
- Login or signup to the library
- my Channels
- All Channels
- List events
- List channels
- List collections
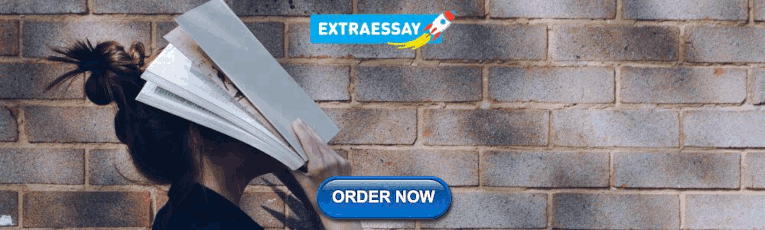
You are here
Tsunami disasters: case studies and reports.

Resources on this Collection
10 Lessons Learned from the South Asia Tsunami of 26th December 2004

Approaches to Equity in Post-Tsunami Assistance - Sri Lanka: A Case Study

Environment and Reconstruction in Aceh: two years after the tsunami

Evolving Strategies For Long-term Rehabilitation On Shelter and Development in the Tsunami Affected Areas of Tamil Nadu

Impact of the tsunami response on local and national capacities: Maldives country report

Indian Ocean Earthquake and Tsunami UNICEF response at six months update
You must be logged in to post a comment
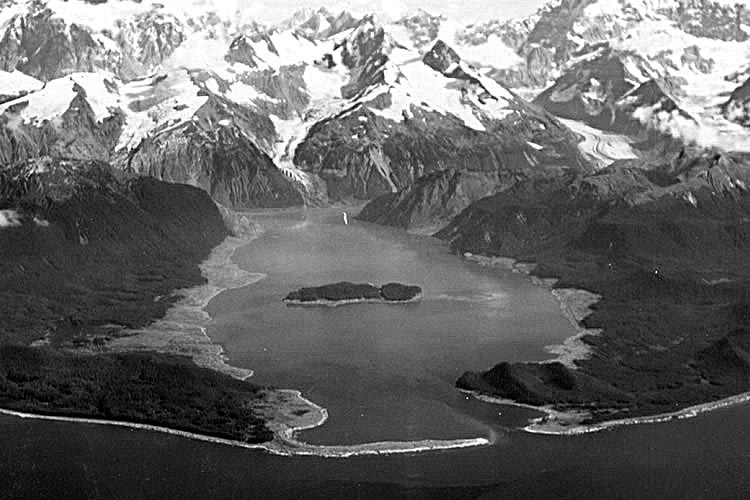
The Science of Tsunamis
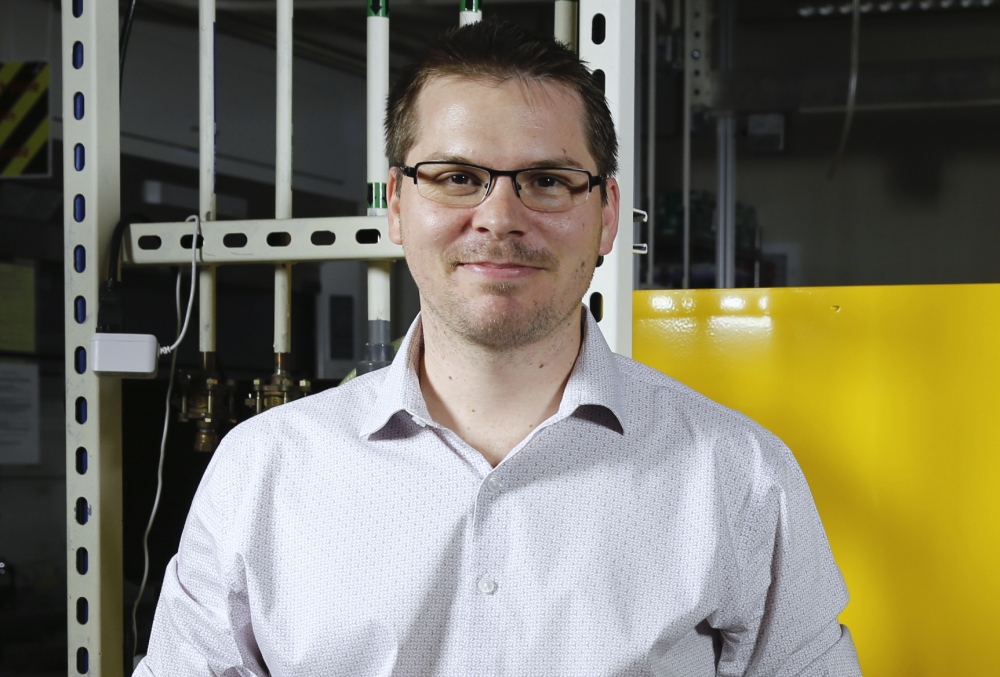
The word “tsunami” brings immediately to mind the havoc that can be wrought by these uniquely powerful waves. The tsunamis we hear about most often are caused by undersea earthquakes, and the waves they generate can travel at speeds of up to 250 miles per hour and reach tens of meters high when they make landfall and break. They can cause massive flooding and rapid widespread devastation in coastal areas, as happened in Southeast Asia in 2004 and in Japan in 2011.
But significant tsunamis can be caused by other events as well. The partial collapse of the volcano Anak Krakatau in Indonesia in 2018 caused a tsunami that killed more than 400 people. Large landslides, which send immense amounts of debris into the sea, also can cause tsunamis. Scientists naturally would like to know how and to what extent they might be able to predict the features of tsunamis under various circumstances.
Most models of tsunamis generated by landslides are based on the idea that the size and power of a tsunami is determined by the thickness, or depth, of the landslide and the speed of the “front” as it meets the water. In a paper titled “Nonlinear regimes of tsunami waves generated by a granular collapse,” published online in the Journal of Fluid Mechanics, UC Santa Barbara mechanical engineer Alban Sauret and his colleagues, Wladimir Sarlin, Cyprien Morize and Philippe Gondret at the Fluids, Automation and Thermal Systems (FAST) Laboratory at the University of Paris-Saclay and the French National Centre for Scientific Research (CNRS), shed more light on the subject. (The article also will appear in the journal’s July 25 print edition.)
