigments, reaction centers (RCs), light-harvesting (LH) antenna systems, electron transport pathways, and carbon fixation pathways. These subsystems clearly interact with each other, for example both the RCs and antenna systems utilize pigments, and the electron transport chains interact with both the RCs and the carbon fixation pathways. However, to a significant degree they can be considered as modules that can be analyzed individually.
ORIGINS OF PHOTOSYNTHESIS
We know very little about the earliest origins of photosynthesis. There have been numerous suggestions as to where and how the process originated, but there is no direct evidence to support any of the possible origins ( Olson and Blankenship, 2004 ). There is suggestive evidence that photosynthetic organisms were present approximately 3.2 to 3.5 billion years ago, in the form of stromatolites, layered structures similar to forms that are produced by some modern cyanobacteria, as well as numerous microfossils that have been interpreted as arising from phototrophs ( Des Marais, 2000 ). In all these cases, phototrophs are not certain to have been the source of the fossils, but are inferred from the morphology or geological context. There is also isotopic evidence for autotrophic carbon fixation at 3.7 to 3.8 billion years ago, although there is nothing that indicates that these organisms were photosynthetic. All of these claims for early photosynthesis are highly controversial and have engendered a great deal of spirited discussion in the literature ( Buick, 2008 ). Evidence for the timing of the origin of oxygenic photosynthesis and the rise of oxygen in the atmosphere is discussed below. The accumulated evidence suggests that photosynthesis began early in Earth’s history, but was probably not one of the earliest metabolisms and that the earliest forms of photosynthesis were anoxygenic, with oxygenic forms arising significantly later.
PHOTOSYNTHETIC PIGMENTS
Chlorophylls are essential pigments for all phototrophic organisms. Chlorophylls are themselves the product of a long evolutionary development, and can possibly be used to help understand the evolution of other aspects of photosynthesis. Chlorophyll biosynthesis is a complex pathway with 17 or more steps ( Beale, 1999 ). The early part of the pathway is identical to heme biosynthesis in almost all steps and has clearly been recruited from that older pathway. The later steps include the insertion of magnesium and the elaboration of the ring system and its substituents. The earliest version of the pathway (and that used by most modern anoxygenic photosynthetic organisms) almost certainly was anaerobic, both not requiring and not tolerating the presence of O 2 . However, all modern oxygenic photosynthetic organisms now require O 2 as an oxidant at several steps in the pathway. This has been explained in terms of gene replacement of the genes coding for the enzymes at these steps, with the result that the overall pathway is unchanged but the enzymes at key steps are completely different in different groups of phototrophs ( Raymond and Blankenship, 2004 ).
A key concept in using chlorophyll biosynthesis pathways to infer the evolution of photosynthesis is the Granick hypothesis, which states that the biosynthetic pathway of chlorophyll recapitulates the evolutionary sequence ( Granick, 1965 ). This is an appealing idea and probably at least partly true. However, in some cases, in particular the situation of chlorophyll and bacteriochlorophyll, it has been argued that the strict version of the Granick hypothesis is misleading and other interpretations are more likely ( Blankenship, 2002 ; Blankenship et al., 2007 ).
All photosynthetic organisms contain carotenoids, which are essential for photoprotection, usually also function as accessory pigments, and in many cases serve as key regulatory molecules. Carotenoids, unlike chlorophylls, are also found in many other types of organisms, so their evolutionary history may reflect many other functions in addition to photosynthesis ( Sandman, 2009 ).
REACTION CENTERS
The RC complex is at the heart of photosynthesis; so much attention has been paid to understand the evolution of RCs. A wealth of evidence, including structural, spectroscopic, thermodynamic, and molecular sequence analysis, clearly segregates all known RCs into two types of complexes, called type I and type II ( Blankenship, 2002 ). Anoxygenic phototrophs have just one type, either type I or II, while all oxygenic phototrophs have one of each type. The primary distinguishing feature of the two types of RCs are the early electron acceptor cofactors, which are FeS centers in type I RCs and pheophytin/quinone complexes in type II RCs. The distribution of RC types on the tree of life is shown in Figure 1 and a comparative electron transport diagram that compares the different RCs in different types of organisms is shown in Figure 2 , with type I RCs color coded green and type II RCs color coded purple.

Electron transport diagram indicating the types or RCs and electron transport pathways found in different groups of photosynthetic organisms. The color coding is the same as for Figure 1 and highlights the electron acceptor portion of the RC. Figure courtesy of Martin Hohmann-Marriott.
Further analysis strongly suggests that all RCs have evolved from a single common ancestor and have a similar protein and cofactor structure. This is clearly seen when structural overlays of both type I and II RCs are made, showing a remarkably conserved three-dimensional protein and cofactor structure, despite only minimal residual sequence identity ( Sadekar et al., 2006 ). These comparisons have been used to derive structure-based evolutionary trees that do not rely on sequence alignments. Figure 3 shows a schematic evolutionary tree of RCs that is derived from this sort of analysis. It proposes that the earliest RC was intermediate between type I and II (type 1.5) and that multiple gene duplications have given rise to the heterodimeric (two related yet distinct proteins that form the core of the RC) complexes that are found in most modern RCs.

Schematic evolutionary tree showing the development of the different types of RC complexes in different types of photosynthetic organisms. This tree is based on structural comparisons of RCs by Sadekar et al. (2006) . Blue color coding indicates protein homodimer, while red indicates protein heterodimer complexes. Red stars indicate gene duplication events that led to heterodimeric RCs. Helio, Heliobacteria; GSB, green sulfur bacteria; FAP, filamentous anoxygenic phototroph.
A second important issue that relates to RC evolution is the question of how both type I and II RCs came to be in cyanobacteria, while all other photosynthetic prokaryotes have only a single RC. The various proposals that have been made to explain this fact can all be divided into either fusion or selective loss scenarios or variants thereof ( Blankenship et al., 2007 ). In the fusion hypothesis, the two types of RCs develop separately in anoxygenic photosynthetic bacteria and are then brought together by a fusion of two organisms, which subsequently developed the ability to oxidize water. In the selective loss hypothesis, the two types of RCs both evolved in an ancestral organism and then loss of one or the other RC gave rise to the organisms with just one RC, while the ability to oxidize water was added later. Both scenarios have proponents, and it is not yet possible to choose between them.
ELECTRON TRANSPORT CHAINS
The primary photochemistry and several of the early secondary electron transfer reactions take place within the RC complex. However, additional electron transfer processes are necessary before the process of energy storage is complete. These include the cytochrome bc 1 and b 6 f complexes. These complexes oxidize quinols produced by photochemistry in type II RCs or via cyclic processes in type I RCs and pumps protons across the membrane that in turn contribute to the proton motive force that is used to make ATP. All phototrophic organisms have a cytochrome bc 1 or b 6 f complex of generally similar architecture, with the exception of the FAP phylum of anoxygenic phototrophs ( Yanyushin et al., 2005 ). This group contains instead a completely different type of complex that is called alternative complex III. The evolutionary origin of this complex is not yet clear. While the cytochrome bc 1 and b 6 f complexes are similar in many ways, the cytochrome c 1 and f subunits are very different and are almost certainly of distinct evolutionary origin ( Baniulis et al., 2008 ).
ANTENNA SYSTEMS
All photosynthetic organisms contain a light-gathering antenna system, which functions to collect excitations and transfer them to the RC where the excited state energy is used to drive photochemistry ( Green and Parson, 2003 ). While the presence of an antenna is universal, the structure of the antenna complexes and even the types of pigments used in them is remarkably varied in different types of photosynthetic organisms. This very strongly suggests that the antenna complexes have been invented multiple times during the course of evolution to adapt organisms to particular photic environments. So while evolutionary relationships are clear among some categories of antennas, such as the LH1 and LH2 complexes of purple bacteria and the LHCI and LHCII complexes of eukaryotic chloroplasts, it is not possible to relate these broad categories of antennas to each other in any meaningful way. This is in contrast to the RCs, where all available evidence clearly points to a single origin that has subsequently undergone a complex evolutionary development.
CARBON FIXATION PATHWAYS
Most phototrophic organisms are capable of photoautotrophic metabolism, in which inorganic substrates such as water, H 2 S, CO 2 , or HCO 3 − are utilized along with light energy to produce organic carbon compounds and oxidized donor species. However, there are some groups of phototrophs that cannot carry out photoautotrophic metabolism and there are at least three entirely separate autotrophic carbon fixation pathways that are found in different types of organisms ( Thauer, 2007 ). By far the dominant carbon fixation pathway is the Calvin-Benson cycle, which is found in all oxygenic photosynthetic organisms, and also in most purple bacteria. The GSB use the reverse tricarboxylic acid cycle, and many of the FAPs use the 3-hydroxypropionate cycle ( Zarzycki et al., 2009 ). The Gram-positive heliobacteria lack any known autotrophic carbon fixation pathway and usually grow photoheterotrophically ( Asao and Madigan, 2010 ). Similarly, the aerobic anoxygenic phototrophs, which are closely related to the purple bacteria, lack any apparent ability to fix inorganic carbon. In the latter case, it seems most likely that the ancestor of this group contained the Calvin-Benson cycle but lost the genes because of their obligate aerobic lifestyle ( Swingley et al., 2007 ).
The carbon fixation machinery is thus similar to the antennas, in that several entirely separate solutions have been adopted by different classes of phototrophic organisms. This would be consistent with the idea that the earliest phototrophs were photoheterotrophic, using light to assimilate organic carbon, instead of being photoautotrophic. The ability to fix inorganic carbon was then added to the metabolism somewhat later during the course of evolution, possibly borrowing carbon fixation pathways that had developed earlier in autotrophic nonphotosynthetic organisms.
TRANSITION TO OXYGENIC PHOTOSYNTHESIS
Perhaps the most widely discussed yet poorly understood event in the evolution of photosynthesis is the invention of the ability to use water as an electron donor, producing O 2 as a waste product and giving rise to what is now called oxygenic photosynthesis. The production of O 2 and its subsequent accumulation in the atmosphere forever changed the Earth and permitted the development of advanced life that utilized the O 2 during aerobic respiration. Several lines of geochemical evidence indicate that free O 2 began to accumulate in the atmosphere by 2.4 billion years ago, although the ability to do oxygenic photosynthesis probably began somewhat earlier ( Buick, 2008 ). In order for O 2 to accumulate, it is necessary that both the biological machinery needed to produce it has evolved, but also the reduced carbon produced must be buried by geological processes, which are controlled by geological processes such as plate tectonics and the buildup of continents. So the buildup of O 2 in the atmosphere represents a coming together of the biology that gives rise to O 2 production and the geology that permits O 2 to accumulate.
Oxygen is produced by PSII in the oxygen evolving center, which contains a tetranuclear manganese complex. The evolutionary origin of the oxygen evolving center has long been a mystery. Several sources have been suggested, but so far no convincing evidence has been found to resolve this issue ( Raymond and Blankenship, 2008 ). The possibility that functional intermediate stages existed that connect the anoxygenic type II RCs to PSII seems likely ( Blankenship and Hartman, 1998 ).
The process of photosynthesis originated early in Earth’s history, and has evolved to its current mechanistic diversity and phylogenetic distribution by a complex, nonlinear process. Current evidence suggests that the earliest photosynthetic organisms were anoxygenic, that all photosynthetic RCs have been derived from a single source, and that antenna systems and carbon fixation pathways have been invented multiple times.
- Asao M, Madigan MT. (2010) Taxonomy, phylogeny, and ecology of the heliobacteria . Photosynth Res 104 : 103–111 [ PubMed ] [ Google Scholar ]
- Baniulis D, Yamashita E, Zhang H, Hasan SS, Cramer WA. (2008) Structure-function of the cytochrome b 6 f complex . Photochem Photobiol 84 : 1349–1358 [ PubMed ] [ Google Scholar ]
- Beale S. (1999) Enzymes of chlorophyll biosynthesis . Photosynth Res 60 : 43–73 [ Google Scholar ]
- Björn LO, Govindjee (2009) The evolution of photosynthesis and chloroplasts . Curr Sci 96 : 1466–1474 [ Google Scholar ]
- Blankenship RE. (2002) Molecular Mechanisms of Photosynthesis . Blackwell Science, Oxford [ Google Scholar ]
- Blankenship RE, Hartman H. (1998) The origin and evolution of oxygenic photosynthesis . Trends Biochem Sci 23 : 94–97 [ PubMed ] [ Google Scholar ]
- Blankenship RE, Sadekar S, Raymond J. (2007) The evolutionary transition from anoxygenic to oxygenic photosynthesis . Falkowski P, Knoll AN, , Evolution of Aquatic Photoautotrophs . Academic Press, New York, pp 21–35 [ Google Scholar ]
- Buick R. (2008) When did oxygenic photosynthesis evolve? Philos Trans R Soc Lond B Biol Sci 363 : 2731–2743 [ PMC free article ] [ PubMed ] [ Google Scholar ]
- Des Marais DJ. (2000) Evolution: When did photosynthesis emerge on Earth? Science 289 : 1703–1705 [ PubMed ] [ Google Scholar ]
- Granick S. (1965) Evolution of heme and chlorophyll . Bryson G, Vogel HJ, , Evolving Genes and Proteins . Academic Press, New York, pp 67–88 [ Google Scholar ]
- Green BR, Parson WW. (2003) Light-Harvesting Antennas . Kluwer, Dordrecht, The Netherlands [ Google Scholar ]
- Margulis L. (1992) Symbiosis in Cell Evolution . WH Freeman, San Francisco [ Google Scholar ]
- Olson JM, Blankenship RE. (2004) Thinking about the evolution of photosynthesis . Photosynth Res 80 : 373–386 [ PubMed ] [ Google Scholar ]
- Pace NR. (1997) A molecular view of microbial diversity and the biosphere . Science 276 : 734–740 [ PubMed ] [ Google Scholar ]
- Raymond J. (2008) Coloring in the tree of life . Trends Microbiol 16 : 41–43 [ PubMed ] [ Google Scholar ]
- Raymond J, Blankenship RE. (2004) Biosynthetic pathways, gene replacement and the antiquity of life . Geobiology 2 : 199–203 [ Google Scholar ]
- Raymond J, Blankenship RE. (2008) The origin of the oxygen-evolving complex . Coord Chem Rev 252 : 377–383 [ Google Scholar ]
- Raymond J, Zhaxybayeva O, Gerdes S, Gogarten JP, Blankenship RE. (2002) Whole genome analysis of photosynthetic prokaryotes . Science 298 : 1616–1620 [ PubMed ] [ Google Scholar ]
- Sadekar S, Raymond J, Blankenship RE. (2006) Conservation of distantly related membrane proteins: photosynthetic reaction centers share a common structural core . Mol Biol Evol 23 : 2001–2007 [ PubMed ] [ Google Scholar ]
- Sandman G. (2009) Evolution of carotenoid desaturation: the complication of a simple pathway . Arch Biochem Biophys 483 : 169–174 [ PubMed ] [ Google Scholar ]
- Swingley WD, Gholba S, Mastrian SD, Matthies HJ, Hao J, Ramos H, Acharya CR, Conrad AL, Taylor HL, Dejesa LC, et al. (2007) The complete genome sequence of Roseobacter denitrificans reveals a mixotrophic rather than photosynthetic metabolism . J Bacteriol 189 : 683–690 [ PMC free article ] [ PubMed ] [ Google Scholar ]
- Thauer RK. (2007) A fifth pathway of carbon metabolism . Science 318 : 1732–1733 [ PubMed ] [ Google Scholar ]
- Yanyushin MF, del Rosario M, Brune DC, Blankenship RE. (2005) A new class of bacterial membrane oxidoreductases . Biochemistry 44 : 10037–10045 [ PubMed ] [ Google Scholar ]
- Zarzycki J, Brecht V, Muller M, Fuchs G. (2009) Identifying the missing steps of the autotrophic 3-hydroxypropionate CO 2 fixation cycle in Chloroflexus aurantiacus . Proc Natl Acad Sci USA 106 : 21317–21322 [ PMC free article ] [ PubMed ] [ Google Scholar ]
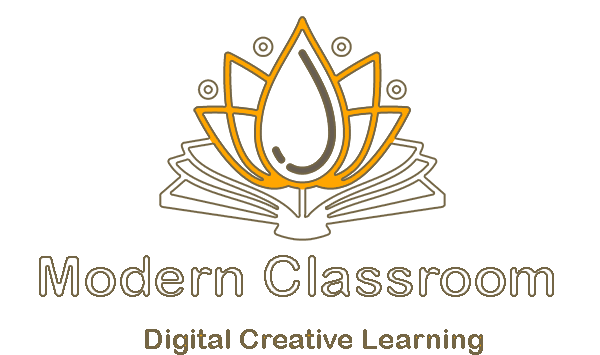
The Historical Perspective of Photosynthesis Summary Grade 11
On this page, we provide the historical perspective of photosynthesis summary.
Title: The Historical Perspective of Photosynthesis: A Journey Through Time
Photosynthesis, the process by which green plants, algae, and some bacteria convert light energy, primarily from the Sun, into chemical energy in the form of glucose, is the fundamental basis of life on Earth. The understanding and conceptualization of this complex process, however, is a testament to centuries of scientific inquiry. In this essay, we will embark on an exploration of the historical perspective of photosynthesis.
Table of Contents
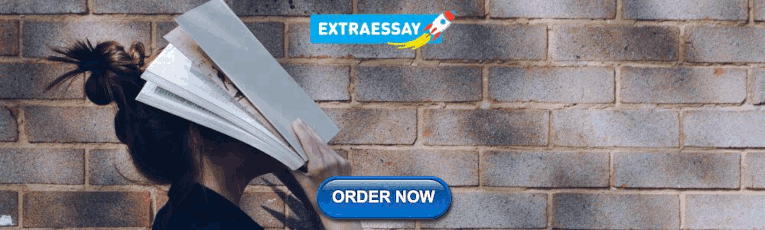
The Historical Perspective of Photosynthesis Summary
The ancients: early understanding and speculations.
Ancient civilizations, including the Greeks, Romans, and Egyptians, recognized the necessity of sunlight for plant growth, but the specific process of photosynthesis remained enigmatic. The notion of plants ‘feeding’ on soil was prevalent, but the essential role of light and its interaction with green parts of plants was yet to be identified. The importance of water in plant growth, too, was noted by early philosophers such as Thales of Miletus.
The 17th and 18th Centuries: Gaining Momentum
Jan Baptista van Helmont, a Belgian physician, conducted one of the earliest photosynthesis experiments in the 17th century. He demonstrated that the mass of a growing plant wasn’t sourced primarily from the soil but from water. This challenged the prevailing belief that plants drew all their nourishment from the soil.
In the 18th century, several scientists such as Joseph Priestley, Jan Ingenhousz, and Jean Senebier contributed to a deeper understanding of photosynthesis. Priestley found that plants could “restore” or “purify” air, which was later understood as the release of oxygen during photosynthesis. Ingenhousz demonstrated that this “restoration” occurred only in the presence of sunlight. Senebier, on the other hand, discovered that plants absorbed carbon dioxide during this process.
19th Century: Elucidation of the Photosynthesis Process
Julius Robert Mayer, a German physician and physicist, proposed the law of conservation of energy in the mid-19th century. This was crucial for understanding that sunlight was the source of the energy that plants stored during photosynthesis. Further advancements came from Julius Sachs who confirmed that chlorophyll in the chloroplasts was the site of photosynthesis and that oxygen was a by-product of this process.
Towards the end of the 19th century, T.W Engelmann conducted an experiment using a prism and filamentous algae to demonstrate that the highest rate of oxygen release (and thus photosynthesis) occurred in red and blue light. This formed the foundation of our understanding of the action spectrum of photosynthesis.