This is the latest in a series of papers the team has published on environmental flows, and on tsunami waves generated by landslides in particular. Earlier this year, they showed that the velocity of a collapse — i.e., the rate at which the landslide is traveling when it enters the water — controls the amplitude, or vertical size, of the wave.
In their most recent experiments, the researchers carefully measured the volume of the granular material, which they then released, causing it to collapse as a cliff would, into a long, narrow channel filled with water. They found that while the density and diameter of the grains within a landslide had little effect on the amplitude of the wave, the total volume of the grains and the depth of the liquid played much more crucial roles.
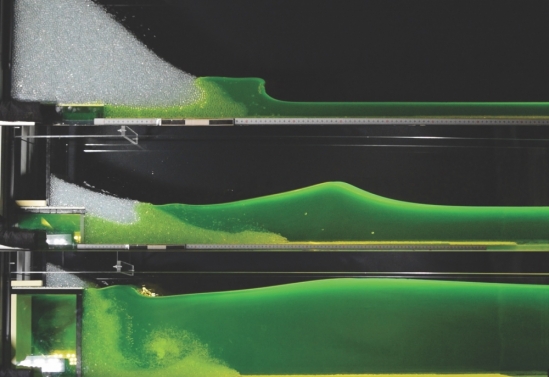
A photograph of an experimental lab setup reflecting the fluid dynamics of a tsunami
Photo Credit: ILLUSTRATION COURTESY OF FAST AND UC SANTA BARBARA
“As the grains enter the water, they act as a piston, the horizontal force of which governs the formation of the wave, including its amplitude relative to the depth of the water,” said Sauret. (A remaining challenge is to understand what governs the speed of the piston.) “The experiments also showed that if we know the geometry of the initial column [the material that flows into the water] before it collapses and the depth of the water where it lands, we can predict the amplitude of the wave.”
The team can now add this element to the evolving model they have developed to couple the dynamics of the landslide and the generation of the tsunami. A particular challenge is to describe the transition from an initial dry landslide, when the particles are separated by air, to an underwater granular flow, when the water has an important impact on particle motion. As that occurs, the forces acting on the grains change drastically, affecting the velocity at which the front of grains that make up the landslide enters the water.
Currently, there is a large gap in the predictions of tsunamis based on simplified models that consider the field complexity (i.e., the geophysics) but do not capture the physics of the landslide as it enters the water. The researchers are now comparing the data from their model with data collected from real-life case studies to see if they correlate well and if any field elements might influence the results.
Share this article
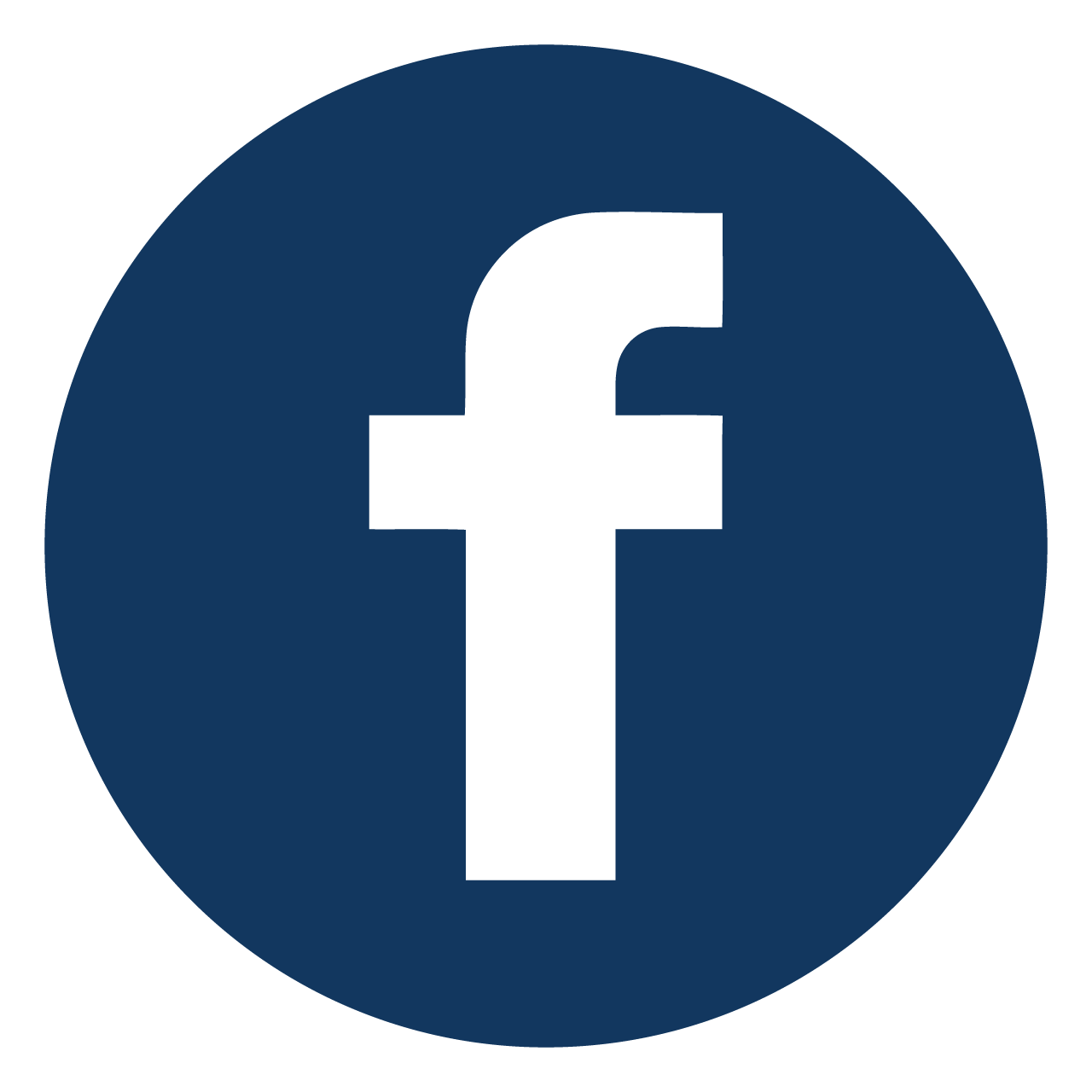
About UC Santa Barbara
The University of California, Santa Barbara is a leading research institution that also provides a comprehensive liberal arts learning experience. Our academic community of faculty, students, and staff is characterized by a culture of interdisciplinary collaboration that is responsive to the needs of our multicultural and global society. All of this takes place within a living and learning environment like no other, as we draw inspiration from the beauty and resources of our extraordinary location at the edge of the Pacific Ocean.
Related Stories
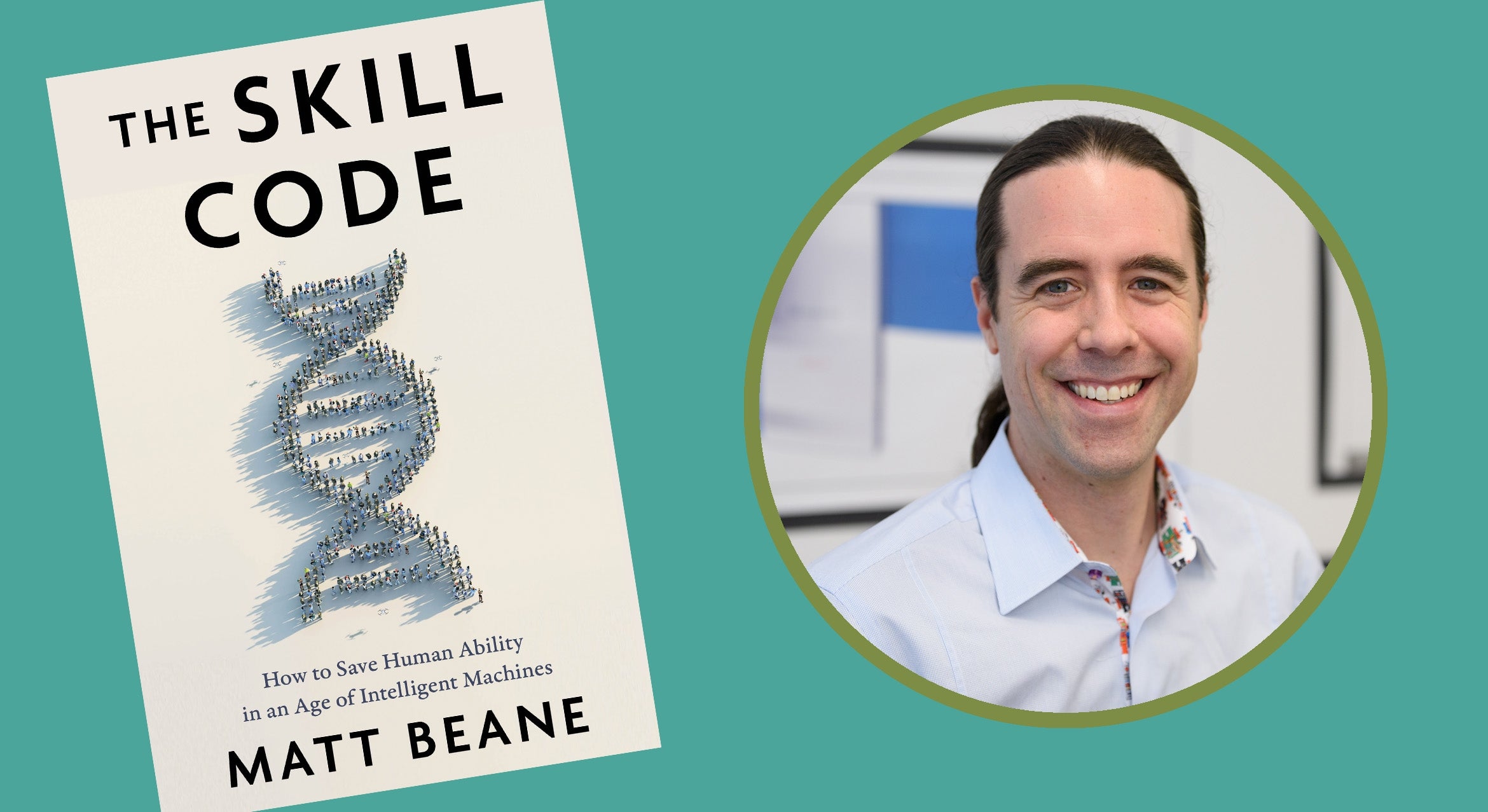
April 30, 2024
How to build and protect skills in our modern workplace, a world filled with AI and robots
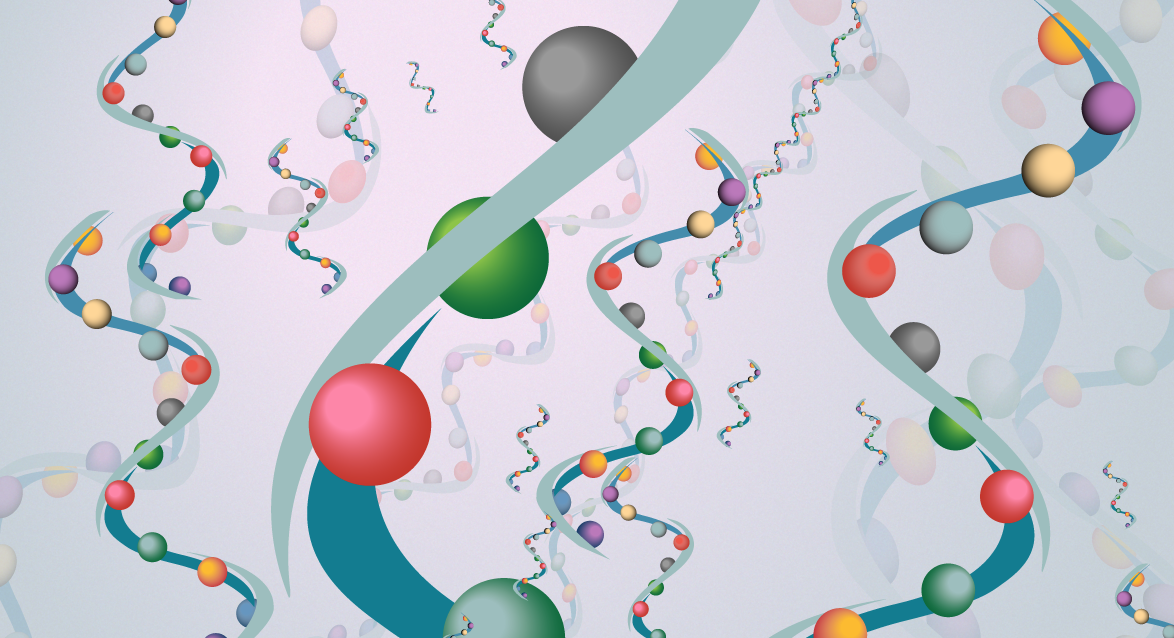
Researchers reveal a new approach for treating degenerative diseases
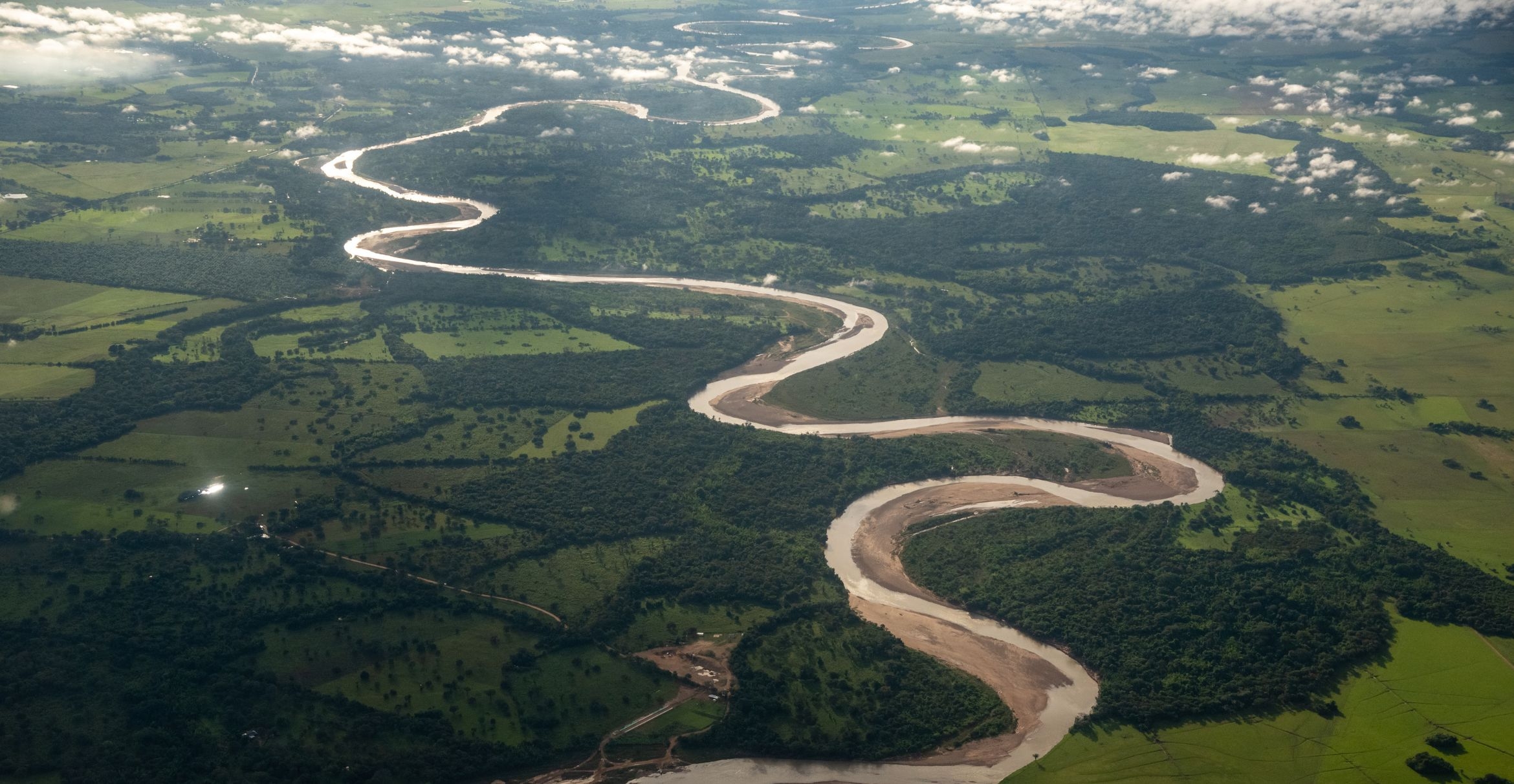
April 25, 2024
Managing meandering waterways in a changing world
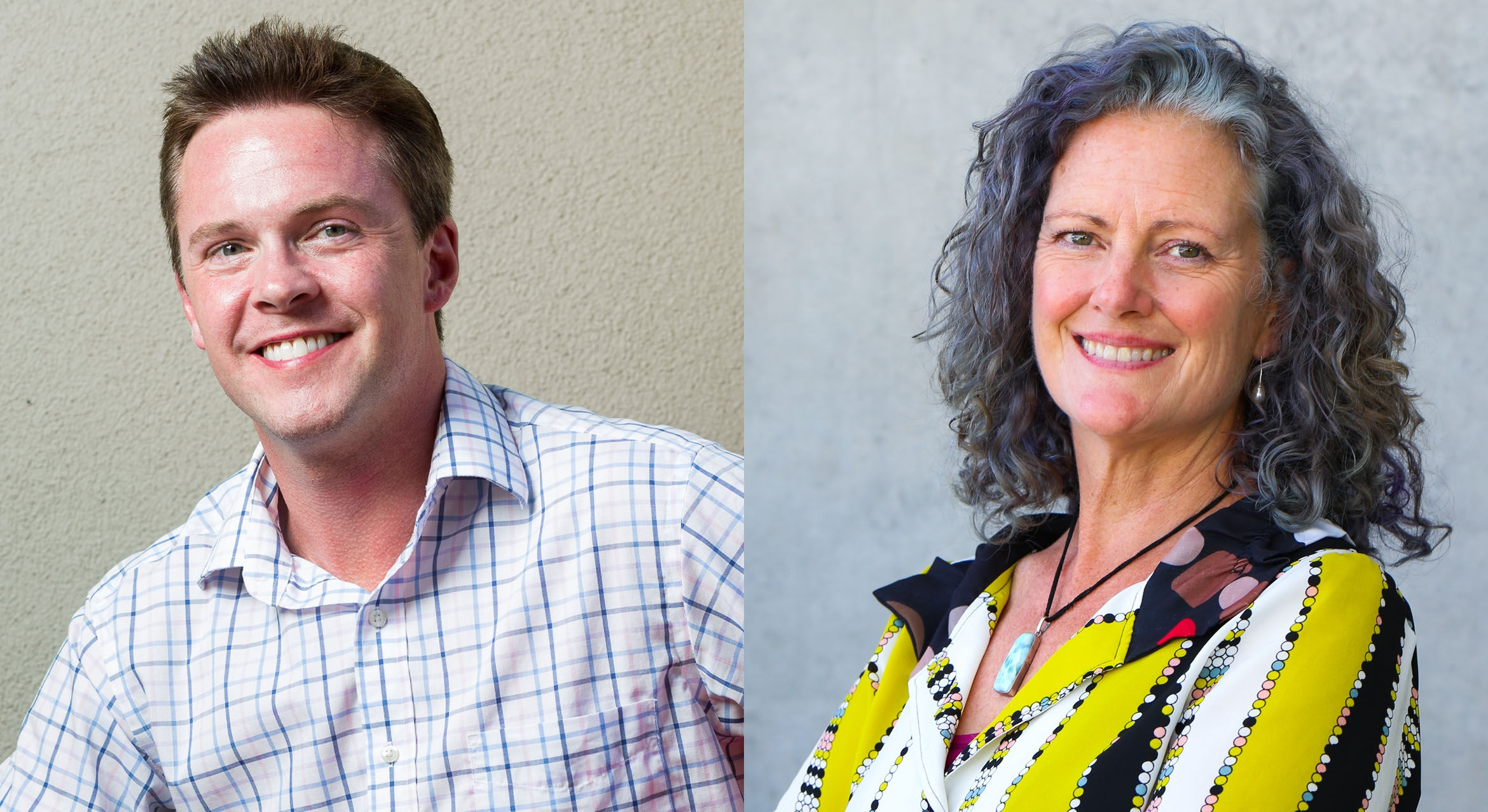
April 23, 2024
A prestigious career honor for two elite engineers

The Science of tsunamis
The word "tsunami" brings immediately to mind the havoc that can be wrought by these uniquely powerful waves. The tsunamis we hear about most often are caused by undersea earthquakes, and the waves they generate can travel at speeds of up to 250 miles per hour and reach tens of meters high when they make landfall and break. They can cause massive flooding and rapid widespread devastation in coastal areas, as happened in Southeast Asia in 2004 and in Japan in 2011.
But significant tsunamis can be caused by other events as well. The partial collapse of the volcano Anak Krakatau in Indonesia in 2018 caused a tsunami that killed more than 400 people. Large landslides, which send immense amounts of debris into the sea, also can cause tsunamis. Scientists naturally would like to know how and to what extent they might be able to predict the features of tsunamis under various circumstances.
Most models of tsunamis generated by landslides are based on the idea that the size and power of a tsunami is determined by the thickness, or depth, of the landslide and the speed of the "front" as it meets the water. In a paper titled "Nonlinear regimes of tsunami waves generated by a granular collapse," published online in the Journal of Fluid Mechanics , UC Santa Barbara mechanical engineer Alban