20th Century: Molecular Mechanisms and the Calvin Cycle
The 20th century marked a major turning point in the understanding of photosynthesis at a molecular level. Cornelis Van Niel proposed that photosynthesis was a process of addition and removal of hydrogen, influencing the subsequent work of many scientists.
The crowning achievement of the 20th century was the elucidation of the Calvin Cycle by Melvin Calvin and his team in the 1950s. They used the radioactive isotope carbon-14 to track the path of carbon in photosynthesis and uncovered the complete sequence of reactions through which plants manufacture glucose. This won Calvin the 1961 Nobel Prize in Chemistry.
21st Century: Contemporary Research and Future Directions
The 21st century saw advancements in our understanding of photosynthesis at the genetic and molecular level, thanks to emerging techniques in biotechnology and molecular biology. Scientists are now able to manipulate photosynthetic genes and proteins to optimize the efficiency of photosynthesis, leading to implications in sustainable agriculture and renewable energy.
Conclusion: Reflection on the Past, Vision for the Future
The understanding of photosynthesis has been a cumulative effort of many scientists across centuries, beginning from simple observations to sophisticated molecular explorations. The journey of understanding photosynthesis provides an excellent example of how scientific knowledge evolves over time.
Today, as we face unprecedented environmental challenges, photosynthesis has regained the spotlight. Through genetic engineering, scientists are trying to optimize the process to enhance crop yields and devise innovative solutions for carbon sequestration to mitigate the impacts of climate change. Additionally, researchers are exploring artificial photosynthesis to create sustainable energy sources.
As we reflect on the historical perspective of photosynthesis, it’s evident that this natural process, which largely contributes to life as we know it on our planet, will continue to be an important area of study. The future research on photosynthesis will play a critical role in the sustainable development of our world, emphasizing the significance of this process beyond the realms of biology and extending into the domains of agriculture, energy, and environmental science.
Did You See These?
- Life Sciences Grade 11 Term 1 March/ April 2022 Question Papers and Memo
- Grade 11 Life Sciences (Biology) Study Guides Free Download (South Africa)
- Life Sciences Grade 11 Past Papers and Memos PDF Download (South Africa)
- Life Science Grade 11 Photosynthesis Practical Question Papers (South Africa)
Leave a Comment Cancel reply
Save my name, email, and website in this browser for the next time I comment.
Celebrating 20 years of historical papers in Photosynthesis Research
- PMID: 16633907
- DOI: 10.1007/s11120-005-9023-y
This editorial has four goals: (1) to inform the readers of 'Photosynthesis Research' about the past of the 'Historical corner'; which began 20 years ago; (2) to encourage photosynthesis researchers and historians of science to contact me for publishing papers of historical interest; these include: (a) Obituaries and Tributes; (b) historical papers on current and past discoveries and controversies; (c) history of research in specific laboratories, or in specific countries, or at specific conferences; (d) Personal perspectives (not discussed any further); (3) to encourage researchers not to discard, but to save correspondence and data of their discoveries for the future historians by donating them to their Archives, when appropriate (not discussed any further); and (4) to reinforce to the readers that the concept of two-light reaction and two-pigment system was already there in 1959. I mention here three key papers presented at the IXth International Botanical Congress, held at Montreal Canada (in August, 1959) prior to the famous April 9, 1960 paper by Robert Hill and Fay Bendall on the 'Z-scheme' of photosynthesis, that was based on thermodynamic and energetic considerations.
Publication types
- Historical Article
- History, 20th Century
- History, 21st Century
- Periodicals as Topic / history*
- Photosynthesis / physiology*
- Publishing / history*
- Research / history*
List of biography and history published mostly in Photosynthesis Research , 1988–2008
- Published: 31 January 2009
- Volume 99 , pages 139–153, ( 2009 )
Cite this article
- Govindjee 1
2409 Accesses
2 Citations
3 Altmetric
Explore all metrics
“ The firefly seems afire, the sky looks flat; Yet sky and fly are neither this nor that ”
As an outgoing Editor of the Historical Corner of Photosynthesis Research , I present here the following list of papers of historical interest for the benefit of all. The first paper I published was: Govindjee ( 1988 ) The Discovery of Chlorophyll–protein Complex by Emil L. Smith during 1937–1941. Photosynth Res 16:285–289. In order to bring to the readers this List of references on the historical papers published in this journal (and some even elsewhere), I have organized these papers under the following headings (some are arbitrarily assigned to a particular section since they may fit in more than one section): (I) biographies (that include obituaries and tributes, arranged alphabetically, with dates of birth and death); (II) recognitions of scientists (arranged alphabetically) by others; (III) personal perspectives (arranged alphabetically); (IV) historical papers (first chronologically, by the year of publication, and then alphabetically by the names of the editors); (V) special issues of Photosynthesis Research (chronologically by the year of publication and then alphabetically by the names of editors); and lastly (VI) Conferences (available reports in Photosynthesis Research ).
Avoid common mistakes on your manuscript.
1 I Biographies
George Akoyunoglou (1927–1986)
Papageorgiou GC ( 1987 ) George Akoyunoglou (1927–1986). Photosynth Res 11:283–286
Jan Amesz (1934 – 2001)
Hoff AJ, Aartsma ( 2002 ) Jan Amesz (11 March 1934–29 January 2001). Photosynth Res 71:1–4
Daniel I. Arnon (1910 – 1994)
Buchanan BB ( 1995 ) Introduction: the life of Daniel I Arnon. Photosynth Res 46:3–6
Buchanan BB, Carlson D ( 1995 ) Daniel I Arnon: portrayal of a research career. Photosynth Res 46:7–12
Malkin R ( 1995 ) Daniel I Arnon (1910–1994). Photosynth Res 43(2):77–80
William Arnold (1904 – 2001)
Herron HA ( 1996 ) About Bill Arnold, my father. Photosynth Res 48(1–2):3–7
Pearlstein RM ( 1996 ) Bill Arnold: scientist, philosopher, friend. Photosynth Res 48(1–2):9–10
Strehler BL ( 1996 ) Halcyon days with Bill Arnold. Photosynth Res 48(1–2):11–18
Mordhay Avron (1931 – 1991)
Malkin S, Gromet-Elhanan Z ( 1992 ) Mordhay Avron (1931–1991). Photosynth Res 31(2):71–73
Sestak Z ( 1992 ) Mordhay Avron (1931–1991). Photosynthetica 26:163–164
Gerald T. Babcock (1946 – 2000)
Yocum C, Ferguson-Miller S, Blankenship R ( 2001 ) Gerald T Babcock (1946–2000). Photosynth Res 68(2):89–94
Charles Reid Barnes (1858 – 1910)
Gest H ( 2002 ) History of the word photosynthesis and evolution of its definition. Photosynth Res 73(1–3):7–10
Smocovitis VB ( 2006 ) One hundred years of American botany: a short history of Botanical Society of America. Am J Bot 93:942–952
John Biggins (1936 – 2004)
Bruce D, Sauer K ( 2005 ) John Biggins (1936–2004): his ingenuity, tenacity and humor; no-nonsense science with a big heart. Photosynth Res 85(3):261–265
Frederick Frost Blackman (1866 – 1947)
Briggs GE ( 1948 ) F.F. Blackman (1866–1947). Obit Notices Fellows R Soc 5(16):651–658
Steward FC, Memorial Committee ( 1947 ) In memoriam: Frederick Frost Blackman (July 25, 1866–January 30, 1947). Plant Physiol 22(3):ii–viii
Lawrence Blinks (1900 – 1989)
A symposium “A tribute to Lawrence R. Blinks: ions, light, and algae” was held on July 31, 2006, University of California-Chico, Botanical Society of America; Chair: Anitra Thorhaug. See abstracts at: http://www.2006.botanyconference.org/engine/search/?func=program&program=110#results
Lawrence Bogorad (1921 – 2003)
Rodermel S, Viret JF, Krebbers E ( 2005 ) Lawrence Bogorad (1921–2003), a pioneer in photosynthesis research: a tribute. Photosynth Res 83(1):17–24
Charles Bonnet (1720 – 1793)
Hedges TR Jr ( 2007 ) Charles Bonnet, his life and his syndrome. Surv Ophthalmol 52(1):111–114
Rieppel O ( 1985 ) The dream of Charles Bonnet (1720–1793). Gesnerus 42(3–4):359–367
Jagadish C. Bose (1858 – 1937)
Mukherjee DC, Sen D ( 2007 ) A tribute to Sir Jagadish Chandra Bose (1858–1937). Photosynth Res 91(1):1–10
Jean-Marie Briantais (1936 – 2004)
de Kouchkovsky Y, Cerovic ZG ( 2005 ) Jean-Marie Briantais (1936–2004), a friend and a champion of interactive and integrative research. Photosynth Res 83(1):1–3
Allan H. Brown (1917 – 2004)
Black CC, Mayne BC ( 2006 ) Allan H Brown (1917–2004), editor and educator: a career of fascination with the biological roles of O 2 in terrestrial life and possibly in extraterrestrial life. Photosynth Res 87(2):159–163
Warren L Butler (1925 – 1984)
Bishop NI ( 1986 ) Warren L Butler; a tribute to a friend and fellow scientist. Photosynth Res 10(3):147–149
Govindjee ( 1986 ) Publications of Warren L Butler on photosynthesis. Photosynth Res 10(3):151–161
Melvin Calvin (1911 – 1997)
Loach P ( 1997 ) A remembrance of Melvin Calvin. Photosynth Res 54(1):1–3
George Cheniae (1928 – 2001)
Frasch WD, Sayre RT ( 2001 ) Remembering George Cheniae, who never compromised his high standards of science. Photosynth Res 70(3):245–247
Germaine Cohen-Bazire (Stanier) (1920 – 2001)
Rippka R ( 2003 ) Germaine Stanier (Cohen-Bazire) 1920–2001. Arch Hydrobiol-Suppl 148:17–34
Therese M. Cotton-Uphaus (1939 – 1998)
Seibert M, Thurnauer M ( 1999 ) Therese Marie Cotton-Uphaus (1939–1998). Photosynth Res 61(3):193–196
R.H. Dastur (1896 – 1961)
Asana RD ( 1961 ) Prof. R.H. Dastur, O.B.E. Nature 192:1128
Nicholas Theodore De Saussure (1767 – 1845)
Hart H ( 1930 ) Nicolas Theodore De Saussure. Plant Physiol 5(3):424–429
Don Charles DeVault (1915 – 1990)
Parson WW ( 1989 ) Don DeVault. A tribute on the occasion of his retirement. Photosynth Res 22(1):11–13
Seibert M ( 1991 ) Don Charles DeVault. Photosynth Res 28(3):95–98
Karl Egle (1912 – 1975)
Fock H ( 1976 ) Professor Dr. Karl Egle (1912–1975). Photosynthetica 10: unnumbered pages (in German)
Theodor W. Engelmann (1843 – 1909)
Drews G ( 2005 ) Contributions of Theodor Wilhelm Engelmann on phototaxis, chemotaxis, and photosynthesis. Photosynth Res 83(1):25–34
Michael C.W. Evans (1940 – 2007)
Heathcote P, Nugent J ( 2008 ) Michael Charles Whitmore Evans (September 24, 1940–February 21, 2007). Photosynth Res 96(1):1–4
Agnes Faludi Daniel (1929 – 1986)
Garab G, Mustardy L, Demeter S ( 1987 ) Agnes Faludi Daniel (1929–1986). Photosynth Res 13:99–100
Gordon E. (Tony) Fogg (1919 – 2005)
Thake B ( 2006 ) Gordon Elliott (Tony) Fogg (1919–2005): pioneering plant physiologist and gifted writer. Photosynth Res 90(1):1–4
James Franck (1882 – 1964)
Rosenberg JL ( 2004 ) The contributions of James Franck to photosynthesis research: a tribute. Photosynth Res 80(1–3):71–76
Charles Stacy French (1907 – 1995)
Fork DC ( 1996 ) Charles Stacy French: a tribute. Photosynth Res 49(1):91–101
Fork DC ( 1996 ) Charles Stacy French (1907–1995). Photosynthetica 33:1–6
Yoshihiko Fujita (1932 – 2005)
Murakami A, Mimuro M ( 2006 ) Yoshihiko Fujita (1932–2005): a pioneer of photoregulation in cyanobacteria. Photosynth Res 88(1):1–5; erratum: p. 7
Hans Gaffron (1902 – 1979)
Homann PH ( 2003 ) Hydrogen metabolism of green algae: discovery and early research—a tribute to Hans Gaffron and his coworkers. Photosynth Res 76(1–3):93–103
Martin Gibbs (1922 – 2006)
Black CC Jr ( 2008 ) Martin Gibbs (1922–2006): pioneer of 14 C research, sugar metabolism & photosynthesis; vigilant editor-in-chief of Plant Physiology ; sage educator; and humanistic mentor. Photosynth Res 95(1):1–10
Black CC, Govindjee ( 2008 ) Martin Gibbs and the peaceful uses of nuclear radiation, 14 C. Photosynth Res 99(1):63–80
Tikhon N. Godnev (1892 – 1982)
Virgin H, Volotovskii ( 1993 ) Tikhon N. Godnev (1892–1982). Photosynthetica 29:163–165
Norman E. Good (1917 – 1992)
Hangarter RP, Ort DR ( 1992 ) Norman E Good (1917–1992). Photosynth Res 34(2):245–247
David John Goodchild (1930 – 1989)
Anderson JM ( 1990 ) David John Goodchild. Photosynth Res 24(2):115–116
Paul R. Gorham (1918 – 2006)
Nozzolillo CG, Gorham H, Govindjee ( 2007 ) Paul R Gorham (April 16, 1918–November 9, 2006). Photosynth Res 92(1):3–5
Zippora Gromet-Elhanan (1931 – 2007)
McCarty RE ( 2008 ) Zippora Gromet-Elhanan (1931–2007), a passionate and fiercely dedicated scientist. Photosynth Res 96(2):117–119
David Hall (1935 – 1999)
Rao KK ( 1999 ) David Hall (1935–1999). Photosynth Res 62(2):117–119
Per Halldal (1922 – 1986)
Björn LO, Sundqvist C, Öquist G ( 2007 ) A tribute to Per Halldal (1922–1986), a Norwegian photobiologist in Sweden. Photosynth Res 92(1):7–11
Robert Hill (1899 – 1991)
Anderson MC ( 1993 ) Robin Hill, FRS: a Cambridge neighbor’s appreciation of a great man and his hemispherical camera. Photosynthetica 28:321–322
Bendall DS, Walker DA ( 1991 ) Robert (Robin) Hill (1899–1991). Photosynth Res 30(1):1–5
Goodwin J ( 1992 ) Dr Robin Hill: natural dyes. Photosynth Res 34(3):321–322
Govindjee ( 2001 ) Calvin and Hill prizes: 2001. Photosynth Res 70(3):325–328
Walker DA ( 2002 ) ‘And whose bring presence’—an appreciation of Robert Hill and his reaction. Photosynth Res 73(1–3):51–54
Gábor Horváth (1944 – 2000)
Garab G ( 2000 ) Gábor Horváth (1944–2000). Photosynth Res 65(2):103–105
Jan Ingen-Housz (1730 – 1799)
Gest H ( 2000 ) Bicentenary homage to Dr Jan Ingen-Housz, MD (1730–1799), pioneer of photosynthesis research. Photosynth Res 63(2):183–190
Seikichi Izawa (1926 – 1997)
Berg S ( 1998 ) Seikichi Izawa (1926–1997). Photosynth Res 58(1):1–4
Melvin P. Klein (1921 – 2000)
Britt RD, Sauer K, Yachandra VK ( 2000 ) Remembering Melvin P Klein. Photosynth Res 65(3):201–206
Elena N. Kondratieva (1925 – 1995)
Olson JM, Ivanovsky RN, Fuller RC ( 1996 ) Elena N Kondratieva (1925–1995). Photosynth Res 47(3):203–205
Hugo P. Kortschak (1911 – 1983)
Nickell LG ( 1993 ) A tribute to Hugo P Kortschak: the man, the scientist and the discoverer of C 4 photosynthesis. Photosynth Res 35(2):201–204
Alexander Abramovich Krasnovsky (1913 – 1993)
Karapetyan N ( 1993 ) AA Krasnovsky (1913–1993). Photosynthetica 29:481–485
Karapetyan N ( 1993 ) AA Krasnovsky (1913–1993). Photosynth Res 38(1):1–3
Julio López-Gorgé (1935 – 2004)
Sahrawy Barragán M ( 2005 ) A tribute to Julio López-Gorgé (1935–2004): the music in science. Photosynth Res 83(3):283–286
Henrik Lundegårdh (1888 – 1969)
Larkum AWD ( 2003 ) Contributions of Henrik Lundegårdh. Photosynth Res 76(1–3):105–110
Helmut Metzner (1925 – 1999)
Fischer-Zeh K ( 2000 ) Helmut Metzner (1925–1999). Photosynth Res 63(3):191–194
Lee McIntosh (1949 – 2004)
Kende H ( 2006 ) Remembering Lee McIntosh (1949–2004), a pioneer in the molecular biology of chloroplast and mitochondrion function. Photosynth Res 87(3):247–251
Peter Mitchell (1920 – 1992)
Crofts A ( 1993 ) Peter Mitchell (1920–1992). Photosynth Res 35(1):1–4
Hans Molisch (1856 – 1937)
Gest H ( 1991 ) The legacy of Hans Molisch (1856–1937), photosynthesis savant. Photosynth Res 30(1):49–59
Alexis Moyse (1912 – 1991)
Champigny ML ( 1992 ) Alexis Moyse (1912–1991). Photosynthetica 26:161–162
Jack E. Myers (1913 – 2006)
Brand JJ, Krogman DW, Patterson CO ( 2008 ) Jack Edgar Myers (1913–2006), an algal physiologist par excellence . Photosynth Res 96(1):9–14
André Pirson (1910 – 2004)
Senger H ( 2004 ) Tribute: in memory of professor Dr Dr hc André Pirson, a pioneer in photosynthesis and a dedicated academic teacher. Photosynth Res 82(2):111–114
John R. Quayle (1926 – 2006)
Kornberg HL ( 2006 ) John Rodney Quayle (1926–2006), a brilliant scientist who was also a wise and innovative academic administrator. Photosynth Res 89(2–3):59–62
Efraim Racker (1931 – 1991)
Nelson N ( 1992 ) Efraim Racker (1913–1991). Photosynth Res 31(3):165–166
K. Krishna Rao (1928 – 2006)
Cammack R ( 2006 ) K Krishna Rao—a lifetime study of ferredoxins and solar hydrogen. Photosynth Res 90(2):97–99
August Ried (1924 – 2004)
Strotmann H, Soeder C-J ( 2005 ) August Ried (1924–2004), an outstanding researcher, and artist and a dear friend. Photosynth Res 83(3):279–281
Eugene Roux (1924 – 2004)
Lutz M, Galmiche JM ( 1987 ) Eugene Roux (1924–2004). Photosynth Res 12:91–93
Samuel Ruben (1913 – 1943)
Gest H ( 2004 ) Samuel Ruben’s contributions to research on photosynthesis and bacterial metabolism with radioactive carbon. Photosynth Res 80(1–3):77–83
Noun Shavit (1930 – 1997)
Aflalo C, Baum H, Chipman DM, McCarty RE, Strotmann H ( 1997 ) Noun Shavit (1930–1997). Photosynth Res 54(3):165–167
Alexander A. Shlyk (1928 – 1984)
Krasnovsky AA ( 2003 ) Alexander A. Shlyk (1928–1984). Photosynth Res 76:389–403
Krasnovsky AA, Voltovski ID, Chaika MT, Fradkin LI ( 1985 ) Alexander A. Shlyk (1928–1984). Photosynthetica 19:485–486
Gauri S. Singhal (1933 – 2004)