Sauret and his colleagues, Wladimir Sarlin, Cyprien Morize and Philippe Gondret at the Fluids, Automation and Thermal Systems (FAST) Laboratory at the University of Paris-Saclay and the French National Centre for Scientific Research (CNRS), shed more light on the subject. (The article also will appear in the journal's July 25 print edition.)
This is the latest in a series of papers the team has published on environmental flows, and on tsunami waves generated by landslides in particular. Earlier this year, they showed that the velocity of a collapse -- i.e., the rate at which the landslide is traveling when it enters the water -- controls the amplitude, or vertical size, of the wave.
In their most recent experiments, the researchers carefully measured the volume of the granular material, which they then released, causing it to collapse as a cliff would, into a long, narrow channel filled with water. They found that while the density and diameter of the grains within a landslide had little effect on the amplitude of the wave, the total volume of the grains and the depth of the liquid played much more crucial roles.
"As the grains enter the water, they act as a piston, the horizontal force of which governs the formation of the wave, including its amplitude relative to the depth of the water," said Sauret. (A remaining challenge is to understand what governs the speed of the piston.) "The experiments also showed that if we know the geometry of the initial column [the material that flows into the water] before it collapses and the depth of the water where it lands, we can predict the amplitude of the wave."
The team can now add this element to the evolving model they have developed to couple the dynamics of the landslide and the generation of the tsunami. A particular challenge is to describe the transition from an initial dry landslide, when the particles are separated by air, to an underwater granular flow, when the water has an important impact on particle motion. As that occurs, the forces acting on the grains change drastically, affecting the velocity at which the front of grains that make up the landslide enters the water.
Currently, there is a large gap in the predictions of tsunamis based on simplified models that consider the field complexity (i.e., the geophysics) but do not capture the physics of the landslide as it enters the water. The researchers are now comparing the data from their model with data collected from real-life case studies to see if they correlate well and if any field elements might influence the results.
- Natural Disasters
- Drought Research
- Environmental Issues
- Underwater explosion
- Ocean surface wave
- Hurricane Katrina
- Seismic wave
- Megatsunami
- 2004 Indian Ocean earthquake
Story Source:
Materials provided by University of California - Santa Barbara . Original written by James Badham. Note: Content may be edited for style and length.
Journal Reference :
- Wladimir Sarlin, Cyprien Morize, Alban Sauret, Philippe Gondret. Nonlinear regimes of tsunami waves generated by a granular collapse . Journal of Fluid Mechanics , 2021; 919 DOI: 10.1017/jfm.2021.400
Cite This Page :
Explore More
- Anticoagulant With an On-Off Switch
- Sleep Resets Brain Connections -- At First
- Far-Reaching Effects of Exercise
- Hidden Connections Between Brain and Body
- Novel Genetic Plant Regeneration Approach
- Early Human Occupation of China
- Journey of Inhaled Plastic Particle Pollution
- Earth-Like Environment On Ancient Mars
- A 'Cosmic Glitch' in Gravity
- Time Zones Strongly Influence NBA Results
Trending Topics
Strange & offbeat.
Thank you for visiting nature.com. You are using a browser version with limited support for CSS. To obtain the best experience, we recommend you use a more up to date browser (or turn off compatibility mode in Internet Explorer). In the meantime, to ensure continued support, we are displaying the site without styles and JavaScript.
- View all journals
- My Account Login
- Explore content
- About the journal
- Publish with us
- Sign up for alerts
- Open access
- Published: 06 July 2020
Rapid identification of tsunamigenic earthquakes using GNSS ionospheric sounding
- Fabio Manta 1 , 2 nAff4 ,
- Giovanni Occhipinti ORCID: orcid.org/0000-0001-9323-3185 3 , 4 ,
- Lujia Feng ORCID: orcid.org/0000-0002-3736-5025 1 &
- Emma M. Hill ORCID: orcid.org/0000-0003-0231-5818 1 , 2
Scientific Reports volume 10 , Article number: 11054 ( 2020 ) Cite this article
3996 Accesses
23 Citations
5 Altmetric
Metrics details
- Natural hazards
The largest tsunamis are generated by seafloor uplift resulting from rupture of offshore subduction-zone megathrusts. The rupture of the shallowest part of a megathrust often produces unexpected outsize tsunami relative to their seismic magnitude. These are so called ‘tsunami earthquakes’, which are difficult to identify rapidly using the current tsunami warning systems, even though, they produce some of the deadliest tsunami. We here introduce a new method to evaluate the tsunami risk by measuring ionospheric total electron content (TEC). We examine two M w 7.8 earthquakes (one is a tsunami earthquake and the other is not) generated in 2010 by the Sunda megathrust, offshore Sumatra, to demonstrate for the first time that observations of ionospheric sounding from Global Navigation Satellite System (GNSS) can be used to evaluate the tsunamigenic potential of earthquakes as early as 8 min after the mainshock.
Similar content being viewed by others
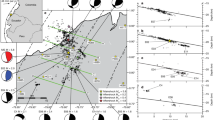
Seismological evidence for a multifault network at the subduction interface
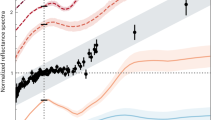
Asteroid Kamo‘oalewa’s journey from the lunar Giordano Bruno crater to Earth 1:1 resonance
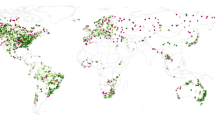
Global prediction of extreme floods in ungauged watersheds
Introduction.
‘Tsunami earthquakes’, as originally defined by Kanamori 1 , are events generating tsunami with larger amplitude than expected from their seismic magnitude. Most tsunami earthquakes are generated by high levels of slip on the shallow megathrust, which results in large seafloor uplifts and hence very dangerous tsunami. The shallow location of the slip—close to the subduction trench—means that the ruptures generating tsunami earthquakes are at significant distance from land-based monitoring networks, limiting our ability to quickly and accurately assess their magnitude and source parameters. Conventional approaches using various seismological methods 2 , 3 , 4 or rapid inversion of GNSS (Global Navigation Satellite System) estimates of ground motion 5 regularly encounter difficulties in accurately estimating the uplift of the seafloor and consequently fail in predicting the tsunamigenic nature of tsunami earthquakes. These events are generated in the relatively soft accretionary wedge and are characterized by slow rupture velocities, and therefore lower levels of seismic energy radiation 6 , 7 ; they are thus only weakly felt by nearby populations, prohibiting natural warnings.
In addition to seismic waves propagating within the solid Earth, tsunamigenic earthquakes can induce waves in the atmosphere, including the ionosphere. Firstly, the uplift of the seafloor at the source generates an acoustic-gravity pulse (AGW epi ) that propagates through the atmosphere above the epicentral area (Fig. 1 ). This perturbation travels at the speed of high-atmospheric acoustic waves (~ 1,000 m/s) and reaches the ionosphere approximately 8 min after the event (Fig. 1 ) 8 . Due to the short response time, this ionospheric perturbation can provide critical information for rapidly assessing the tsunami potential. Secondly, if an ocean tsunami is generated, another perturbation later reaches the ionosphere. A tsunami is essentially an oceanic gravity wave; consequently, by linear dynamic coupling, it produces an atmospheric internal gravity wave (IGW tsuna ), which reaches the ionosphere approximately 40 min after the event (Fig. 1 ) 9 . During the upward propagation, the generated AGW epi and IGW tsuna are both strongly amplified by the double effects of the exponential decrease of the atmospheric density ρ with altitude and the conservation of the kinetic energy ρv 2 , where v is the local speed oscillation induced by wave crossing. At ~ 300 km altitude in the ionosphere, the AGW epi and IGW tsuna are amplified enough to induce strong perturbations in the plasma density. These perturbations can be observed by ionospheric monitoring techniques, such as measurements of Total Electron Content (TEC) by GNSS (see 10 for a review) or airglow cameras 11 , 12 . The TEC represents the number of electrons integrated along the ray-path between the GPS satellite and the station (see “ Material and methods " for more details about TEC calculation). The signature of tsunamigenic earthquakes in the GNSS-derived TEC is consequently detectable in both the near-field by observing the AGW epi directly related to the uplift of the seafloor 13 , 14 and the far-field by observing the IGW tsuna related to tsunami waves 9 , 11 , 15 , 16 , 17 .