Andley UP, Velagaleti PNR, Sen A, Tripathy BC ( 2005 ) Gauri Shankar Singhal (1933–2004): a photochemist, a photobiologist, a great mentor and a generous friend. Photosynth Res 85(2):145–148
William R. Sistrom (1927 – 1993)
Castenholz RW ( 1994 ) William R Sistrom (1927–1993). Photosynth Res 42(3):167–168
Stanier, Roger (1916 – 1982)
Ingraham JL ( 1982 ) Roger Y. Stanier (1916–1982). Arch Mikrobiol 133(1):1
Ken-ichiro Takamiya (1943 – 2005)
Ohta H, Masuda T, Matsuura K ( 2008 ) Ken-ichiro Takamiya (1943–2005), a gentleman and a scientist, a superb experimentalist and a visionary. Photosynth Res 97(2):115–119
Hiroshi Tamiya (1903 – 1986)
Sestak Z ( 1986 ) Hiroshi Tamiya (1903–1986). Photosynthetica 20:81
Vidyadhar G. (Pandit) Tatake (1926 – 2004)
Sane PV (Raj), Phondke GP (Bal) ( 2006 ) Vidyadhar Govind (Pandit) Tatake (1926–2004): an ingenious instrumentalist, an authority on thermoluminescence, and a lover of classical Indian music. Photosynth Res 89(1):49–51
Jan Bartholomeus Thomas (1907 – 1991)
van Ginkel G, Goedheer J ( 1991 ) Jan Bartholomeus Thomas (1907–1991). Photosynth Res 30(2–3):65–69
Philip Thornber (1934 – 1996)
Cogdell R ( 1996 ) Philip Thornber (1934–1996). Photosynth Res 50(1):1–3
Nathan Edward Tolbert (1919 – 1998)
Goyal A ( 2000 ) Ed Tolbert and his love for science: a journey from sheep ranch continues…. Photosynth Res 65(1):1–6
Cornelis Bernardus van Niel (1897 – 1985)
Hungate RE ( 1986 ) Cornelis Bernardus van Niel (1897–1985). Photosynth Res 10(1–2):139–142
Ilya Vassiliev (1959 – 2005)
Barry BA ( 2006 ) Ilya Vassiliev (January 12, 1959–August 10, 2005). Photosynth Res 87(3):245–246
Birgit Vennesland (1913 – 2001)
Conn EE, Pistorius EK, Solomonson LP ( 2005 ) Remembering Birgit Vennesland (1913–2001), a great biochemist. Photosynth Res 83(1):11–16
Hemming Virgin (1918 – 2005)
Sundqvist C, Björn LO ( 2007 ) A tribute to Hemming Virgin (1918–2005), a Swedish pioneer in plant photobiology. Photosynth Res 92(1):13–16
E.C. Wassink (1904 – 1981)
Vredenberg WJ ( 1981 ) Professor Dr. E.C. Wassink (1904–1981). Photosynthetica 15:315–316
Samuel G. Wildman (1912 – 2004)
Tobin E ( 2006 ) Samuel Goodnow Wildman (1912–2004): discoverer of fraction I protein, later named Rubisco, who worked till he was 92. Photosynth Res 88(2):105–108
Horst T. Witt (1922 – 2007)
Renger G ( 2008 ) Horst Tobias Witt (March 1, 1922–May 14, 2007). Photosynth Res 96(1):5–8
René Wurmser (1890 – 1993)
Joliot P ( 1996 ) René Wurmser (September 24, 1890–November 9, 1993). Photosynth Res 48(3):321–323
2 II Recognitions
William A. Arnold
Duysens LNM ( 1996 ) W.A. Arnold’s inspiring experiments. Photosynth Res 48(1–2):25–29
Knox RS ( 1996 ) Electronic excitation transfer in the photosynthetic unit: reflections on work of William Arnold. Photosynth Res 48(1–2):35–39
Lavorel J ( 1996 ) The importance of being lucky: a tribute to William Arnold. Photosynth Res 48(1–2):31–34
Malkin S, Fork DC ( 1996 ) Bill Arnold and calorimetric measurements of the quantum requirement of photosynthesis-once again ahead of his time. Photosynth Res 48(1–2):41–46
Mauzerall D ( 1996 ) Bill Arnold’s concept of solid state photosynthesis and his discoveries. Photosynth Res 48(1–2):19–23
Daniel I. Arnon
Buchanan BB, Tagawa K ( 1995 ) Perspective on Daniel I Arnon’s contributions to research, 1960–1994. Photosynth Res 46(1–2):27–35
Andrew A. Benson
Anderson JM ( 2007 ) Thylakoid membrane landscape in the sixties: a tribute to Andrew Benson. Photosynth Res 92(2):193–197
Buchanan BB, Douce R, Lichtenthaler HK ( 2007 ) Andrew A Benson. Photosynth Res 92(2):143–144
Jeffrey SW ( 2007 ) Professor Andrew A Benson: inspirational mentor. Photosynth Res 92(2):187–192
Lichtenthaler HK, Buchanan BB, Douce R ( 2008 ) Honoring Andrew Benson in Paris. Photosynth Res 92(2):181–183
Olle Björkman
Govindjee ( 2001 ) Our greetings to Olle Björkman, Christopher Field, and Alexander Glazer. Photosynth Res 70(2):241–243
Warren Butler
Govindjee, Barber J, Cramer WA, Goedheer JHC, Lavorel J, Macelle R, Zilinskas B (eds) ( 1986 ) Excitation and electron transfer in photosynthesis—special issue—dedicated to Warren L. Butler. Photosynth Res 10:147–518
Melvin Calvin
Don Devault
Blankenship RE, Amesz J, Holten D, Jortner J (eds) ( 1989 ) Tunneling processes in photosynthesis—dedicated to Donald DeVault. Part 1. Photosynth Res 22:1–122
Blankenship RE, Amesz J, Holten D, Jortner J (eds) ( 1989 ) Tunneling processes in photosynthesis—dedicated to Donald DeVault. Part 2: Photosynth Res 22:173–301
Parson WW ( 1989 ) Don Devault: a tribute on the occasion of his retirement. Photosynth Res 22(1):11–13
Louis N.M. Duysens
Amesz J, Hoff AJ, Van Gorkom HJ (eds) ( 1986 ) Current topics in Photosynthesis—double issue dedicated to Professor Louis N. M. Duysens on the occasion of his retirement. Photosynth Res 9:1–283
Robert Emerson (1903 – 1959)
Emerson had passed away long before ‘Photosynthesis Research’ came into existence, but no article has appeared thus far dedicated to him in this journal. I, however, list below three articles on him, published elsewhere.
Govindjee ( 2001 ) Lighting the path: a tribute to Robert Emerson (1903–1959). S43-001 (6 pp); available free at http://www.publish.csiro.au/?act=view_file&file_id=SA0403744.pdf
[site to download all papers in the Proceedings of the 12th international congress on photosynthesis. Online is at http://www.publish.csiro.au/issue/1342.htm ]
Govindjee ( 2004 ) Robert Emerson and Eugene Rabinowitch: understanding photosynthesis. In: Hoddeson L (ed) No boundaries. University of Illinois Vignettes. University of Illinois Press, Urbana, pp 181–194
Rabinowitch E ( 1961 ) Robert Emerson (1903–1959). Biogr Mem Natl Acad Sci USA 25:112–131
Christopher Field
Alexander Glazer
Eaton-Rye JJ ( 2007 ) Celebrating Govindjee’s 50 years in Photosynthesis Research and his 75th birthday. Photosynth Res 93(1–3):1–5
Eaton-Rye JJ ( 2007 ) Snapshots of the Govindjee lab from the late 1960s to the late 1990s, and beyond. Photosynth Res 94(2–3):153–178
Rebeiz CA, Benning C, Bohnert H, Hoober JK, Portis AR ( 2007 ) Govindjee was honored with the first lifetime achievement award, and Britta Förster and coworkers, with the first annual paper prize of Rebeiz foundation for basic research. Photosynth Res 94(1):147–151
Robert Hill
Kamen MD ( 1992 ) Robert (‘Robin’) Hill: an appreciation. Photosynth Res 34(3):323–325
Krasnovsky AA ( 1992 ) Two days with Robin Hill and forty-five years with Hill reaction. Photosynth Res 34(3):327–328
Prince RC ( 1992 ) Robert Hill, FRS; his published work. Photosynth Res 34(3):329–332
Rich PR ( 1992 ) Robin Hill: a personal perspective. Photosynth Res 34(3):333–335
Walker DA ( 1992 ) Robert Hill. Photosynth Res 34(3):337–338
Jan Ingen-Housz
Gest H ( 1997 ) A misplaced chapter in the history of photosynthesis research. The second publication (1796) on plant processes by Dr. Jan Ingen-Housz, MD, discoverer of photosynthesis, Photosynth Res 53:65–72
Gest H ( 2000 ) Bicentenary homage to Jan Ingen-Housz, pioneer of photosynthesis research. Photosynth Res 63:183–190
Myroslawa Miginiac-Maslow
Gadal P ( 2004 ) Myroslawa Miginiac-Maslow. Photosynth Res 79(3):229–230
Jacquot J-P ( 2004 ) Comments on the contributions of Myroslawa Miginiac-Maslow and Peter Schürmann to the light-dependent redox regulation of choloroplastic enzymes. Photosynth Res 79(3):231–232
Eugene I. Rabinowitch (1898 – 1973)
Bannister TT ( 1972 ) The careers and contributions of Eugene Rabinowitch. Biophys J 12(7):707–718
Brody SS ( 1995 ) We remember Eugene. Photosynth Res 43(1):67–74
Rabinowitch A ( 2005 ) Founder and father. Bull At Sci 61(1):30–37
Rotblatt J ( 2000 ) Fifty Pugwash conferences: a tribute to Eugene Rabinowitch. Available online at: http://www.pugwash.org/reports/pac/pac256/rotblat.htm
Kimiyuki Satoh
Enami I, Shen J-R ( 2008 ) A brief introduction of Kimiyuki Satoh. Photosynth Res 98(1–3):7–11
Ohta H, Masuda T, Matsuura K ( 2008 ) Professor Ken-ichiro Takamiya (1943–2005) gentleman & a scientist, a superb experimentalist and a visionary. Photosynth Res 97(2):115–119
Peter Schürmann
Buchanan BB ( 2004 ) Peter Schürmann. Photosynth Res 79(3):227–228
Emil L. Smith
Govindjee ( 1988 ) The discovery of chlorophyll–protein complex by Emil L. Smith during 1937–1941. Photosynth Res 16:285–289
Thomas J. Wydrzynski
Govindjee ( 2008 ) Recollections of Thomas John Wydrzynski. Photosynth Res 98(1–3):13–31
Charles F. Yocum
Siedow JN ( 2002 ) A biographical sketch of Charles F Yocum: “it’s the biochemistry, stupid.” Photosynth Res 72(2):123–130
3 III Personal perspectives
Akazawa T ( 1994 ) Reminiscences, collaborations and reflections. Photosynth Res 46(1–2):93–113
Arnold WA ( 1991 ) Experiments. Photosynth Res 27(2):73–82
Barber J ( 2004 ) Engine of life and big bang of evolution: a personal perspective. Photosynth Res 80(1–3):137–155
Benson AA ( 2002 ) Paving the path. Annu Rev Plant Biol 53:1–25
Calvin M ( 1989 ) Forty years of photosynthesis and related activities. Photosynth Res 21(1):1–16
Chance B ( 1991 ) Optical method. Annu Rev Biophys Biophys Chem 20:1–28
Clayton RK ( 1988 ) Memories of many lives. Photosynth Res 19(3):205–224
Devault D ( 1989 ) Tunneling enters biology. Photosynth Res 22(1):5–10
Drews G ( 1996 ) Forty-five years of developmental biology of photosynthetic bacteria. Photosynth Res 48:325–352
Duysens LNM ( 1989 ) The discovery of the two photosynthetic systems: a personal account. Photosynth Res 21(2):61–79
Feher G ( 1998 ) Three decades of research in bacterial photosynthesis and the road leading to it: a personal account. Photosynth Res 55(1):1–40
Feher G ( 2002 ) My road to biophysics: picking flowers on the path to photosynthesis. Annu Rev Biophys Biomol Struct 31:1–44
Forti G ( 1999 ) Personal recollections of 40 years in photosynthesis research. Photosynth Res 60(2–3):99–110
French CS ( 1979 ) Fifty years of photosynthesis. Annu Rev Plant Physiol 30:1–26
Frenkel AW ( 1993 ) Recollections. Photosynth Res 35(2):103–116
Fujita Y ( 1997 ) A study on the dynamic features of photosystem stoichiometry: accomplishments and problems for future studies. Photosynth Res 53(2–3):83–93
Fuller RC ( 1999 ) Forty years of microbial photosynthesis research: where it came from and what it led to. Photosynth Res 62(1):1–29
Gaffron H ( 1969 ) Resistance to knowledge. Annu Rev Plant Physiol 20:1–40
Gerhart D ( 1996 ) Forty-five years of developmental biology of photosynthetic bacteria. Photosynth Res 48(3):325–352
Gest H ( 1994 ) A microbiologist’s odyssey: bacterial viruses to photosynthetic bacteria. Photosynth Res 40(2):129–146
Gest H ( 1994 ) Discovery of the heliobacteria. Photosynth Res 41(1):17–21
Gest H ( 1999 ) Memoir of a 1949 railway journey with photosynthetic bacteria. Photosynth Res 61(1):91–96
Gibbs M ( 1999 ) Educator and editor. Annu Rev Plant Physiol Plant Mol Biol 50:1–25
Good NE ( 1986 ) Confessions of a habitual skeptic. Annu Rev Plant Physiol 37:1–22
Gunsalus IC ( 1984 ) Learning. Annu Rev Microbiol 38:1–26
Hatch MD (Hal) ( 1992 ) I can’t believe my luck. Photosynth Res 33(1):1–14
Hill R ( 1975 ) Days of visual spectroscopy. Annu Rev Plant Physiol 26:1–11
Jagendorf AT ( 1998 ) Chance, luck and photosynthesis research: an inside story. Photosynth Res 57(3):215–229
Joliot P ( 1993 ) Earlier researches on the mechanism of oxygen evolution: a personal account. Photosynth Res 38(3):214–223
Kamen MD ( 1986 ) A cupful of luck, a pinch of sagacity. Annu Rev Biochem 55:1–34
Kamen MD ( 1989 ) Onward into a fabulous half-century. Photosynth Res 21(3):137–144 [Also see Kauffman GB ( 2002 ) Martin D. Kamen (1913–2002), nuclear scientist and biochemist. Chem Educ 7:304–308]
Katoh S ( 1995 ) The discovery and function of plastocyanin: a personal account. Photosynth Res 43(3):177–189
Katz JJ ( 1990 ) Green thoughts in a green shade. Photosynth Res 26(3):143–160
Krasnovsky AA ( 1992 ) Excited chlorophyll and related problems. Photosynth Res 33:177–193
Krogmann DW ( 2000 ) The golden age of biochemical research in photosynthesis. Photosynth Res 63(2):109–121
Menke W ( 1990 ) Retrospective of a botanist. Photosynth Res 25(2):77–82
Myers J ( 1996 ) Country boy to scientist. Photosynth Res 50(3):195–208
Myers J ( 2002 ) In one ear and out the other. Photosynth Res 73(1–3):21–28
Ochoa S ( 1980 ) The pursuit of a hobby. Annu Rev Biochem 49:1–30
Olson JM ( 1994 ) Reminiscence about ‘Chloropseudomonas ethylicum’ and the FMO-protein. Photosynth Res 41(1):3–5
Pierson BK ( 1994 ) Reflections on Chloroflexux. Photosynth Res 41(1):7–15
Pirson A ( 1994 ) Sixty years in algal physiology and photosynthesis. Photosynth Res 40(3):207–221
San Pietro A ( 2008 ) Memories: from protein synthesis to photosynthesis. Photosynth Res 96(3):185–199
Shen Y ( 1994 ) Dynamic approaches to the mechanism of photosynthesis. Photosynth Res 39(1):1–13
Stanier RY ( 1980 ) The journey, not the arrival matters. Annu Rev Microbiol 34:1–48
Sweeney BM ( 1987 ) Living in the golden age of biology. Annu Rev Plant Physiol 38:1–9
Tamiya H ( 1966 ) Synchronous cultures of algae. Annu Rev Plant Physiol 17:1–21
Thornber JP ( 1995 ) Thirty years of fun with antenna pigment-proteins and photochemical reaction centers: a tribute to the people who have influenced my career. Photosynth Res 44(1–2):3–22
Tolbert NE ( 1997 ) The C2 oxidative photosynthetic cycle. Annu Rev Plant Physiol Plant Mol Biol 48:1–25
Van Niel CB ( 1967 ) The education of a microbiologist: some reflections. Annu Rev Microbiol 21:1–30
Vennesland B ( 1981 ) Recollections and small confessions. Annu Rev Plant Physiol 32:1–48
Walker DA ( 1997 ) ‘Tell me where all the past years are’. Photosynth Res 51:1–26
Warburg O ( 1964 ) Prefatory chapter. Annu Rev Biochem 33:1–14
Weber G ( 1990 ) Whither biophysics. Annu Rev Biophys 19:1–6
Witt HT ( 1991 ) Functional mechanism of water splitting photosynthesis. Photosynth Res 29(2):55–77
Zelitch I ( 2001 ) Travels in a world of small science. Photosynth Res 67(3):157–176
4 IV Historical papers
Benning C ( 2007 ) Questions remaining in sulfolipid biosynthesis: a historical perspective. Photosynth Res 92(2):199–203
Block MA, Douce R, Joyard J, Rolland N ( 2007 ) Chloroplast envelop membranes: a dynamic interface between plastids and the cytosol. Photosynth Res 92(2):225–244
Buchanan BB ( 2007 ) Thioredoxin: an unexpected meeting place. Photosynth Res 92(2):145–148
Govindjee, Yoo H ( 2007 ) The International Society of Photosynthesis Research (ISPR) and its associated international congress on photosynthesis (ICP) a pictoral report. Photosynth Res 91:95–106
Höxtermann E ( 2007 ) A comment on Warburg’s early understanding of biocatalysis. Photosynth Res 92(1):121–127
Nickelsen K ( 2007 ) Otto Warburg’s first approach to photosynthesis. Photosynth Res 92(1):109–120
Portis AR Jr, Parry MAJ ( 2007 ) Discoveries in Rubisco (Ribulose 1,5-bisphosphate carboxylase/oxygenase): a historical perspective. Photosynth Res 94(1):121–143
Trebst A ( 2007 ) Inhibitors in the functional dissection of the photosynthetic electron transport system. Photosynth Res 92(2):217–224
Wada H, Murata N ( 2007 ) The essential role of phosphatidylglycerol in photosynthesis. Photosynth Res 92(2):205–215
Walker DA ( 2007 ) From Chlorella to chloroplasts: a personal note. Photosynth Res 92(2):181–185
Forti G, Agostiano A, Barbato R, Bassi R, Brugnoli E, Finazzi G, Garlaschi FM, Jennings RC, Melandri BA, Trotta M. Venturoli G, Zanetti G, Zannoni D, Zucchelli G ( 2006 ) Photosynthesis Research in Italy: a review. Photosynth Res 88(3):211–240
Giacometti GM, Giacometti G ( 2006 ) Twenty years of biophysics of photosynthesis in Padova, Italy (1984–2005): a tale of two brothers. Photosynth Res 88(3):241–258
Gorham PR, Nozzolillo CG ( 2006 ) Photosynthesis research in Canada from 1945 to the early 1970s. Photosynth Res 88(1):83–100
Govindjee ( 2006 ) Celebrating 20 years of historical papers in photosynthesis research. Photosynth Res 87(2):151–158
Zeinalov Y ( 2006 ) A brief history of the investigations on photosynthesis in Bulgaria. Photosynth Res 88(2):195–204
Williams RJP ( 2005 ) The discovery of the nature of ferredoxin in photosystems: a recollection. Photosynth Res 85(2):247–250
Allen JP ( 2004 ) My daily constitutional in Martinsried. Photosynth Res 80(1–3):157–163
Bauer C ( 2004 ) Regulation of photosystem synthesis in Rhodobacter capsulatus . Photosynth Res 80(1–3):353–360
Bendall DS ( 2004 ) The unfinished story of cytochrome f . Photosynth Res 80(1–3):265–276
Camm EL, Green BR ( 2004 ) How the chlorophyll-proteins got their names. Photosynth Res 80(1–3):189–196
Chance B ( 2004 ) The stopped-flow method and chemical intermediates in enzyme reactions—a personal essay. Photosynth Res 80(1–3):387–400
Cogdell RJ, Hashimoto H, Gardiner AT ( 2004 ) Purple bacterial light-harvesting complexes: from dreams to structures. Photosynth Res 80(1–3):173–179
Cramer WA ( 2004 ) Ironies in photosynthetic electron transport: a personal perspective. Photosynth Res 80(1–3):293–305
Crofts AR ( 2004 ) The Q-cycle—a personal perspective. Photosynth Res 80(1–3):223–243