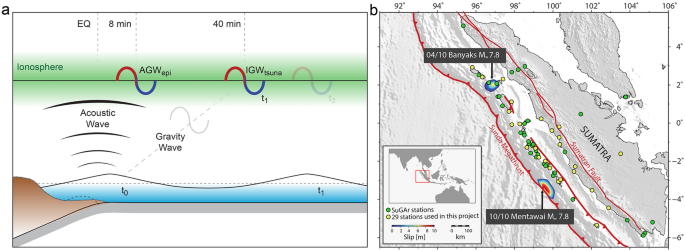
( a ) Schematic of the two ionospheric signals associated with a tsunamigenic earthquake. At the time of the earthquake (t 0 ), the sudden rupture of the fault causes an uplift of the sea floor and subsequently triggers a tsunami. Acoustic Gravity Waves (AGW epi ) associated with the uplift propagate through the atmosphere and reach the ionosphere ~ 8 min after the rupture. The tsunami is an oceanic internal gravity wave; consequently, by a linear coupling, it produces an atmospheric Internal Gravity Wave (IGW tsuna ). The IGW tsuna reaches the ionosphere at time t 1 , ~ 40 min after the tsunami is initiated. ( b ) Main geological features of the Sumatran subduction zone. The contoured areas represent estimated coseismic rupture patches for the 6 April 2010 M w 7.8 Banyaks earthquake in the north 19 , and the 25 October 2010 M w 7.8 Mentawai earthquake in the south 20 . The yellow dots represent the SuGAr stations existing and available at the time of the events, while the green dots represent the current total extension of the SuGAr network.
Based on the relation between the AGW epi, which reaches the ionosphere approximately 8 min after an event, and the uplift of the seafloor, we investigate the potential of using GNSS observations of ionospheric TEC to discriminate tsunami earthquakes. We propose a technique to validate the risk estimated by conventional seismological methods, based on quantification of the intensity of the AGW epi , to discriminate the tsunamigenic nature of an earthquake. As a case study, we examine two M w 7.8 megathrust earthquakes that occurred in 2010 along the Sumatran subduction zone, which were both recorded by the Sumatran GPS Array (SuGAr) (Fig. 2 ). Although both events had the same magnitude, and were issued similar tsunami early warnings (later canceled), the 6 April 2010 Banyaks earthquake 18 , 19 , did not generate a significant tsunami and caused only minor earthquake-related damage to local infrastructure. On the contrary, the 25 October 2010 Mentawai event produced a large tsunami—recorded within 10 min of the earthquake by the Mentawai GPS buoy—which claimed more than 400 lives from communities along the coastlines of the Pagai Islands 7 , 20 , 21 (see “ Material and methods " for a comprehensive description of the two events and the corresponding responses of tsunami warning systems).
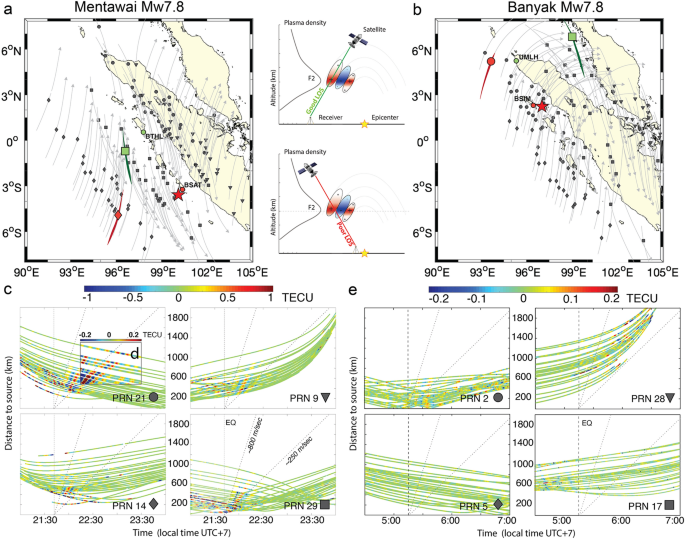
( a ) Map of the distribution of ionospheric piercing points (IPPs) for the pairs of 29 GNSS stations and the four closest satellites at the time of the Mentawai earthquake (local time: 21:42:23 UTC + 7) ( b ) Map of the distribution of ionospheric piercing points (IPPs) for the pairs of 29 GNSS stations and the four closest satellites at the time of the Banyaks earthquake (local time: 5:15:02 UTC + 7). The IPPs are represented by four different symbols corresponding to different satellites; the symbols are located at positions along the trace of the IPPs corresponding to the event time. Note the bold traces highlighting the trajectory of the satellite-receivers pairs discussed in Fig. 3 . The red stars represent the epicenter of the earthquakes. The cartoon of “Good LOS” (green) and “Poor LOS” (red) configurations is shown between a and b . ( c ) Hodochrones of the TEC perturbation observed by GPS satellites PRN 21, 9, 14, and 29. ( d ) Inset with color-scale 5 times smaller to highlight the gravity waves related to the IGW tsuna recorded by PRN 21. ( e ) Hodochrones of the TEC perturbation observed by GPS satellites PRN 2, 28, 5, and 17. Color-scale in ( e ) is 5 time smaller than ( c ). Vertical dashed lines represent the time of the event. The gray dashed lines show the speed of the AGWepi (800 m/s) and IGWtsuna (250 m/s).
Our analysis of the ionospheric response to these two events provides a unique natural experiment to test if the ionosphere is sensitive to the rupture properties of earthquakes and tsunami genesis, and whether GNSS-TEC observations are capable of identifying the events that have a high level of tsunami potential.
At the time of the Mentawai earthquake, 29 stations of the SuGAr network were in operation (Figs. 1 b and 2 a), while 25 stations were operating at the time of the Banyaks earthquake (Figs. 1 b and 2 b). In both cases, more than eight GPS satellites were visible in the sky, and we selected the four that better detected the events with comparable observation geometries. Cahyadi and Heki 22 observed that changes of the penetration angles of the line-of-sight (LOS) with respect to the AGW epi wave front propagating in the ionosphere can influence the amplitude of the detected signals. The authors referred to “good LOS” as the most favorable scenario for which the ionospheric piercing point (IPP) is located between the epicenter and the GNSS station (Fig. 2 ), in this configuration the LOS crosses mainly the positive part of the wave front returning a strong TEC signal. On the contrary they referred to “poor LOS” as the scenario for which the GNSS station is located between the epicenter and the IPP. With this second geometric configuration the LOS simultaneously penetrates the positive and negative parts of the anomaly, partially cancelling each other and consequently returning a weak signature in the GNSS-derived TEC. Moreover, as a consequence of the integrated nature of TEC along the station-satellite LOS, satellites at low elevation angles have a better detection capability 8 (see Supplemental Figs. 1 and 2 ).