Dilley RA ( 2004 ) On why thylakoids energize ATP formation using either delocalized or localized proton gradients—a Ca 2+ mediated role in thylakoid stress responses. Photosynth Res 80(1–3):245–263
Ellis RJ ( 2004 ) From chloroplasts to chaperones: how one thing led to another. Photosynth Res 80(1–3):333–343
Fajer J ( 2004 ) Chlorophyll chemistry before and after crystals of photosynthetic reaction centers. Photosynth Res 80(1–3):165–172
Fromme P, Mathis P ( 2004 ) Unraveling the photosystem I-reaction center: a history, or the sum of many efforts. Photosynth Res 80(1–3):109–124
Gest H, Blankenship RE ( 2004 ) Time line of discoveries: anoxygenic bacterial photosynthesis. Photosynth Res 80(1–3):59–70
Ghosh AK ( 2004 ) Passage of a young Indian physical chemist through the world of photosynthesis research at Urbana, Illinois, in the 1960s: a personal essay. Photosynth Res 80(1–3):427–437
Govindjee, Krogmann D ( 2004 ) Discoveries in oxygenic photosynthesis (1727–2003): a perspective. Photosynth Res 80(1–3):15–57
Govindjee, Allen JF, Beatty JT ( 2004 ) Celebrating the millennium: historical highlights of photosynthesis research, part 3. Photosynth Res 80(1–3):1–13
Hangarter RP, Gest H ( 2004 ) Pictorial demonstrations of photosynthesis. Photosynth Res 80(1–3):421–425
Hauska G ( 2004 ) The isolation of a functional cytochrome b 6 f complex: from lucky encounter to rewarding experiences. Photosynth Res 80(1–3):277–291
Jensen RG ( 2004 ) Activation of rubisco controls CO 2 assimilation in light: a perspective on its discovery. Photosynth Res 82(2):187–193
Junge W ( 2004 ) Protons, proteins and ATP. Photosynth Res 80(1–3):197–221
Melis A, Happe T ( 2004 ) Trails of green alga hydrogen research—from Han Gaffron to new frontiers. Photosynth Res 80(1–3):401–409
Olson JM ( 2004 ) The FMO protein. Photosynth Res 80(1–3):181–187
Olson JM, Blankenship RE ( 2004 ) Thinking about the evolution of photosynthesis. Photosynth Res 80(1–3):373–386
Shin M ( 2004 ) How is ferredoxin-NADP reductase involved in the NADP photoreduction of chloroplasts? Photosynth Res 80(1–3):307–313
Tabita FR ( 2004 ) Research on carbon dioxide fixation in photosynthetic microorganisms (1971–present). Photosynth Res 80(1–3):315–332
Wildman SG, Hirsch AM, Kirchanski SJ, Spencer D ( 2004 ) Chloroplasts in living cells and the string-of-grana concept of chloroplast structure revisited. Photosynth Res 80(1–3):345–352
Witt HT ( 2004 ) Steps on the way to building blacks, topologies, crystals and X-ray structural analysis of photosystems I and II of water-oxidizing photosynthesis. Photosynth Res 80(1–3):85–107
Woese CR ( 2004 ) The archaeal concept and the world it lives in: a retrospective. Photosynth Res 80(1–3):361–372
Wydrzynski TJ ( 2004 ) Early indications for manganese oxidation state changes during photosynthetic oxygen production: a personal account. Photosynth Res 80(1–3):125–135
Xiong L, Sayre RT ( 2004 ) Engineering the chloroplast encoded proteins of Chlamydomonas. Photosynth Res 80(1–3):411–419
Adir N, Zer H, Shochat S, Ohad I ( 2003 ) Photoinhibition—a historical perspective. Photosynth Res 76(1–3):343–370
Albertsson P-A ( 2003 ) The contribution of photosynthetic pigments to the development of biochemical separation methods: 1990–1980. Photosynth Res 76(1–3):217–225
Armitage JP, Hellingwerf KJ ( 2003 ) Light-induced behavioral responses (‘phototaxis’) in prokaryotes. Photosynth Res 76(1–3):145–155
Bassham JA ( 2003 ) Mapping the carbon reduction cycle: a personal retrospective. Photosynth Res 76(1–3):35–52
Belyaeva OB ( 2003 ) Studies of chlorophyll biosynthesis in Russia. Photosynth Res 76(1–3):405–411
Black CC, Osmond CB ( 2003 ) Crassulacean acid metabolism photosynthesis: ‘working the night shift.’ Photosynth Res 76(1–3):329–341
Bogorad L ( 2003 ) Photosynthesis research: advances through molecular biology—the beginnings, 1975–1980s and on. Photosynth Res 76(1–3):13–33
Borisov A ( 2003 ) The beginnings of research on biophysics of photosynthesis and initial contributions made by Russian scientists to its development. Photosynth Res 76(1–3):413–426
Daldal F, Deshmukh M, Prince RC ( 2003 ) Membrane-anchored cytochrome c as an electron carrier in photosynthesis and respiration: past, present and future of an unexpected discovery. Photosynth Res 76(1–3):127–134
Delosme R ( 2003 ) On some aspects of photosynthesis revealed by photoacoustic studies: a critical evaluation. Photosynth Res 76(1–3):289–301
Demmig-Adams B ( 2003 ) Linking the xanthophyll cycle with thermal energy dissipation. Photosynth Res 76(1–3):73–80
Govindjee, Beatty JT, Gest H ( 2003 ) Celebrating the millennium—historical highlights of photosynthesis research, part 2. Photosynth Res 76(1–3):1–11
Grossman AR ( 2003 ) A molecular understanding of complementary chromatic adaptation. Photosynth Res 76(1–3):207–215
Gupta RS ( 2003 ) Evolutionary relationships among photosynthetic bacteria. Photosynth Res 76(1–3):173–183
Joliot P ( 2003 ) Period-four oscillations of the flash-induced oxygen formation in photosynthesis. Photosynth Res 76(1–3):65–72
Joliot P, Joliot A ( 2003 ) Excitation transfer between photosynthetic units: the 1964 experiment. Photosynth Res 76(1–3):241–245
Katoh S ( 2003 ) Early research on the roles of plastocyanin in photosynthesis. Photosynth Res 76(1–3):255–261
Klimov VV ( 2003 ) Discovery of pheophytin function in the photosynthetic energy conversion as the primary electron acceptor of photosystem II. Photosynth Res 76(1–3):247–253
Krasnovsky AA Jr ( 2003 ) Chlorophyll isolation, structure and function: major landmarks of the early history of research in the Russian empire and the Soviet Union. Photosynth Res 76(1–3):389–403
Kuang T-Y, Xu C, Li L-B, Shen Y-K ( 2003 ) Photosynthesis research in the People’s Republic of China. Photosynth Res 76(1–3):451–458
Madigan MT ( 2003 ) Anoxygenic phototrophic bacteria from extreme environments. Photosynth Res 76(1–3):157–171
Meyer TE, Cusanovich MA ( 2003 ) Discovery and characterization of electron transfer proteins in the photosynthetic bacteria. Photosynth Res 76(1–3):111–126
Miyachi S, Iwasaki I, Shiraiwa Y ( 2003 ) Historical perspective on microalgal and cyanobacterial acclimation to low- and extremely high-CO 2 conditions. Photosynth Res 77(2–3):139–153
Ogawa T ( 2003 ) Physical separation of chlorophyll–protein complexes. Photosynth Res 76(1–3):227–232
Ogren WL ( 2003 ) Affixing the O to rubisco: discovering the source of photorespiratory glycolate and its regulation. Photosynth Res 76(1–3):53–63
Ormerod J ( 2003 ) ‘Every dogma has its day’: a personal look at carbon metabolism in photosynthetic bacteria. Photosynth Res 76(1–3):135–143
Papageorgiou GC ( 2003 ) Photosynthesis research in Greece: a historical snapshot (1960–2001). Photosynth Res 76(1–3):427–433
Parson WW ( 2003 ) Electron donors and acceptors in the initial steps of photosynthesis in purple bacteria: a personal account. Photosynth Res 76(1–3):81–92
Raghavendra AS, Sane PV, Mohanty P ( 2003 ) Photosynthesis research in India: transition from yield physiology into molecular biology. Photosynth Res 76(1–3):435–450
Renger G ( 2003 ) Apparatus and mechanism of photosynthetic oxygen evolution: a personal perspective. Photosynth Res 76(1–3):269–288
Satoh K ( 2003 ) The identification of the photosystem II reaction center: a personal story. Photosynth Res 76(1–3):233–240
Schröder WP, Kieselbach T ( 2003 ) Update on chloroplast proteomics. Photosynth Res 78(3):181–193
Seibert M, Wasielewski MR ( 2003 ) The isolated photosystem II reaction center: first attempts to directly measure the kinetics of primary charge separation. Photosynth Res 76(1–3):263–268
Staehelin LA ( 2003 ) Chloroplast structure: from chlorophyll granutes to supra-molecular architecture of thylakoid membranes. Photosynth Res 76(1–3):185–196
Sugiura M ( 2003 ) History of chloroplast genomics. Photosynth Res 76(1–3):371–377
Tandeau de Marsac N ( 2003 ) Phycobiliproteins and phycobilisomes: the early observations. Photosynth Res 76(1–3):197–205
Vass I ( 2003 ) The history of photosynthetic thermoluminescence. Photosynth Res 76(1–3):303–318
Vernon LP ( 2003 ) Photosynthesis and the Charles F Kettering research laboratory. Photosynth Res 76(1–3):379–388
Walker DA ( 2003 ) Chloroplasts in envelopes: CO 2 fixation by fully functional intact chloroplasts. Photosynth Res 76(1–3):319–327
Allen JF ( 2002 ) Plastoquinone redox control of chloroplast thylakoid protein phosphorylation and distribution of excitation energy between photosystems: discovery, background, implication. Photosynth Res 73(1–3):139–148
Amesz J, Neerken S ( 2002 ) Excitation energy trapping in anoxygenic photosynthetic bacteria. Photosynth Res 73(1–3):73–81
Anderson JM ( 2002 ) Changing concepts about the distribution of photosystems I and II between grana-appressed and stroma-exposed thylakoid membranes. Photosynth Res 73(1–3):157–164
Beatty JT ( 2002 ) On the natural selection and evolution of the aerobic phototrophic bacteria. Photosynth Res 73(1–3):109–114
Bennoun P ( 2002 ) The present model for chlororespiration. Photosynth Res 73(1–3):273–277
Benson AA ( 2002 ) Following the path of carbon in photosynthesis: a personal story. Photosynth Res 73(1–3):29–49
Brody SS ( 2002 ) Fluorescence lifetime, yield, energy transfer and spectrum in photosynthesis, 1950–1960. Photosynth Res 73(1–3):127–132
Buchanan BB, Schürmann P, Wolosiuk RA, Jacquot J-P ( 2002 ) The ferredoxin/thioredoxin system: from discovery to molecular structures and beyond. Photosynth Res 73(1–3):215–222
Clayton RK ( 2002 ) Research on photosynthetic reaction centers from 1932 to 1987. Photosynth Res 73(1–3):63–71
Delosme R, Joliot P ( 2002 ) Period four oscillations in chlorophyll a fluorescence. Photosynth Res 73(1–3):165–168
Drews G, Niederman RA ( 2002 ) Membrane biogenesis in anoxygenic photosynthetic prokaryotes. Photosynth Res 73(1–3):87–94
Gest H ( 2002 ) Photosynthesis and phage: early studies on phosphorus metabolism in photosynthetic microorganisms with 32 P, and how they led to the serendipic discovery of 32 P-decay suicide of bacteriophage. Photosynth Res 74(3):331–339
Govindjee, Krogmann DW ( 2002 ) A list of personal perspectives with selected quotations, along with lists of tributes, historical notes, Nobel and Kettering awards related to photosynthesis. Photosynth Res 73(1–3):11–20
Govindjee, Sestak Z, Peters WR ( 2002 ) The early history of “Photosynthetica”, “Photosynthesis Research”, and their publishers. Photosynthetica 40(1):1–11
Hatch MD ( 2002 ) C 4 photosynthesis: discovery and resolution. Photosynth Res 73(1–3):251–256
Heber U ( 2002 ) Irrungen, Wirrungen? The Mehler reaction in relation to cyclic electron transport in C3 plants. Photosynth Res 73(1–3):223–231
Heldt H-W ( 2002 ) Three decades in transport business: studies of metabolite transport in chloroplasts—a personal perspective. Photosynth Res 73(1–3):265–272
Homann PH ( 2002 ) Chloride and calcium in photosystem II: from effects to enigma. Photosynth Res 73(1–3):169–175
Jagendorf AT ( 2002 ) Photophosphorylation and the chemiosmotic perspective. Photosynth Res 73(1–3):233–241
Kaplan S ( 2002 ) Photosynthesis genes and their expression in Rhodobacter sphaeroides 2.4.1: a tribute to my students and associates. Photosynth Res 73(1–3):95–108
Ke B ( 2002 ) P430: a retrospective, 1971–2001. Photosynth Res 73(1–3):207–214
de Kouchkovsky Y ( 2002 ) The laboratory of photosynthesis and its successors at Gif-sur-Yvette, France. Photosynth Res 73(1–3):295–303
Lewin RA ( 2002 ) Prochlorophyta—a matter of class disctinctions. Photosynth Res 73(1–3):59–61
Ludden PW, Roberts GP ( 2002 ) Nitrogen fixation by photosynthetic bacteria. Photosynth Res 73(1–3):115–118
Marrs BL ( 2002 ) The early history of the genetics of photosynthetic bacteria: a personal account. Photosynth Res 73(1–3):55–58
Mimuro M ( 2002 ) Visualization of excitation energy transfer processes in plants and algae. Photosynth Res 73(1–3):133–138
Nelson N, Ben-Shem A ( 2002 ) Photosystem I reaction center: past and future. Photosynth Res 73(1–3):193–206
Pearlstein RM ( 2002 ) Photosynthetic exciton theory in the 1960s. Photosynth Res 73(1–3):119–126
Porra RJ ( 2002 ) The chequered history of the development and use of simultaneous equations for the accurate determination of chlorophylls a and b . Photosynth Res 73(1–3):149–156
Portis AR Jr, Salvucci ME ( 2002 ) The discovery of rubisco activase—yet another story of serendipity. Photosynth Res 73(1–3):257–264
Rochaix J-D ( 2002 ) The three genomes of Chlamydomonas. Photosynth Res 73(1–3):285–293
Shestakov SV ( 2002 ) Gene-targeted and site-directed mutagenesis of photosynthesis genes in Cyanobacteria. Photosynth Res 73(1–3):279–284
Stemler AJ ( 2002 ) The bicarbonate effect, oxygen evolution, and the shadow of Otto Warburg. Photosynth Res 73(1–3):177–183
Van Rensen JJS ( 2002 ) Role of bicarbonate at the acceptor side of photosystem II. Photosynth Res 73(1–3):185–192
Verméglio A ( 2002 ) The two-electron gate in photosynthetic bacteria. Photosynth Res 73(1–3):83–86
Walker DA ( 2002 ) ‘And whose bright presence’—an appreciation of Robert Hill and his reaction. Photosynth Res 73(1–3):51–54
Wildman SG ( 2002 ) Along the trail from fraction I protein to rubisco (ribulose bisphosphate carboxylase-oxygenase). Photosynth Res 73(1–3):243–250
Govindjee ( 2000 ) Milestones in photosynthesis research. In: Younis M, Pathre U, Mohanty P (eds) Probing photosynthesis. Taylor & Francis, London, pp 9–39
Govindjee ( 1999 ) On the requirement of minimum number of four versus eight quanta of light for the evolution of one molecule of oxygen in photosynthesis: a historical note. Photosynth Res 59(2–3):249–254
Feher G ( 1998 ) Light reflections III. Photosynth Res 55(2–3):375–378
Dutton HJ ( 1997 ) Carotenoid-sensitized photosynthesis: quantum efficiency, fluorescence and energy transfer. Photosynth Res 52(2):175–185
Walker DA ( 1997 ) ‘Tell me where all past years are.’ Photosynth Res 51(1):1–26
Arnon DI ( 1995 ) Divergent pathways of photosynthetic electro transfer: the autonomous oxygenic and anoxygenic photosystems. Photosynth Res 46(1–2):47–71
Epstein E ( 1995 ) Photosynthesis, inorganic plant nutrition, solutions, and problems. Photosynth Res 46(1–2):37–39
Frenkel AW ( 1995 ) Photosynthetic phosphorylation. Photosynth Res 46(1–2):73–77
Jukes TH ( 1995 ) Mineral nutrition of plants. Photosynth Res 46(1–2):13–15
Trebst A, Depka B ( 1995 ) Polyphenol oxidase and photosynthesis research. Photosynth Res 46(1–2):41–44
Walker DA ( 1995 ) One thing leading to another. Photosynth Res 46(1–2):45–46
Whatley FR ( 1995 ) Photosynthesis by isolated chloroplasts: the early work in Berkeley. Photosynth Res 46(1–2):17–26
Myers J ( 1994 ) The 1932 experiments. Photosynth Res 40(3):303–310
Cheniae GM ( 1993 ) A recollection of the development of the Kok-Joliot model for photosynthetic oxygen evolution. Photosynth Res 38(3):225–227
Gest H ( 1993 ) History of concepts of the comparative biochemist of oxygenic and anoxygenic photosyntheses. Photosynth Res 35(1):87–96
Huzisige H, Ke B ( 1993 ) Dynamics of the history of photosynthesis research. Photosynth Res 35(1):185–209
Hill DJ ( 1992 ) An overlooked symbiosis. Photosynth Res 34(3):339–340
Kooten O, Snel JFH ( 1990 ) The use of chlorophyll fluorescence nomenclature in plant stress physiology. Photosynth Res 25(3):147–150
Gest H ( 1988 ) Sun-beams, cucumbers, and purple bacteria. Historical milestones in early studies of photosynthesis revisited. Photosynth Res 19(3):287–308
Govindjee ( 1988 ) Growth of Photosynthesis Research: 1980–1986. Photosynth Res 15(3):193–194
5 V Special issues
Cogdell R, Mullineaux C (eds) ( 2008 ) Photosynthetic light harvesting. Photosynth Res 95(2–3):117–371
Gunner MR (ed) ( 2008 ) Computational analysis of photosynthetic systems. Photosynth Res 97(1):1–114
Allakhverdiev SI, Klimov VV, Nagata T, Nixon P, Shen J-R (eds) ( 2008 ) Recent perspectives of photosystem II: structure, function and dynamics—in honour of Kimiyuki Satoh and Thomas Wydrzynski. Photosynth Res 98(1–3):1–700
Buchanan BB, Douce R, Lichtenthaler HK (eds) ( 2007 ) A tribute to Andrew A. Benson. Photosynth Res 92(2):143–271
Putnam-Evans C, Barry B (eds) ( 2007 ) Photosynthetic water oxidation. Photosynth Res 92(3):273–425
Eaton-Rye JJ (ed) ( 2007 ) Govindjee special issue: part A—celebrating Govindjee's 50 years in photosynthesis research and his 75th birthday. Photosynth Res 93(1–3):1–244
Eaton-Rye JJ (ed) ( 2007 ) Govindjee special issue: part B—celebrating Govindjee's 50 years in photosynthesis research and his 75th birthday. Photosynth Res 94(2–3):153–466
Carpentier R, Allakhverdiev SI, Aro EM, Brudvig G, Diner BA, Knaff DB, Satoh K, Wydrzynski TJ (eds) ( 2005 ) Photosynthesis and the post-genomic era: from biophysics to molecular biology, a path in the research of photosystem II. Photosynth Res 84(1–3):1–372
Allen JP, Knaff DB (eds) ( 2004 ) Structural biology of proteins from photosynthetic organisms. Photosynth Res 81(3):205–348
Buchanan BB, Knaff DB, Jacquot JP (eds) ( 2004 ) Plant thioredoxins and related proteins. Photosynth Res 79(3):225–373
Sayre RT, Hippler M (eds) ( 2004 ) Molecular genomics of the Chlamydomonas chloroplast. Photosynth Res 82(3):201–354
Burnap RL, Vermaas WFJ (eds) ( 2003 ) Proteomics. Photosynth Res 78(3):179–302
Beale SI (ed) ( 2002 ) Tetrapyrrole photoreceptors in photosynthetic organisms. Photosynth Res 74(2):95–233
Govindjee, Gest H (eds) ( 2002 ) Celebrating the millennium—historical highlights of photosynthesis research, Part 1. Photosynth Res 73(1–3):1–308
Miller M, Aartsma TJ, Blankenship RE (eds) ( 2002 ) Special issue in honour of Jan Amesz: green and heliobacteria. Photosynth Res 71(1–2):vii+ 1–183
Berry JA, Field CB, Grossman AR (eds) ( 2001 ) Special issue in honour of Olle Björkman: plants and their light environment. Photosynth Res 67(1–2):1–156
Mackenzie C, Kaplan S (eds) ( 2001 ) Genomics. Photosynth Res 70(1):1–127
Bassi R, Cinque G (eds) ( 2001 ) Tetrapyrrole photoreceptors in plants and algae. Photosynth Res 64(2–3):iii+ 1–280
Kramer DM (ed) ( 2000 ) Emerging techniques in Photosynthesis Research. Photosynth Res 66(1–2):1–158
Breton J, Nabedryk E, Verméglio A (eds) ( 1998 ) Reaction centers of photosynthetic purple bacteria: structure, spectroscopy, dynamics. Photosynth Res 55(2–3):117–384
Bauer CE (ed) ( 1997 ) Symposium in print: diversity, genetics, and physiology of photosynthetic prokaryotes in honor of the 75th birthday of Howard Gest. Photosynth Res 53(1):1–79
Mimuro M, Gantt E, Bryant DA (eds) ( 1997 ) Molecular approaches to light acclimation from Cyanobacteria to higher plants. Photosynth Res 53(2–3):81–266
Govindjee, Knox RS, Amesz J (eds) ( 1996 ) Special issue dedicated to William A Arnold: photosynthetic unit: antenna and reaction centers. Photosynth Res 48(1–2):1–319
Cogdell R, Nechusthtai R, Malkin R (eds) ( 1995 ) Structure, function and biogenesis of chlorophyll–protein complexes. Photosynth Res 44(1–2):1–219
Melis A, Buchanan BB (eds) ( 1995 ) A tribute to Daniel I Arnon. Photosynth Res 46(1–2):1–377
Falkowski PG, Long SP, Edwards GE (eds) ( 1994 ) Photosynthesis and global changes in the environment. Photosynth Res 39(3):207–495
Olson JM, Amesz J, Ormerod JG, Blankenship RE (eds) ( 1994 ) Green and heliobacteria. Photosynth Res 41(1):1–294
Govindjee, Renger G (eds) ( 1993 ) How plants and Cyanobacteria make oxygen: 25 years of period four oscillations. Photosynth Res 38(3):211–482
Hartman H (Ed) ( 1992 ) Photosynthesis and the origin of life. Photosynth Res 33(2):73–176
Rich PR (ed) ( 1992 ) Robin Hill. Photosynth Res 34(3):319–488
Blankenship RE, Amesz J, Holten D, Jortner J (eds) ( 1989 ) Tunneling processes in photosynthesis. Part 1. Photosynth Res 22(1):1–122
Govindjee, Bohnert HJ, Bottomley W, Bryant DA, Mullet JE, Ogren WL, Pakrasi H, Somerville CR (eds) ( 1988 ) Molecular biology of photosynthesis 1. Photosynth Res 16(1–2):5–186
Govindjee, Bohnert HJ, Bottomley W, Bryant DA, Mullet JE, Ogren WL, Pakrasi H, Somerville CR (eds) ( 1988 ) Molecular biology of photosynthesis 2. Photosynth Res 17(1–2):5–194
Govindjee, Bohnert HJ, Bottomley W, Bryant DA, Mullet JE, Ogren WL, Pakrasi H, Somerville CR (eds) ( 1988 ) Molecular biology of photosynthesis 3. Photosynth Res 18(1–2):5–262
Govindjee, Bohnert HJ, Bottomley W, Bryant DA, Mullet JE, Ogren WL, Pakrasi H, Somerville CR (eds) ( 1988 ) Molecular biology of photosynthesis 4. Photosynth Res 19(1–2):5–204
Amesz J, Hoff AJ, van Gorkom HJ (eds) ( 1986 ) Double special issue dedicated to Professor Louis NM Duysens on the occasion of his retirement. Photosynth Res 9(1–2):vii+ 1–283
6 VI Conferences
Prasil O, Suggett DJ, Cullen JJ, Babin M, Govindjee ( 2008 ) Aquafluo 2007: chlorophyll fluorescence in aquatic sciences, an international conference held in Nové Hrady. Photosynth Res 95(1):111–115
Blankenship RE ( 2007 ) 2007 Awards of the International Society of Photosynthesis Research (ISPR). Photosynth Res 94(2–3):179–181
Govindjee, Telfer A ( 2007 ) Six young research investigators were honored at an international conference in Russia. Photosynth Res 92(1):139–141
Govindjee, Yoo H ( 2007 ) The International Society of Photosynthesis Research (ISPR) and its associated international congress on photosynthesis (ICP): a pictorial report. Photosynth Res 91(2–3):95–106
Govindjee, Rutherford AW, Britt RD ( 2007 ) Four young research investigators were honored at the 2006 Gordon research conference on photosynthesis. Photosynth Res 92(1):137–138
Aro EM, Golbeck JH, Osmond B ( 2006 ) Message from the International Society of Photosynthesis Research (ISPR). Photosynth Res 89(1):7–9
Foyer CH ( 2006 ) Photosynthesis coming of age to meet the needs of the 21st century: an invitation to the 14th international congress on photosynthesis research in 2007. Photosynth Res 89(1):3–6
Govindjee, Knaff D ( 2006 ) International photosynthesis congresses (1968–2007). Photosynth Res 89(1):1–2
Govindjee ( 2004 ) A list of photosynthesis conferences and of edited books in photosynthesis. Photosynth Res 80(1–3):447–460
Rurainski HJ ( 2004 ) The conference at Airlie House in 1963. Photosynth Res 80(1–3):439–446
Govindjee, Beatty JT, Gest H (eds) ( 2003 ) Celebrating the Golden jubilee of the 1952 conference of photosynthesis (Gatlinburg, Tennessee, USA). Photosynth Res 76(1–3): see a photograph, p. vii
Renger G ( 1987 ) Conference report on the Japan/US-binational seminar on “energy conversion: photochemical reaction centers and oxygen evolving complexes of plant photosynthesis.” Photosynth Res 13(3):261–268
Adir N, Zer H, Shochat S, Ohad I (2003) Photoinhibition—a historical perspective. Photosynth Res 76(1–3):343–370
Article PubMed CAS Google Scholar
Aflalo C, Baum H, Chipman DM, McCarty RE, Strotmann H (1997) Noun Shavit (1930–1997). Photosynth Res 54(3):165–167
Article CAS Google Scholar
Akazawa T (1994) Reminiscences, collaborations and reflections. Photosynth Res 46(1–2):93–113
Article Google Scholar
Albertsson P-A (2003) The contribution of photosynthetic pigments to the development of biochemical separation methods: 1990–1980. Photosynth Res 76(1–3):217–225
Allakhverdiev SI, Klimov VV, Nagata T, Nixon P, Shen J-R (eds) (2008) Recent perspectives of photosystem II: structure, function and dynamics—in honour of Kimiyuki Satoh and Thomas Wydrzynski. Photosynth Res 98(1–3):1–700
Google Scholar
Allen JF (2002) Plastoquinone redox control of chloroplast thylakoid protein phosphorylation and distribution of excitation energy between photosystems: discovery, background, implication. Photosynth Res 73(1–3):139–148
Allen JP (2004) My daily constitutional in Martinsried. Photosynth Res 80(1–3):157–163
Allen JP, Knaff DB (eds) (2004) Structural biology of proteins from photosynthetic organisms. Photosynth Res 81(3):205–348
Amesz J, Neerken S (2002) Excitation energy trapping in anoxygenic photosynthetic bacteria. Photosynth Res 73(1–3):73–81
Amesz J, Hoff AJ, Van Gorkom HJ (eds) (1986) Current topics in photosynthesis—double issue dedicated to Professor Louis N. M. Duysens on the occasion of his retirement. Photosynth Res 9:1–283
Amesz J, Hoff AJ, van Gorkom HJ (eds) (1986) Double special issue dedicated to professor Louis NM Duysens on the occasion of his retirement. Photosynth Res 9(1–2):vii+ 1–283
Anderson JM (1990) David John Goodchild. Photosynth Res 24(2):115–116
Anderson MC (1993) Robin Hill, FRS: a Cambridge neighbor’s appreciation of a great man and his hemispherical camera. Photosynthetica 28:321–322
Anderson JM (2002) Changing concepts about the distribution of photosystems I and II between grana-appressed and stroma-exposed thylakoid membranes. Photosynth Res 73(1–3):157–164
Anderson JM (2007) Thylakoid membrane landscape in the sixties: a tribute to Andrew Benson. Photosynth Res 92(2):193–197
Andley UP, Velagaleti PNR, Sen A, Tripathy BC (2005) Gauri Shankar Singhal (1933–2004): a photochemist, a photobiologist, a great mentor and a generous friend. Photosynth Res 85(2):145–148
Armitage JP, Hellingwerf KJ (2003) Light-induced behavioral responses (‘phototaxis’) in prokaryotes. Photosynth Res 76(1–3):145–155
Arnold WA (1991) Experiments. Photosynth Res 27(2):73–82
Arnon DI (1995) Divergent pathways of photosynthetic electro transfer: the autonomous oxygenic and anoxygenic photosystems. Photosynth Res 46(1–2):47–71
Aro EM, Golbeck JH, Osmond B (2006) Message from the International Society of Photosynthesis Research (ISPR). Photosynth Res 89(1):7–9
Asana RD (1961) Prof. R.H. Dastur, O.B.E. Nature 192:1128
Bannister TT (1972) The careers and contributions of Eugene Rabinowitch. Biophys J 12(7):707–718
Barber J (2004) Engine of life and big bang of evolution: a personal perspective. Photosynth Res 80(1–3):137–155
Barry BA (2006) Ilya Vassiliev (January 12, 1959–August 10, 2005). Photosynth Res 87(3):245–246
Bassham JA (2003) Mapping the carbon reduction cycle: a personal retrospective. Photosynth Res 76(1–3):35–52
Article PubMed Google Scholar
Bassi R, Cinque G (eds) (2001) Tetrapyrrole photoreceptors in plants and algae. Photosynth Res 64(2–3):iii+ 1–280
Bauer CE (ed) (1997) Symposium in print: diversity, genetics, and physiology of photosynthetic prokaryotes in honor of the 75th birthday of Howard Gest. Photosynth Res 53(1):1–79
Bauer C (2004) Regulation of photosystem synthesis in Rhodobacter capsulatus . Photosynth Res 80(1–3):353–360
Beale SI (ed) (2002) Tetrapyrrole photoreceptors in photosynthetic organisms. Photosynth Res 74(2):95–233
Beatty JT (2002) On the natural selection and evolution of the aerobic phototrophic bacteria. Photosynth Res 73(1–3):109–114
Belyaeva OB (2003) Studies of chlorophyll biosynthesis in Russia. Photosynth Res 76(1–3):405–411
Bendall DS (2004) The unfinished story of cytochrome f . Photosynth Res 80(1–3):265–276
Bendall DS, Walker DA (1991) Robert (Robin) Hill (1899–1991). Photosynth Res 30(1):1–5
Benning C (2007) Questions remaining in sulfolipid biosynthesis: a historical perspective. Photosynth Res 92(2):199–203
Bennoun P (2002) The present model fro chlororespiration. Photosynth Res 73(1–3):273–277
Benson AA (2002) Paving the path. Annu Rev Plant Biol 53:1–25
Benson AA (2002) Following the path of carbon in photosynthesis: a personal story. Photosynth Res 73(1–3):29–49
Berg S (1998) Seikichi Izawa (1926–1997). Photosynth Res 58(1):1–4
Berry JA, Field CB, Grossman AR (eds) (2001) Special issue in honour of Olle Björkman: plants and their light environment. Photosynth Res 67(1–2):1–156
Bishop NI (1986) Warren L Butler; a tribute to a friend and fellow scientist. Photosynth Res 10(3):147–149
Björn LO, Sundqvist C, Öquist G (2007) A tribute to Per Halldal (1922–1986), a Norwegian photobiologist in Sweden. Photosynth Res 92(1):7–11
Black CC Jr (2008) Martin Gibbs (1922–2006): pioneer of 14 C research, sugar metabolism & photosynthesis; vigilant editor-in-chief of Plant Physiology ; sage educator; and humanistic mentor. Photosynth Res 95(1):1–10
Black CC, Govindjee (2008) Martin Gibbs and the peaceful uses of nuclear radiation, 14 C. Photosynth Res 99(1):63–80
Black CC, Mayne BC (2006) Allan H Brown (1917–2004), editor and educator: a career of fascination with the biological roles of O 2 in terrestrial life and possibly in extraterrestrial life. Photosynth Res 87(2):159–163
Black CC, Osmond CB (2003) Crassulacean acid metabolism photosynthesis: ‘working the night shift’. Photosynth Res 76(1–3):329–341
Blankenship RE (2007) 2007 Awards of the International Society of Photosynthesis Research (ISPR). Photosynth Res 94(2–3):179–181
Blankenship RE, Amesz J, Holten D, Jortner J (eds) (1989) Tunneling processes in photosynthesis. Part 1. Photosynth Res 22(1):1–122
Blankenship RE, Amesz J, Holten D, Jortner J (eds) (1989) Tunneling processes in photosynthesis—dedicated to Donald DeVault. Part 2. Photosynth Res 22:173–301
Block MA, Douce R, Joyard J, Rolland N (2007) Chloroplast envelop membranes: a dynamic interface between plastids and the cytosol. Photosynth Res 92(2):225–244
Bogorad L (2003) Photosynthesis research: advances through molecular biology—the beginnings, 1975–1980s and on. Photosynth Res 76(1–3):13–33
Borisov A (2003) The beginnings of research on biophysics of photosynthesis and initial contributions made by Russian scientists to its development. Photosynth Res 76(1–3):413–426
Brand JJ, Krogman DW, Patterson CO (2008) Jack Edgar Myers (1913–2006), an algal physiologist par excellence . Photosynth Res 96(1):9–14
Breton J, Nabedryk E, Verméglio A (eds) (1998) Reaction centers of photosynthetic purple bacteria: structure, spectroscopy, dynamics. Photosynth Res 55(2–3):117–384
Briggs GE (1948) F.F. Blackman (1866–1947). Obit Notices Fellows R Soc 5(16):651–658
Britt RD, Sauer K, Yachandra VK (2000) Remembering Melvin P Klein. Photosynth Res 65(3):201–206
Brody SS (1995) We remember Eugene. Photosynth Res 43(1):67–74
Brody SS (2002) Fluorescence lifetime, yield, energy transfer and spectrum in photosynthesis, 1950–1960. Photosynth Res 73(1–3):127–132
Bruce D, Sauer K (2005) John Biggins (1936–2004): his ingenuity, tenacity and humor; no-nonsense science with a big heart. Photosynth Res 85(3):261–265
Buchanan BB (1995) Introduction: the life of Daniel I Arnon. Photosynth Res 46:3–6
Buchanan BB (2004) Peter Schürmann. Photosynth Res 79(3):227–228
Buchanan BB (2007) Thioredoxin: an unexpected meeting place. Photosynth Res 92(2):145–148
Buchanan BB, Carlson D (1995) Daniel I Arnon: portrayal of a research career. Photosynth Res 46:7–12
Buchanan BB, Tagawa K (1995) Perspective on Daniel I Arnon’s contributions to research, 1960–1994. Photosynth Res 46(1–2):27–35
Buchanan BB, Schürmann P, Wolosiuk RA, Jacquot J-P (2002) The ferredoxin/thioredoxin system: from discovery to molecular structures and beyond. Photosynth Res 73(1–3):215–222
Buchanan BB, Knaff DB, Jacquot JP (eds) (2004) Plant thioredoxins and related proteins. Photosynth Res 79(3):225–373
Buchanan BB, Douce R, Lichtenthaler HK (2007) Andrew A Benson. Photosynth Res 92(2):143–144
Buchanan BB, Douce R, Lichtenthaler HK (eds) (2007) A tribute to Andrew A. Benson. Photosynth Res 92(2):143–271
Burnap RL, Vermaas WFJ (eds) (2003) Proteomics. Photosynth Res 78(3):179–302
Calvin M (1989) Forty years of photosynthesis and related activities. Photosynth Res 21(1):1–16
Camm EL, Green BR (2004) How the chlorophyll-proteins got their names. Photosynth Res 80(1–3):189–196
Cammack R (2006) K. Krishna Rao—a lifetime study of ferredoxins and solar hydrogen. Photosynth Res 90(2):97–99
Carpentier R, Allakhverdiev SI, Aro EM, Brudvig G, Diner BA, Knaff DB, Satoh K, Wydrzynski TJ (eds) (2005) Photosynthesis and the post-genomic era: from biophysics to molecular biology, a path in the research of photosystem II. Photosynth Res 84(1–3):1–372
Castenholz RW (1994) William R Sistrom (1927–1993). Photosynth Res 42(3):167–168
Champigny ML (1992) Alexis Moyse (1912–1991). Photosynthetica 26:161–162
Chance B (1991) Optical method. Annu Rev Biophys Biophys Chem 20:1–28
Chance B (2004) The stopped-flow method and chemical intermediates in enzyme reactions—a personal essay. Photosynth Res 80(1–3):387–400
Cheniae GM (1993) A recollection of the development of the Kok-Joliot model for photosynthetic oxygen evolution. Photosynth Res 38(3):225–227
Clayton RK (1988) Memories of many lives. Photosynth Res 19(3):205–224
Clayton RK (2002) Research on photosynthetic reaction centers from 1932 to 1987. Photosynth Res 73(1–3):63–71
Cogdell R (1996) Philip Thornber (1934–1996). Photosynth Res 50(1):1–3
Cogdell R, Mullineaux C (eds) (2008) Photosynthetic light harvesting. Photosynth Res 95(2–3):117–371
Cogdell R, Nechusthtai R, Malkin R (eds) (1995) Structure, function and biogenesis of chlorophyll–protein complexes. Photosynth Res 44(1–2):1–219
Cogdell RJ, Hashimoto H, Gardiner AT (2004) Purple bacterial light-harvesting complexes: from dreams to structures. Photosynth Res 80(1–3):173–179
Conn EE, Pistorius EK, Solomonson LP (2005) Remembering Birgit Vennesland (1913–2001), a great biochemist. Photosynth Res 83(1):11–16
Cramer WA (2004) Ironies in photosynthetic electron transport: a personal perspective. Photosynth Res 80(1–3):293–305
Crofts A (1993) Peter Mitchell (1920–1992). Photosynth Res 35(1):1–4
Crofts AR (2004) The Q-cycle—a personal perspective. Photosynth Res 80(1–3):223–243
Daldal F, Deshmukh M, Prince RC (2003) Membrane-anchored cytochrome c as an electron carrier in photosynthesis and respiration: past, present and future of an unexpected discovery. Photosynth Res 76(1–3):127–134
de Kouchkovsky Y (2002) The laboratory of photosynthesis and its successors at Gif-sur-Yvette, France. Photosynth Res 73(1–3):295–303
de Kouchkovsky Y, Cerovic ZG (2005) Jean-Marie Briantais (1936–2004), a friend and a champion of interactive and integrative research. Photosynth Res 83(1):1–3
Delosme R (2003) On some aspects of photosynthesis revealed by photoacoustic studies: a critical evaluation. Photosynth Res 76(1–3):289–301
Delosme R, Joliot P (2002) Period four oscillations in chlorophyll a fluorescence. Photosynth Res 73(1–3):165–168
Demmig-Adams B (2003) Linking the xanthophyll cycle with thermal energy dissipation. Photosynth Res 76(1–3):73–80
Devault D (1989) Tunneling enters biology. Photosynth Res 22(1):5–10
Dilley RA (2004) On why thylakoids energize ATP formation using either delocalized or localized proton gradients—a Ca 2+ mediated role in thylakoid stress responses. Photosynth Res 80(1–3):245–263
Drews G (1996) Forty-five years of developmental biology of photosynthetic bacteria. Photosynth Res 48:325–352
Drews G (2005) Contributions of Theodor Wilhelm Engelmann on phototaxis, chemotaxis, and photosynthesis. Photosynth Res 83(1):25–34
Drews G, Niederman RA (2002) Membrane biogenesis in anoxygenic photosynthetic prokaryotes. Photosynth Res 73(1–3):87–94
Dutton HJ (1997) Carotenoid-sensitized photosynthesis: quantum efficiency, fluorescence and energy transfer. Photosynth Res 52(2):175–185
Duysens LNM (1989) The discovery of the two photosynthetic systems: a personal account. Photosynth Res 21(2):61–79
CAS Google Scholar
Duysens LNM (1996) WA Arnold’s inspiring experiments. Photosynth Res 48(1–2):25–29
Eaton-Rye JJ (2007) Celebrating Govindjee’s 50 years in Photosynthesis Research and his 75th birthday. Photosynth Res 93(1–3):1–5
Eaton-Rye JJ (2007) Snapshots of the Govindjee lab from the late 1960s to the late 1990s, and beyond. Photosynth Res 94(2–3):153–178
Eaton-Rye JJ (ed) (2007) Govindjee special issue: part A—celebrating Govindjee's 50 years in photosynthesis research and his 75th birthday. Photosynth Res 93(1–3):1–244
Eaton-Rye, JJ (ed) (2007) Govindjee special issue: part B—celebrating Govindjee's 50 years in photosynthesis research and his 75th birthday. Photosynth Res 94(2–3):153–466
Ellis RJ (2004) From chloroplasts to chaperones: how one thing led to another. Photosynth Res 80(1–3):333–343
Enami I, Shen J-R (2008) A brief introduction of Kimiyuki Satoh. Photosynth Res
Epstein E (1995) Photosynthesis, inorganic plant nutrition, solutions, and problems. Photosynth Res 46(1–2):37–39
Fajer J (2004) Chlorophyll chemistry before and after crystals of photosynthetic reaction centers. Photosynth Res 80(1–3):165–172
Falkowski PG, Long SP, Edwards GE (eds) (1994) Photosynthesis and global changes in the environment. Photosynth Res 39(3):207–495
Feher G (1998) Three decades of research in bacterial photosynthesis and the road leading to it: a personal account. Photosynth Res 55(1):1–40
Feher G (1998) Light reflections III. Photosynth Res 55(2–3):375–378
Feher G (2002) My road to biophysics: picking flowers on the path to photosynthesis. Annu Rev Biophys Biomol Struct 31:1–44
Fischer-Zeh K (2000) Helmut Metzner (1925–1999). Photosynth Res 63(3):191–194
Fock H (1976) Professor Dr. Karl Egle (1912–1975). Photosynthetica 10: unnumbered pages (in German)
Fork DC (1996) Charles Stacy French: a tribute. Photosynth Res 49(1):91–101
Fork DC (1996) Charles Stacy French (1907–1995). Photosynthetica 33:1–6
Forti G (1999) Personal recollections of 40 years in photosynthesis research. Photosynth Res 60(2–3):99–110
Forti G, Agostiano A, Barbato R, Bassi R, Brugnoli E, Finazzi G, Garlaschi FM, Jennings RC, Melandri BA, Trotta M, Venturoli G, Zanetti G, Zannoni D, Zucchelli G (2006) Photosynthesis research in Italy: a review. Photosynth Res 88(3):211–240
Foyer CH (2006) Photosynthesis coming of age to meet the needs of the 21st century: an invitation to the 14th international congress on photosynthesis research in 2007. Photosynth Res 89(1):3–6
Frasch WD, Sayre RT (2001) Remembering George Cheniae, who never compromised his high standards of science. Photosynth Res 70(3):245–247
French CS (1979) Fifty years of photosynthesis. Annu Rev Plant Physiol 30:1–26
Frenkel AW (1993) Recollections. Photosynth Res 35(2):103–116
Frenkel AW (1995) Photosynthetic phosphorylation. Photosynth Res 46(1–2):73–77
Fromme P, Mathis P (2004) Unraveling the photosystem I-reaction center: a history, or the sum of many efforts. Photosynth Res 80(1–3):109–124
Fujita Y (1997) A study on the dynamic features of photosystem stoichiometry: accomplishments and problems for future studies. Photosynth Res 53(2–3):83–93
Fuller RC (1999) Forty years of microbial photosynthesis research: where it came from and what it led to. Photosynth Res 62(1):1–29
Gadal P (2004) Myroslawa Miginiac-Maslow. Photosynth Res 79(3):229–230
Gaffron H (1969) Resistance to knowledge. Annu Rev Plant Physiol 20:1–40
Garab G (2000) Gábor Horváth (1944–2000). Photosynth Res 65(2):103–105
Garab G, Mustardy L, Demeter S (1987) Agnes Faludi Daniel (1929–1986). Photosynth Res 13:99–100
Gerhart D (1996) Forty-five years of developmental biology of photosynthetic bacteria. Photosynth Res 48(3):325–352
Gest H (1988) Sun-beams, cucumbers, and purple bacteria. Historical milestones in early studies of photosynthesis revisited. Photosynth Res 19(3):287–308
Gest H (1991) The legacy of Hans Molisch (1856–1937), photosynthesis savant. Photosynth Res 30(1):49–59
Gest H (1993) History of concepts of the comparative biochemist of oxygenic and anoxygenic photosyntheses. Photosynth Res 35(1):87–96
Gest H (1994) A microbiologist’s odyssey: bacterial viruses to photosynthetic bacteria. Photosynth Res 40(2):129–146
Gest H (1994) Discovery of the heliobacteria. Photosynth Res 41(1):17–21
Gest H (1997) A misplaced chapter in the history of photosynthesis research. The second publication (1796) on plant processes by Dr. Jan Ingen-Housz, MD, discoverer of photosynthesis. Photosynth Res 53:65–72
Gest H (1999) Memoir of a 1949 railway journey with photosynthetic bacteria. Photosynth Res 61(1):91–96
Gest H (2000) Bicentenary homage to Dr Jan Ingen-Housz, MD (1730–1799), pioneer of photosynthesis research. Photosynth Res 63(2):183–190
Gest H (2000) Bicentenary homage to Jan Ingen-Housz, pioneer of photosynthesis research. Photosynth Res 63:183–190
Gest H (2002) History of the word photosynthesis and evolution of its definition. Photosynth Res 73(1–3):7–10
Gest H (2002) Photosynthesis and phage: early studies on phosphorus metabolism in photosynthetic microorganisms with 32 p, and how they led to the serendipic discovery of 32 p-decay suicide of bacteriophage. Photosynth Res 74(3):331–339
Gest H (2004) Samuel Ruben’s contributions to research on photosynthesis and bacterial metabolism with radioactive carbon. Photosynth Res 80(1–3):77–83
Gest H, Blankenship RE (2004) Time line of discoveries: anoxygenic bacterial photosynthesis. Photosynth Res 80(1–3):59–70
Ghosh AK (2004) Passage of a young Indian physical chemist through the world of photosynthesis research at Urbana, Illinois, in the 1960s: a personal essay. Photosynth Res 80(1–3):427–437
Giacometti GM, Giacometti G (2006) Twenty years of biophysics of photosynthesis in Padova, Italy (1984–2005): a tale of two brothers. Photosynth Res 88(3):241–258
Gibbs M (1999) Educator and editor. Annu Rev Plant Physiol Plant Mol Biol 50:1–25
Good NE (1986) Confessions of a habitual skeptic. Annu Rev Plant Physiol 37:1–22
Goodwin J (1992) Dr Robin Hill: natural dyes. Photosynth Res 34(3):321–322
Gorham PR, Nozzolillo CG (2006) Photosynthesis research in Canada from 1945 to the early 1970s. Photosynth Res 88(1):83–100
Govindjee (1986) Publications of Warren L Butler on photosynthesis. Photosynth Res 10(3):151–161
Govindjee (1988) The discovery of chlorophyll–protein complex by Emil L. Smith during 1937–1941. Photosynth Res 16:285–289
Govindjee (1988) Growth of Photosynthesis Research: 1980–1986. Photosynth Res 15(3):193–194
Govindjee N (1999) On the requirement of minimum number of four versus eight quanta of light for the evolution of one molecule of oxygen in photosynthesis: a historical note. Photosynth Res 59(2–3):249–254
Govindjee (2000) Milestones in photosynthesis research. In: Younis M, Pathre U, Mohanty P (eds) Probing photosynthesis. Taylor & Francis, London, pp 9–39
Govindjee (2001) Calvin and Hill prizes: 2001. Photosynth Res 70(3):325–328
Govindjee (2001) Our greetings to Olle Björkman, Christopher Field, and Alexander Glazer. Photosynth Res 70(2):241–243
Govindjee (2001) Lighting the path: a tribute to Robert Emerson (1903–1959). S43-001 (6 pp); available free at http://www.publish.csiro.au/?act=view_file&file_id=SA0403744.pdf
Govindjee (2004) Robert Emerson and Eugene Rabinowitch: understanding photosynthesis. In: Hoddeson L (ed) No boundaries. University of Illinois Vignettes. University of Illinois Press, Urbana, pp 181–194
Govindjee (2004) A list of photosynthesis conferences and of edited books in photosynthesis. Photosynth Res 80(1–3):447–460
PubMed CAS Google Scholar
Govindjee (2006) Celebrating 20 years of historical papers in photosynthesis research. Photosynth Res 87(2):151–158
Govindjee (2008) Recollections of Thomas John Wydrzynski. Photosynth Res, 18 pp
Govindjee, Gest H (eds) (2002) Celebrating the millennium—historical highlights of photosynthesis research, part 1. Photosynth Res 73(1–3):1–308
Govindjee, Knaff D (2006) International photosynthesis congresses (1968–2007). Photosynth Res 89(1):1–2
Govindjee, Krogmann DW (2002) A list of personal perspectives with selected quotations, along with lists of tributes, historical notes, Nobel and Kettering awards related to photosynthesis. Photosynth Res 73(1–3):11–20
Govindjee, Krogmann D (2004) Discoveries in oxygenic photosynthesis (1727–2003): a perspective. Photosynth Res 80(1–3):15–57
Govindjee, Renger G (eds) (1993) How plants and Cyanobacteria make oxygen: 25 years of period four oscillations. Photosynth Res 38(3):211–482
Govindjee, Telfer A (2007) Six young research investigators were honored at an international conference in Russia. Photosynth Res 92(1):139–141
Govindjee, Yoo H (2007) The international society of photosynthesis research (ISPR) and its associated international congress on photosynthesis (ICP) a pictoral report. Photosynth Res 91:95–106
Govindjee, Barber J, Cramer WA, Goeddheer JHC, Lavorel J, Macelle R, Zilinskas B (eds) (1986) Excitation and electron transfer in photosynthesis—special issue—dedicated to Warren L. Butler. Photosynth Res 10:147–518
Govindjee, Bohnert HJ, Bottomley W, Bryant DA, Mullet JE, Ogren WL, Pakrasi H, Somerville CR (eds) (1988) Molecular biology of photosynthesis 1. Photosynth Res 16(1–2):5–186
Govindjee, Bohnert HJ, Bottomley W, Bryant DA, Mullet JE, Ogren WL, Pakrasi H, Somerville CR (eds) (1988) Molecular biology of photosynthesis 2. Photosynth Res 17(1–2):5–194
Govindjee, Bohnert HJ, Bottomley W, Bryant DA, Mullet JE, Ogren WL, Pakrasi H, Somerville CR (eds) (1988) Molecular biology of photosynthesis 3. Photosynth Res 18(1–2):5–262
Govindjee, Bohnert HJ, Bottomley W, Bryant DA, Mullet JE, Ogren WL, Pakrasi H, Somerville CR (eds) (1988) Molecular biology of photosynthesis 4. Photosynth Res 19(1–2):5–204
Govindjee, Knox RS, Amesz J (eds) (1996) Special issue dedicated to William A Arnold: photosynthetic unit: antenna and reaction centers. Photosynth Res 48(1–2):1–319
Govindjee, Sestak Z, Peters WR (2002) The early history of “Photosynthetica”, “Photosynthesis Research”, and their publishers. Photosynthetica 40(1):1–11
Govindjee, Beatty JT, Gest H (2003) Celebrating the millennium—historical highlights of photosynthesis research, part 2. Photosynth Res 76(1–3):1–11
Govindjee, Beatty JT, Gest H (eds) (2003) Celebrating the Golden jubilee of the 1952 conference of photosynthesis (Gatlinburg, Tennessee, USA). Photosynth Res 76(1–3): see a photograph, p. vii
Govindjee, Allen JF, Beatty JT (2004) Celebrating the millennium: historical highlights of photosynthesis research, part 3. Photosynth Res 80(1–3):1–13
Govindjee, Rutherford AW, Britt RD (2007) Four young research investigators were honored at the 2006 Gordon research conference on photosynthesis. Photosynth Res 92(1):137–138
Goyal A (2000) Ed Tolbert and his love for science: a journey from sheep ranch continues…. Photosynth Res 65(1):1–6
Grossman AR (2003) A molecular understanding of complementary chromatic adaptation. Photosynth Res 76(1–3):207–215
Gunner MR (ed) (2008) Computational analysis of photosynthetic systems. Photosynth Res 97(1):1–114
Gunsalus IC (1984) Learning. Annu Rev Microbiol 38:1–26
Gupta RS (2003) Evolutionary relationships among photosynthetic bacteria. Photosynth Res 76(1–3):173–183
Hangarter RP, Gest H (2004) Pictorial demonstrations of photosynthesis. Photosynth Res 80(1–3):421–425
Hangarter RP, Ort DR (1992) Norman E Good (1917–1992). Photosynth Res 34(2):245–247
Hart H (1930) Nicolas Theodore De Saussure. Plant Physiol 5(3):424–429
Hartman H (ed) (1992) Photosynthesis and the origin of life. Photosynth Res 33(2):73–176
Hatch MD (1992) I can’t believe my luck. Photosynth Res 33(1):1–14
Hatch MD (2002) C 4 photosynthesis: discovery and resolution. Photosynth Res 73(1–3):251–256
Hauska G (2004) The isolation of a functional cytochrome b 6 f complex: from lucky encounter to rewarding experiences. Photosynth Res 80(1–3):277–291
Heathcote P, Nugent J (2008) Michael Charles Whitmore Evans (September 24, 1940–February 21, 2007). Photosynth Res 96(1):1–4
Heber U (2002) Irrungn, Wirrungen? The Mehler reaction in relation to cyclic electron transport in C3 plants. Photosynth Res 73(1–3):223–231
Hedges TR Jr (2007) Charles Bonnet, his life and his syndrome. Surv Ophthalmol 52(1):111–114
Heldt H-W (2002) Three decades in transport business: studies of metabolite transport in chloroplasts—a personal perspective. Photosynth Res 73(1–3):265–272
Herron HA (1996) About Bill Arnold, my father. Photosynth Res 48(1–2):3–7
Hill R (1975) Days of visual spectroscopy. Annu Rev Plant Physiol 26:1–11
Hill DJ (1992) An overlooked symbiosis. Photosynth Res 34(3):339–340
Hoff AJ, Aartsma (2002) Jan Amesz (11 March 1934–29 January 2001). Photosynth Res 71:1–4
Homann PH (2002) Chloride and calcium in photosystem II: from effects to enigma. Photosynth Res 73(1–3):169–175
Homann PH (2003) Hydrogen metabolism of green algae: discovery and early research—a tribute to Hans Gaffron and his coworkers. Photosynth Res 76(1–3):93–103
Höxtermann E (2007) A comment on Warburg’s early understanding of biocatalysis. Photosynth Res 92(1):121–127
Hungate RE (1986) Cornelis Bernardus van Niel (1897–1985). Photosynth Res 10(1–2):139–142
Huzisige H, Ke B (1993) Dynamics of the history of photosynthesis research. Photosynth Res 35(1):185–209
Ingraham JL (1982) Roger Y. Stanier (1916–1982). Arch Mikrobiol 133(1):1
Jacquot J-P (2004) Comments on the contributions of Myroslawa Miginiac-Maslow and Peter Schürmann to the light-dependent redox regulation of choloroplastic enzymes. Photosynth Res 79(3):231–232
Jagendorf AT (1998) Chance, luck and photosynthesis research: an inside story. Photosynth Res 57(3):215–229
Jagendorf AT (2002) Photophosphorylation and the chemiosmotic perspective. Photosynth Res 73(1–3):233–241
Jeffrey SW (2007) Professor Andrew A Benson: inspirational mentor. Photosynth Res 92(2):187–192
Jensen RG (2004) Activation of rubisco controls CO 2 assimilation in light: a perspective on its discovery. Photosynth Res 82(2):187–193
Joliot P (1993) Earlier researches on the mechanism of oxygen evolution: a personal account. Photosynth Res 38(3):214–223
Joliot P (1996) René Wurmser (September 24, 1890–November 9, 1993). Photosynth Res 48(3):321–323
Joliot P (2003) Period-four oscillations of the flash-induced oxygen formation in photosynthesis. Photosynth Res 76(1–3):65–72
Joliot P, Joliot A (2003) Excitation transfer between photosynthetic units: the 1964 experiment. Photosynth Res 76(1–3):241–245
Jukes TH (1995) Mineral nutrition of plants. Photosynth Res 46(1–2):13–15
Junge W (2004) Protons, proteins and ATP. Photosynth Res 80(1–3):197–221
Kamen MD (1986) A cupful of luck, a pinch of sagacity. Annu Rev Biochem 55:1–34
Kamen MD (1989) Onward into a fabulous half-century. Photosynth Res 21(3):137–144
Kamen MD (1992) Robert (‘Robin’) Hill: an appreciation. Photosynth Res 34(3):323–325
Kaplan S (2002) Photosynthesis genes and their expression in Rhodobacter sphaeroides 2.4.1: a tribute to my students and associates. Photosynth Res 73(1–3):95–108
Karapetyan N (1993) AA Krasnovsky (1913–1993). Photosynthetica 29:481–485
Karapetyan N (1993) AA Krasnovsky (1913–1993). Photosynth Res 38(1):1–3
Katoh S (1995) The discovery and function of plastocyanin: a personal account. Photosynth Res 43(3):177–189
Katoh S (2003) Early research on the roles of plastocyanin in photosynthesis. Photosynth Res 76(1–3):255–261
Katz JJ (1990) Green thoughts in a green shade. Photosynth Res 26(3):143–160
Kauffman GB (2002) Martin D. Kamen (1913–2002), nuclear scientist and biochemist. Chem Educ 7:304–308
Ke B (2002) P430: a retrospective, 1971–2001. Photosynth Res 73(1–3):207–214
Kende H (2006) Remembering Lee McIntosh (1949–2004), a pioneer in the molecular biology of chloroplast and mitochondrion function. Photosynth Res 87(3):247–251
Klimov VV (2003) Discovery of pheophytin function in the photosynthetic energy conversion as the primary electron acceptor of photosystem II. Photosynth Res 76(1–3):247–253
Knox RS (1996) Electronic excitation transfer in the photosynthetic unit: reflections on work of William Arnold. Photosynth Res 48(1–2):35–39
Kooten O, Snel JFH (1990) The use of chlorophyll fluorescence nomenclature in plant stress physiology. Photosynth Res 25(3):147–150
Kornberg HL (2006) John Rodney Quayle (1926–2006), a brilliant scientist who was also a wise and innovative academic administrator. Photosynth Res 89(2–3):59–62
Kramer DM (ed) (2000) Emerging techniques in Photosynthesis Research. Photosynth Res 66(1–2):1–158
Krasnovsky AA (1992) Two days with Robin Hill and forty-five years with Hill reaction. Photosynth Res 34(3):327–328
Krasnovsky AA (1992) Excited chlorophyll and related problems. Photosynth Res 33:177–193
Krasnovsky AA (2003) Alexander A. Shlyk (1928–1984). Photosynth Res 76:389–403
Krasnovsky AA Jr (2003) Chlorophyll isolation, structure and function: major landmarks of the early history of research in the Russian empire and the Soviet Union. Photosynth Res 76(1–3):389–403
Krasnovsky AA, Voltovski ID, Chaika MT, Fradkin LI (1985) Alexander A. Shlyk (1928–1984). Photosynthetica 19:485–486
Krogmann DW (2000) The golden age of biochemical research in photosynthesis. Photosynth Res 63(2):109–121
Kuang T-Y, Xu C, Li L-B, Shen Y-K (2003) Photosynthesis research in the People’s Republic of China. Photosynth Res 76(1–3):451–458
Larkum AWD (2003) Contributions of Henrik Lundegårdh. Photosynth Res 76(1–3):105–110
Lavorel J (1996) The importance of being lucky: a tribute to William Arnold. Photosynth Res 48(1–2):31–34
Lewin RA (2002) Prochlorophyta—a matter of class disctinctions. Photosynth Res 73(1–3):59–61
Lichtenthaler HK, Buchanan BB, Douce R (2008) Honoring Andrew Benson in Paris. Photosynth Res 92(2):181–183
Loach P (1997) A remembrance of Melvin Calvin. Photosynth Res 54(1):1–3
Ludden PW, Roberts GP (2002) Nitrogen fixation by photosynthetic bacteria. Photosynth Res 73(1–3):115–118
Lutz M, Galmiche JM (1987) Eugene Roux (1924–2004). Photosynth Res 12:91–93
Mackenzie C, Kaplan S (eds) (2001) Genomics. Photosynth Res 70(1):1–127
Madigan MT (2003) Anoxygenic phototrophic bacteria from extreme environments. Photosynth Res 76(1–3):157–171
Malkin R (1995) Daniel I Arnon (1910–1994). Photosynth Res 43(2):77–80
Malkin S, Fork DC (1996) Bill Arnold and calorimetric measurements of the quantum requirement of photosynthesis-once again ahead of his time. Photosynth Res 48(1–2):41–46
Malkin S, Gromet-Elhanan Z (1992) Mordhay Avron (1931–1991). Photosynth Res 31(2):71–73
Marrs BL (2002) The early history of the genetics of photosynthetic bacteria: a personal account. Photosynth Res 73(1–3):55–58
Mauzerall D (1996) Bill Arnold’s concept of solid state photosynthesis and his discoveries. Photosynth Res 48(1–2):19–23
McCarty RE (2008) Zippora Gromet-Elhanan (1931–2007), a passionate and fiercely dedicated scientist. Photosynth Res 96(2):117–119
Melis A, Buchanan BB (eds) (1995) A tribute to Daniel I Arnon. Photosynth Res 46(1–2):1–377
Melis A, Happe T (2004) Trails of green alga hydrogen research—from Han Gaffron to new frontiers. Photosynth Res 80(1–3):401–409
Menke W (1990) Retrospective of a botanist. Photosynth Res 25(2):77–82
Meyer TE, Cusanovich MA (2003) Discovery and characterization of electron transfer proteins in the photosynthetic bacteria. Photosynth Res 76(1–3):111–126
Miller M, Aartsma TJ, Blankenship RE (eds) (2002) Special issue in honour of Jan Amesz: green and heliobacteria. Photosynth Res 71(1–2):vii+ 1–183
Mimuro M (2002) Visualization of excitation energy transfer processes in plants and algae. Photosynth Res 73(1–3):133–138
Mimuro M, Gantt E, Bryant DA (eds) (1997) Molecular approaches to light acclimation from Cyanobacteria to higher plants. Photosynth Res 53(2–3):81–266
Miyachi S, Iwasaki I, Shiraiwa Y (2003) Historical perspective on microalgal and cyanobacterial acclimation to low- and extremely high-CO 2 conditions. Photosynth Res 77(2–3):139–153
Mukherjee DC, Sen D (2007) A tribute to Sir Jagadish Chandra Bose (1858–1937). Photosynth Res 91(1):1–10
Murakami A, Mimuro M (2006) Yoshihiko Fujita (1932–2005): a pioneer of photoregulation in Cyanobacteria. Photosynth Res 88(1):1–5; erratum: p. 7
Myers J (1994) The 1932 experiments. Photosynth Res 40(3):303–310
Myers J (1996) Country boy to scientist. Photosynth Res 50(3):195–208
Myers J (2002) In one ear and out the other. Photosynth Res 73(1–3):21–28
Nelson N (1992) Efraim Racker (1913–1991). Photosynth Res 31(3):165–166
Nelson N, Ben-Shem A (2002) Photosystem I reaction center: past and future. Photosynth Res 73(1–3):193–206
Nickell LG (1993) A tribute to Hugo P Kortschak: the man, the scientist and the discoverer of C 4 photosynthesis. Photosynth Res 35(2):201–204
Nickelsen K (2007) Otto Warburg’s first approach to photosynthesis. Photosynth Res 92(1):109–120
Nozzolillo CG, Gorham H, Govindjee N (2007) Paul R Gorham (April 16, 1918–November 9, 2006). Photosynth Res 92(1):3–5
Ochoa S (1980) The pursuit of a hobby. Annu Rev Biochem 49:1–30
Ogawa T (2003) Physical separation of chlorophyll–protein complexes. Photosynth Res 76(1–3):227–232
Ogren WL (2003) Affixing the O to rubisco: discovering the source of photorespiratory glycolate and its regulation. Photosynth Res 76(1–3):53–63
Ohta H, Masuda T, Matsuura K (2008) Ken-ichiro Takamiya (1943–2005), a gentleman and a scientist, a superb experimentalist and a visionary. Photosynth Res 97(2):115–119
Olson JM (1994) Reminiscence about ‘Chloropseudomonas ethylicum’ and the FMO-protein. Photosynth Res 41(1):3–5
Olson JM (2004) The FMO protein. Photosynth Res 80(1–3):181–187
Olson JM, Blankenship RE (2004) Thinking about the evolution of photosynthesis. Photosynth Res 80(1–3):373–386
Olson JM, Amesz J, Ormerod JG, Blankenship RE (eds) (1994) Green and heliobacteria. Photosynth Res 41(1):1–294
Olson JM, Ivanovsky RN, Fuller RC (1996) Elena N Kondratieva (1925–1995). Photosynth Res 47(3):203–205
Ormerod J (2003) ‘Every dogma has its day’: a personal look at carbon metabolism in photosynthetic bacteria. Photosynth Res 76(1–3):135–143
Papageorgiou GC (1987) George Akoyunoglou (1927–1986). Photosynth Res 11:283–286
Papageorgiou GC (2003) Photosynthesis research in Greece: a historical snapshot (1960–2001). Photosynth Res 76(1–3):427–433
Parson WW (1989) Don DeVault. A tribute on the occasion of his retirement. Photosynth Res 22:11–13
Parson WW (1989) Don Devault: a tribute on the occasion of his retirement. Photosynth Res 22(1):11–13
Parson WW (2003) Electron donors and acceptors in the initial steps of photosynthesis in purple bacteria: a personal account. Photosynth Res 76(1–3):81–92
Pearlstein RM (1996) Bill Arnold: scientist, philosopher, friend. Photosynth Res 48(1–2):9–10
Pearlstein RM (2002) Photosynthetic exciton theory in the 1960s. Photosynth Res 73(1–3):119–126
Pierson BK (1994) Reflections on Chloroflexux. Photosynth Res 41(1):7–15
Pirson A (1994) Sixty years in algal physiology and photosynthesis. Photosynth Res 40(3):207–221
Porra RJ (2002) The chequered history of the development and use of simultaneous equations for the accurate determination of chlorophylls a and b . Photosynth Res 73(1–3):149–156
Portis AR Jr, Parry MAJ (2007) Discoveries in Rubisco (Ribulose 1,5-bisphosphate carboxylase/oxygenase): a historical perspective. Photosynth Res 94(1):121–143
Portis AR Jr, Salvucci ME (2002) The discovery of rubisco activase—yet another story of serendipity. Photosynth Res 73(1–3):257–264
Prasil O, Suggett DJ, Cullen JJ, Babin M, Govindjee (2008) Aquafluo 2007: chlorophyll fluorescence in aquatic sciences, an international conference held in Nové Hrady. Photosynth Res 95(1):111–115
Prince RC (1992) Robert Hill, FRS; his published work. Photosynth Res 34(3):329–332
Putnam-Evans C, Barry B (eds) (2007) Photosynthetic water oxidation. Photosynth Res 92(3):273–425
Rabinowitch E (1961) Robert Emerson (1903–1959). Biogr Mem Natl Acad Sci USA 25:112–131
Rabinowitch A (2005) Founder and father. Bull At Sci 61(1):30–37
Raghavendra AS, Sane PV, Mohanty P (2003) Photosynthesis research in India: transition from yield physiology into molecular biology. Photosynth Res 76(1–3):435–450
Rao KK (1999) David Hall (1935–1999). Photosynth Res 62(2):117–119
Rebeiz CA, Benning C, Bohnert H, Hoober JK, Portis AR (2007) Govindjee was honored with the first lifetime achievement award, and Britta Förster and coworkers, with the first annual paper prize of Rebeiz foundation for basic research. Photosynth Res 94(1):147–151
Renger G (1987) Conference report on the Japan/US-binational seminar on “energy conversion: photochemical reaction centers and oxygen evolving complexes of plant photosynthesis”. Photosynth Res 13(3):261–268
Renger G (2003) Apparatus and mechanism of photosynthetic oxygen evolution: a personal perspective. Photosynth Res 76(1–3):269–288
Renger G (2008) Horst Tobias Witt (March 1, 1922–May 14, 2007). Photosynth Res 96(1):5–8
Rich PR (1992) Robin Hill: a personal perspective. Photosynth Res 34(3):333–335
Rich PR (ed) (1992) Robin Hill. Photosynth Res 34(3):319–488
Rieppel O (1985) The dream of Charles Bonnet (1720–1793). Gesnerus 42(3–4):359–367
Rippka R (2003) Germaine Stanier (Cohen-Bazire) 1920–2001. Arch Hydrobiol-Suppl 148:17–34
Rochaix J-D (2002) The three genomes of Chlamydomonas. Photosynth Res 73(1–3):285–293
Rodermel S, Viret JF, Krebbers E (2005) Lawrence Bogorad (1921–2003), a pioneer in photosynthesis research: a tribute. Photosynth Res 83(1):17–24
Rosenberg JL (2004) The contributions of James Franck to photosynthesis research: a tribute. Photosynth Res 80(1–3):71–76
Rotblatt J (2000) Fifty Pugwash conferences: a tribute to Eugene Rabinowitch. Available online at: http://www.pugwash.org/reports/pac/pac256/rotblat.htm )
Rurainski HJ (2004) The conference at Airlie House in 1963. Photosynth Res 80(1–3):439–446
Sahrawy Barragán M (2005) A tribute to Julio López-Gorgé (1935–2004): the music in science. Photosynth Res 83(3):283–286
San Pietro A (2008) Memories: from protein synthesis to photosynthesis. Photosynth Res 96(3):185–199
Sane PV, Phondke GP (2006) Vidyadhar Govind (Pandit) Tatake (1926–2004): an ingenious instrumentalist, an authority on thermoluminescence, and a lover of classical Indian music. Photosynth Res 89(1):49–51
Satoh K (2003) The identification of the photosystem II reaction center: a personal story. Photosynth Res 76(1–3):233–240
Sayre RT, Hippler M (eds) (2004) Molecular genomics of the Chlamydomonas chloroplast. Photosynth Res 82(3):201–354
Schröder WP, Kieselbach T (2003) Update on chloroplast proteomics. Photosynth Res 78(3):181–193
Seibert M (1991) Don Charles DeVault. Photosynth Res 28(3):95–98
Seibert M, Thurnauer M (1999) Therese Marie Cotton-Uphaus (1939–1998). Photosynth Res 61(3):193–196
Seibert M, Wasielewski MR (2003) The isolated photosystem II reaction center: first attempts to directly measure the kinetics of primary charge separation. Photosynth Res 76(1–3):263–268
Senger H (2004) Tribute: in memory of professor Dr Dr hc André Pirson, a pioneer in photosynthesis and a dedicated academic teacher. Photosynth Res 82(2):111–114
Sestak Z (1986) Hiroshi Tamiya (1903–1986). Photosynthetica 20:81
Sestak Z (1992) Mordhay Avron (1931–1991). Photosynthetica 26:163–164
Shen Y (1994) Dynamic approaches to the mechanism of photosynthesis. Photosynth Res 39(1):1–13
Shestakov SV (2002) Gene-targeted and site-directed mutagenesis of photosynthesis genes in Cyanobacteria. Photosynth Res 73(1–3):279–284
Shin M (2004) How is ferredoxin-NADP reductase involved in the NADP photoreduction of chloroplasts? Photosynth Res 80(1–3):307–313
Siedow JN (2002) A biographical sketch of Charles F Yocum: “it’s the biochemistry, stupid”. Photosynth Res 72(2):123–130
Smocovitis VB (2006) One hundred years of American botany: a short history of Botanical Society of America. Am J Bot 93:942–952
Staehelin LA (2003) Chloroplast structure: from chlorophyll granutes to supra-molecular architecture of thylakoid membranes. Photosynth Res 76(1–3):185–196
Stanier RY (1980) The journey, not the arrival matters. Annu Rev Microbiol 34:1–48
Stemler AJ (2002) The bicarbonate effect, oxygen evolution, and the shadow of Otto Warburg. Photosynth Res 73(1–3):177–183
Steward FC, Memorial Committee (1947) In memoriam: Frederick Frost Blackman (July 25, 1866–January 30, 1947). Plant Physiol 22(3):ii–viii
Strehler BL (1996) Halcyon days with Bill Arnold. Photosynth Res 48(1–2):11–18
Strotmann H, Soeder C-J (2005) August Ried (1924–2004), an outstanding researcher, and artist and a dear friend. Photosynth Res 83(3):279–281
Sugiura M (2003) History of chloroplast genomics. Photosynth Res 76(1–3):371–377
Sundqvist C, Björn LO (2007) A tribute to Hemming Virgin (1918–2005), a Swedish pioneer in plant photobiology. Photosynth Res 92(1):13–16
Sweeney BM (1987) Living in the golden age of biology. Annu Rev Plant Physiol 38:1–9
Tabita FR (2004) Research on carbon dioxide fixation in photosynthetic microorganisms (1971–present). Photosynth Res 80(1–3):315–332
Tamiya H (1966) Synchronous cultures of algae. Annu Rev Plant Physiol 17:1–21
Tandeau de Marsac N (2003) Phycobiliproteins and phycobilisomes: the early observations. Photosynth Res 76(1–3):197–205
Thake B (2006) Gordon Elliott (Tony) Fogg (1919–2005): pioneering plant physiologist and gifted writer. Photosynth Res 90(1):1–4
Thornber JP (1995) Thirty years of fun with antenna pigment-proteins and photochemical reaction centers: a tribute to the people who have influenced my career. Photosynth Res 44(1–2):3–22
Tobin E (2006) Samuel Goodnow Wildman (1912–2004): discoverer of fraction I protein, later named Rubisco, who worked till he was 92. Photosynth Res 88(2):105–108
Tolbert NE (1997) The C2 oxidative photosynthetic cycle. Annu Rev Plant Physiol Plant Mol Biol 48:1–25
Trebst A (2007) Inhibitors in the functional dissection of the photosynthetic electron transport system. Photosynth Res 92(2):217–224
Trebst A, Depka B (1995) Polyphenol oxidase and photosynthesis research. Photosynth Res 46(1–2):41–44
van Ginkel G, Goedheer J (1991) Jan Bartholomeus Thomas (1907–1991). Photosynth Res 30(2–3):65–69
Van Niel CB (1967) The education of a microbiologist: some reflections. Annu Rev Microbiol 21:1–30
Van Rensen JJS (2002) Role of bicarbonate at the acceptor side of photosystem II. Photosynth Res 73(1–3):185–192
Vass I (2003) The history of photosynthetic thermoluminescence. Photosynth Res 76(1–3):303–318
Vennesland B (1981) Recollections and small confessions. Annu Rev Plant Physiol 32:1–48
Verméglio A (2002) The two-electron gate in photosynthetic bacteria. Photosynth Res 73(1–3):83–86
Vernon LP (2003) Photosynthesis and the Charles F Kettering research laboratory. Photosynth Res 76(1–3):379–388
Virgin H, Volotovskii (1993) Tikhon N Godnev (1892–1982). Photosynthetica 29:163–165
Vredenberg WJ (1981) Professor Dr. E.C. Wassink (1904–1981). Photosynthetica 15:315–316
Wada H, Murata N (2007) The essential role of phosphatidylglycerol in photosynthesis. Photosynth Res 92(2):205–215
Walker DA (1992) Robert Hill. Photosynth Res 34(3):337–338
Walker DA (1995) One thing leading to another. Photosynth Res 46(1–2):45–46
Walker DA (1997) Tell me where all the past years are. Photosynth Res 51:1–26
Walker DA (2002) ‘And whose bring presence’—an appreciation of Robert Hill and his reaction. Photosynth Res 73(1–3):51–54
Walker DA (2003) Chloroplasts in envelopes: CO 2 fixation by fully functional intact chloroplasts. Photosynth Res 76(1–3):319–327
Walker DA (2007) From Chlorella to chloroplasts: a personal note. Photosynth Res 92(2):181–185
Warburg O (1964) Prefatory chapter. Annu Rev Biochem 33:1–14
Weber G (1990) Whither biophysics. Annu Rev Biophys 19:1–6
Whatley FR (1995) Photosynthesis by isolated chloroplasts: the early work in Berkeley. Photosynth Res 46(1–2):17–26
Wildman SG (2002) Along the trail from fraction I protein to rubisco (ribulose bisphosphate carboxylase-oxygenase). Photosynth Res 73(1–3):243–250
Wildman SG, Hirsch AM, Kirchanski SJ, Spencer D (2004) Chloroplasts in living cells and the string-of-grana concept of chloroplast structure revisited. Photosynth Res 80(1–3):345–352
Williams RJP (2005) The discovery of the nature of ferredoxin in photosystems: a recollection. Photosynth Res 85(2):247–250
Witt HT (1991) Functional mechanism of water splitting photosynthesis. Photosynth Res 29(2):55–77
Witt HT (2004) Steps on the way to building blacks, topologies, crystals and x-ray structural analysis of photosystems I and II of water-oxidizing photosynthesis. Photosynth Res 80(1–3):85–107
Woese CR (2004) The archaeal concept and the world it lives in: a retrospective. Photosynth Res 80(1–3):361–372
Wydrzynski TJ (2004) Early indications for manganese oxidation state changes during photosynthetic oxygen production: a personal account. Photosynth Res 80(1–3):125–135
Xiong L, Sayre RT (2004) Engineering the chloroplast encoded proteins of Chlamydomonas. Photosynth Res 80(1–3):411–419
Yocum C, Ferguson-Miller S, Blankenship R (2001) Gerald T Babcock (1946–2000). Photosynth Res 68(2):89–94
Zeinalov Y (2006) A brief history of the investigations on photosynthesis in Bulgaria. Photosynth Res 88(2):195–204
Zelitch I (2001) Travels in a world of small science. Photosynth Res 67(3):157–176
Download references
Acknowledgments
I thank Vanessa Conrad for typing this text, and I am grateful to Feng Sheng Hu, Head of Plant Biology, University of Illinois, for his support.
Author information
Authors and affiliations.
Department of Plant Biology, University of Illinois at Urbana-Champaign, 265 Morrill Hall, 505 South Goodwin Avenue, Urbana, IL, 61801, USA
You can also search for this author in PubMed Google Scholar
Corresponding author
Correspondence to Govindjee .
Additional information
I will appreciate readers to send me (by e-mail: [email protected]) corrections, if any, and additional references from other journals.
Rights and permissions
Reprints and permissions
About this article
Govindjee List of biography and history published mostly in Photosynthesis Research , 1988–2008. Photosynth Res 99 , 139–153 (2009). https://doi.org/10.1007/s11120-008-9392-0
Download citation
Published : 31 January 2009
Issue Date : February 2009
DOI : https://doi.org/10.1007/s11120-008-9392-0
Share this article
Anyone you share the following link with will be able to read this content:
Sorry, a shareable link is not currently available for this article.
Provided by the Springer Nature SharedIt content-sharing initiative
- Find a journal
- Publish with us
- Track your research

The Historical Perspective of Photosynthesis
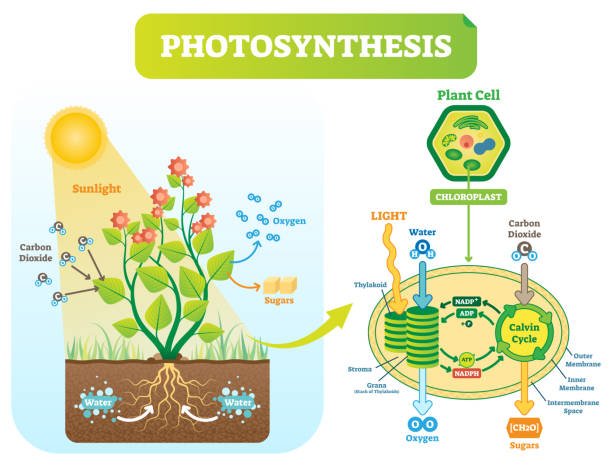
The Historical Perspective of Photosynthesis:
Photosynthesis is the process by which green plants, algae, and some bacteria convert light energy, usually from the sun, into chemical energy in the form of glucose . It is a crucial biological process, not just because it provides food for the organism conducting it, but because it plays an instrumental role in the carbon cycle and in the production of the oxygen we breathe. The understanding of this process, however, did not occur overnight. It was the culmination of centuries of observation, experimentation, and debate among scientists from diverse fields of study.
Highlights:
- Early Conceptions (Ancient Greeks to the 17th Century) : Theophrastus, an Ancient Greek scholar, first suggested that plants fed from their roots. In the 17th century, Jan Baptista van Helmont discovered that plants grew in weight but the soil’s weight barely changed, indicating that plants gained mass from somewhere else, though he attributed it solely to water.
- Understanding Gases (18th Century) : Joseph Priestley found that plants could ‘restore’ air, marking the discovery of oxygen. Jan Ingenhousz then showed that this process only occurred in sunlight, demonstrating the first explicit understanding of photosynthesis.
- Formation of Photosynthesis Equation (19th Century) : Jean Senebier discovered that plants absorb carbon dioxide and release oxygen. Later, Julius von Sachs provided evidence that glucose produced during photosynthesis was stored as starch in plants, forming the basis of the photosynthesis equation we know today.
- Discovering Chlorophyll and Electron Transport Chain (20th Century) : Richard Willstätter discovered the crucial role of chlorophyll in absorbing light energy. Later in the century, scientists like Daniel Arnon further elaborated the concept of the electron transport chain in photosynthesis.
- Exploring Photosystems and Genetic Manipulation (21st Century) : Modern science is investigating photosystems and the efficiency of photosynthesis. Genetic manipulation of plants to increase photosynthetic efficiency has significant implications for agricultural productivity and sustainability.
- Future Prospects : Scientists are currently exploring artificial photosynthesis and the potential to use this process to create energy-rich fuels. Genetic engineering and synthetic biology are being leveraged to improve photosynthesis, and the study of marine organisms is shedding light on unique and potentially more efficient methods of photosynthesis. These developments highlight the potential of photosynthesis to address pressing global issues like climate change, food security, and energy sustainability.
Table of Contents
Early Conceptions: From Ancient Greeks to the 17th Century
The Ancient Greeks were among the first civilizations to propose theories related to photosynthesis. Theophrastus (371–287 BC) , a pupil of Aristotle, suggested that plants feed from their roots. However, it took many centuries for humanity to progress beyond these early assumptions.
In the 17th century, Belgian physician Jan Baptista van Helmont conducted a five-year experiment growing a willow tree. He observed that the tree gained considerable weight while the soil’s weight barely changed. Van Helmont concluded that the increase in the tree’s mass came from the water he added, but he did not realize the role of the sunlight or air in plant growth.
Understanding Gases and the Emergence of the Photosynthesis Concept
The 18th century brought significant progress in understanding photosynthesis, thanks to the pioneering work of British scientist Joseph Priestley . Priestley discovered that plants could ‘restore’ air that had been ‘injured’ by burning or breathing, which he demonstrated using a bell jar, a candle, and a sprig of mint. This marked the discovery of oxygen, although he did not name it as such.
Building on Priestley’s work, Dutch physician Jan Ingenhousz demonstrated in the late 18th century that this ‘restorative’ process only occurred when the plant was exposed to sunlight. This was the first explicit demonstration of photosynthesis as we understand it today.
The Formation of the Modern Photosynthesis Equation
In the 19th century, several scientists made strides in understanding the details of photosynthesis. Jean Senebier , a Swiss pastor and naturalist, discovered that plants absorb carbon dioxide and release oxygen. This discovery helped to form the basis of our modern understanding of the photosynthesis equation.
A little later, the German botanist Julius von Sachs provided evidence that the glucose produced during photosynthesis is stored as starch in the plant. This research solidified the understanding of photosynthesis as a process converting light energy into chemical energy.
Discovering Chlorophyll and the Electron Transport Chain
The 20th century witnessed tremendous strides in the comprehension of photosynthesis at the molecular level. In the early 1900s, Richard Willstätter, a German chemist , found that chlorophyll, a pigment in plants, was integral to the absorption of light energy.
Later in the 20th century, scientists like Daniel Arnon contributed to the understanding of the electron transport chain, a crucial component of the ‘light reactions’ of photosynthesis. This understanding helped to elucidate how light energy is transformed into chemical energy.
Into the 21st Century: Photosystems and Genetic Manipulation
As the 21st century dawned, the focus shifted to understanding the photosystems and the overall efficiency of the photosynthesis process. Researchers began exploring the possibilities of genetically manipulating plants to increase the efficiency of photosynthesis, which could have vast implications for agricultural productivity and sustainability.
From early speculation in ancient Greece to the modern detailed understanding of the molecular processes involved, the history of understanding photosynthesis is a prime example of the collective human endeavor for knowledge. As we continue to face global challenges such as climate change and food security, the study of photosynthesis and ways to enhance its efficiency remains ever crucial.
Today, the world is also turning its attention to the potential of artificial photosynthesis, hoping to harness solar energy efficiently just as plants do. This technology aims to convert sunlight, water, and carbon dioxide directly into energy-rich fuels, while at the same time reducing greenhouse gas emissions and reliance on fossil fuels.
In 2020, a team from the University of Cambridge created a device — a type of artificial leaf — that utilizes sunlight, carbon dioxide, and water to produce a fuel known as syngas. While the technology is in its early stages and there are many hurdles to overcome, it shows the revolutionary potential of using our understanding of photosynthesis to tackle energy and environmental challenges.
In another notable development, researchers are also studying marine organisms, like algae and cyanobacteria, that perform photosynthesis. These organisms are particularly interesting because they have unique methods for carbon fixation, potentially more efficient than terrestrial plants.
Moreover, genetic engineering and synthetic biology are providing unprecedented possibilities for improving the photosynthetic process. Scientists are experimenting with introducing genes from more efficient photosynthesizing organisms into crops, with the aim to increase agricultural yields. Others are looking into altering chloroplasts, the organelles where photosynthesis occurs, to boost the process.
The exploration of photosynthesis and its potential enhancements is a testament to the never-ending scientific quest for knowledge and application. From the nascent understanding of Ancient Greeks to the profound insights of contemporary scientists, photosynthesis remains a focal point of biological and environmental research.
With the pressing need to address climate change, promote sustainable agriculture, and seek alternative energy sources, the study of photosynthesis and its manipulation for greater efficiency is likely to remain at the forefront of scientific endeavors in the coming decades.
Indeed, the history of photosynthesis is still being written. As scientists continue to unravel its intricacies and make technological advancements, we will better understand how this process underpins life on Earth and how we can harness its power to secure our future on this planet
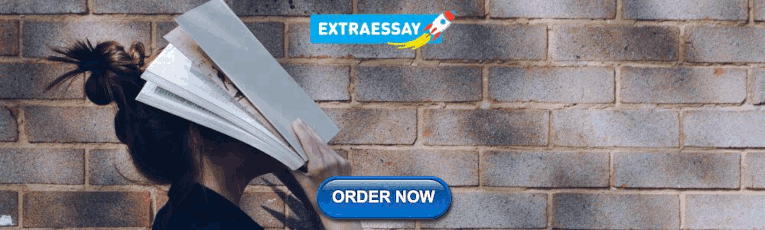
IMAGES
VIDEO
COMMENTS
With limited agricultural land and increasing human population, it is essential to enhance overall photosynthesis and thus productivity. Oxygenic photosynthesis begins with light absorption, followed by excitation energy transfer to the reaction centres, primary photochemistry, electron and proton transport, NADPH and ATP synthesis, and then CO ...
6CO2 + 12H2O + light energy → C6H12O6 + 6O2 + 6H2O. Note that the above global equation of photosynthesis emphasizes that the oxygen molecules released into the atmosphere originate from water oxidation, not from carbon dioxide, as established using 18 O-labelled water ( Ruben et al., 1941 ).
Thus, Olson's and other less impactful perspectives on the origin of photosynthesis before the 90s, inevitably see the emergence of the trait as a natural progression from a primordial soup origin. Nevertheless, in the 80s, perspectives on the evolution of photosynthesis derived from Oparin's heterotrophic hypothesis had been firmly
4(1) in 1983. (For a history of the journal Photo-synthesis Research, see Govindjee et al. (2002).1) The Historical corner of Photosynthesis Research began publishing dedications, historical papers, personal perspectives, tributes and obituaries only in 1986. In 2006, we celebrate its 20th anniversary. The very first dedication volume was to ...
The history of the studies done on photosynthesis dates back into the 17th century with Jan Baptist van Helmont. He rejected the ancient idea that plants take most of their biomass from the soil. For the proof, he performed willow tree experiment. He started with a willow tree of 2.27 kg. Over 5 years, it grew to 67.7 kg.
A wealth of evidence indicates that photosynthesis is an ancient process that originated not long after the origin of life and has evolved via a complex path to produce the distribution of types of photosynthetic organisms and metabolisms that are found today ( Blankenship, 2002; Björn and Govindjee, 2009 ). Figure 1 shows an evolutionary tree ...
Govindjee and Gest H (eds) (2002) Celebrating the millennium - historical highlights of photosynthesis research, Part 1. Photosynth Res 73: 1-308. Article Google Scholar Govindjee and Krogmann D (2004) Discoveries in oxygenic photosynthesis (1727-2003): a perspective. Photosynth Res 80: 15-57
Oxygen-producing, or oxygenic, photosynthesis "was the last of the great inventions of microbial metabolism, and it changed the planetary environment forever," says geobiologist Paul Falkowski of Rutgers University in New Brunswick, New Jersey. Given its importance in making and keeping Earth lush, photosynthesis ranks high on the top-10 ...
The personal perspectives are delightful, it is truly a "must own" book for its focus on the personal historical context surrounding most of the important breakthroughs in photosynthesis research." (Douglas Bruce, Professor of Biological Sciences, Brock University, St. Catharines, ON, Canada)
In here, some of the historical context of the study of the evolution of photosynthesis is examined, which led to the consolidation of the current notion that the origin of anoxygenic photosynthesis occurred before theorigin of oxygenic photosynthesis. What if oxygenic photosynthesis is a primordial process with roots at the origin of life? What would the impact of this change in perspective ...
Instead of plants, Schulten chose to study purple bacteria, which embody the simplest form of photosynthesis. Bacteria emerged around 3.5 billion years ago on the earth; photosynthesis, an ancient process, arose sometime thereafter, although no firm evidence exists to prove its exact origins.
Historical Perspectives. The Beginnings of Research on Photosynthesis. Chapter. pp 7-17. Cite this chapter. Download book PDF. J. Kenneth Hoober. Part of the book series: Cellular Organelles ( (CORG)) 151 Accesses.
Tree adapted from Pace (1997). Overwhelming evidence indicates that eukaryotic photosynthesis originated from endosymbiosis of cyanobacterial-like organisms, which ultimately became chloroplasts ( Margulis, 1992 ). So the evolutionary origin of photosynthesis is to be found in the bacterial domain.
In chemical terms, photosynthesis is a light-energized oxidation-reduction process. (Oxidation refers to the removal of electrons from a molecule; reduction refers to the gain of electrons by a molecule.) In plant photosynthesis, the energy of light is used to drive the oxidation of water (H 2 O), producing oxygen gas (O 2 ), hydrogen ions (H ...
Cyanobacteria and plants carry out oxygenic photosynthesis. They use water to generate the atmospheric oxygen we breathe and carbon dioxide to produce the biomass serving as food, feed, fibre and fuel. This paper scans the emergence of structural and mechanistic understanding of oxygen evolution over the past 50 years.
This editorial has four goals: (1) to inform the readers of 'Photosynthesis Research' about the past of the 'Historical corner'; which began 20 years ago; (2) to encourage photosynthesis ...
The history of changes in the meaning of photosynthesis is examined, which began with the discovery of anoxygenic photosynthetic bacteria and photophosphorylation and necessitated redefinition of the term. In 1893, Charles Barnes (1858-1910) proposed that the biological process for 'synthesis of complex carbon compounds out of carbonic acid, in the presence of chlorophyll, under the ...
The emergence of structural and mechanistic understanding of oxygen evolution over the past 50 years is scanned and speculative concepts and the stepped insight provided by novel experimental and theoretical techniques are reviewed. Abstract Cyanobacteria and plants carry out oxygenic photosynthesis. They use water to generate the atmospheric oxygen we breathe and carbon dioxide to produce the ...
This Editorial has four goals: (1) to inform the readers of 'Photosynthesis Research' about the past of the 'Historical corner'; which began 20 years ago; (2) to encourage photosynthesis researchers and historians of science to contact me for publishing papers of historical interest; these include: (a) Obituaries and Tributes; (b) historical papers on current and past discoveries and ...
Title: The Historical Perspective of Photosynthesis: A Journey Through Time. Photosynthesis, the process by which green plants, algae, and some bacteria convert light energy, primarily from the Sun, into chemical energy in the form of glucose, is the fundamental basis of life on Earth. The understanding and conceptualization of this complex ...
This editorial has four goals: (1) to inform the readers of 'Photosynthesis Research' about the past of the 'Historical corner'; which began 20 years ago; (2) to encourage photosynthesis researchers and historians of science to contact me for publishing papers of historical interest; these include: (a) Obituaries and Tributes; (b) historical papers on current and past discoveries and ...
As an outgoing Editor of the Historical Corner of Photosynthesis Research, I present here the following list of papers of historical interest for the benefit of all. The first paper I published was: Govindjee (1988) The Discovery of Chlorophyll-protein Complex by Emil L. Smith during 1937-1941. Photosynth Res 16:285-289. In order to bring to the readers this List of references on the ...
The Historical Perspective of Photosynthesis: Photosynthesis is the process by which green plants, algae, and some bacteria convert light energy, usually from the sun, into chemical energy in the form of glucose.It is a crucial biological process, not just because it provides food for the organism conducting it, but because it plays an instrumental role in the carbon cycle and in the ...