Accordingly, to be able to compare the TEC signatures of the two events analyzed in this work, we selected (from Fig. 2 ) four satellite-station pairs (Fig. 3 ) with similar angles and distances from the epicenter area that better represent the different LOS scenarios: Good LOS, with receiver-satellite pairs BTHL-PRN29 and UMLH-PRN17 for the Mentawai and Banyak Islands respectively; and Poor LOS, with BSAT-PRN14 and BSIM-PRN2 for the Mentawai and Banyak Islands respectively. During the Mentawai event the TEC shows a perturbation related to the AGW epi (Fig. 2 ) with a frequency signature between 1 and 7 mHz (Fig. 3 a,b) appearing 8 min after the main shock (21:42:23 local time, UTC + 7) and propagating away from the epicenter at a horizontal speed of 600–800 m/s (Fig. 2 c). Later, a second weaker TEC perturbation at a lower frequency (~ 1.5 mHz), related to the IGW tsuna appears to travel at a horizontal speed of ~ 250 m/s (Fig. 2 d), consistent with the tsunami speed of ~ 220 m/s observed by DART buoy 56,001, located ~ 1,600 km from the epicenter 6 . The arrival time of IGW tsuna observed at the distance of 480 km from the epicenter (Fig. 3 a) is coherent with the tsunami propagation. A much smaller TEC perturbation followed the Banyaks earthquake (Figs. 2 e, 3 c,d), which generated a much weaker tsunami that did not cause any damage (see “ Material and methods " section).
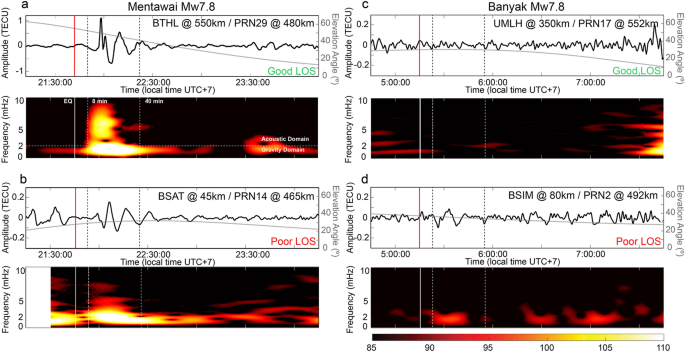
Filtered ionospheric TEC time series and related spectrograms extracted by ( a ) observations of satellite PRN29 with respect to station BTHL ( b ) observations of satellite PRN14 with respect to station BSAT at the time of the 2010 M w 7.8 Mentawai earthquake ( c ) observations of satellite PRN17 with respect to station UMLH and ( d ) observation of satellite PRN2 with respect to station BSIM at the time of the 2010 M w 7.8 Banyaks earthquake. Grey curves represent the elevation angle of the satellite-receiver ray path. Solid red and white vertical lines indicate the time of the events. Dashed vertical lines indicate the time of the first potential arrival of the AGW epi (8 min) and the minimum time of IGW tsuna observation (40 min) in the ionosphere. Horizontal dashed lines are the Brünt-Vaïsalla frequency that represents the limit between gravity and acoustic domains.
Figure 3 shows that regardless the LOS scenario, the Mentawai earthquake induces a greater signature in the GNSS-TEC highlighting the important amount of energy transferred in the ionosphere by this event. On the contrary for the Banyaks earthquake the TEC signature remains very weak also with a Good LOS geometry. Indeed, the GNSS-TEC traces in the hodochrones in Fig. 2 c,f show that the satellite coverage is dense enough to assure the favorable observation of the AGW epi and avoid attenuation effects related to the GNSS LOS geometry 22 .
Spectral analysis (Fig. 4 ) of observed TEC during the days before and after the Mentawai event reveals the unique characteristics of the AGW epi compared to the mean background level (MBL). The Ionospheric Tsunami Power Index (ITPI) is here introduced to define the ratio between TEC perturbation and MBL in order to remove the ionospheric dynamics, and consequently be able to compare the ITPI computed in different ionospheric conditions (see “ Material and methods " section). We highlight that the ITPI is related to the entire spectrum of energy of the AGW epi and consequently provides an estimate of the strength of a perturbation detected in the ionosphere. Compared to the dTEC amplitude estimation used by previous authors, our approach is not affected by frequency pass-band filtering or polynomial fit to obtain the perturbation of TEC. The ITPI for the Mentawai event was 14, as TEC observations revealed a perturbation 14% larger than the MBL (Fig. 4 ). A comparable energetic signature related to external localized phenomena (e.g., plasma bubbles, traveling ionospheric disturbances) appeared on 22 October 2010 but this was not related to a seismic event and consequent tsunami early warning, showing the importance to couple ionospheric observations with conventional techniques. Weaker TEC perturbations followed the Banyaks earthquake, at approximately 5% of the MBL (Supplemental Fig. 3 ), confirming the presence of a weaker tsunami (ITPI = 5). The ITPI measured for the two events shows a correlation with the values of seafloor uplift reported by Cahyadi and Heki 22 , by the United States Geological Survey (USGS, https://earthquake.usgs.gov/earthquakes/ ), and by Hill et al. 20 . We complete our observations for the Mentawai and Banyak Islands with TEC observations for additional 17 tsunamigenic events extracted by Cahyadi and Heki 22 and listed in Table 1 . We correlated the TEC amplitude (blue symbols in Fig. 5 ) with the seafloor uplift and, for comparison, with our new ITPI. To better quantify the contribution of the seafloor uplift, we introduce a weighting factor here referred as the seafloor maximum volume displaced (V max ). This parameter is obtained from the product of the maximum seafloor uplift and the rupture area (A) (see “ Material and methods " section). Seafloor uplift is in reality quite spatially variable, often poorly constrained by slip inversions, and likely to be supplemented by inelastic uplift that is not accounted for in most models. However, this parameter can provide a first-order proxy for comparison with the ITPI. To emphasize the originality of our work we highlight that Cahyadi and Heki 22 correlate the TEC (%) perturbations with the moment magnitude of the events and not with the consequent seafloor maximum volume displaced (V max ), which takes into account also the extent of the seafloor uplift directly related to the tsunami genesis. Our empirical model, showed in Fig. 5 , supports the possibility of obtaining information about the tsunami genesis from ionospheric perturbations.
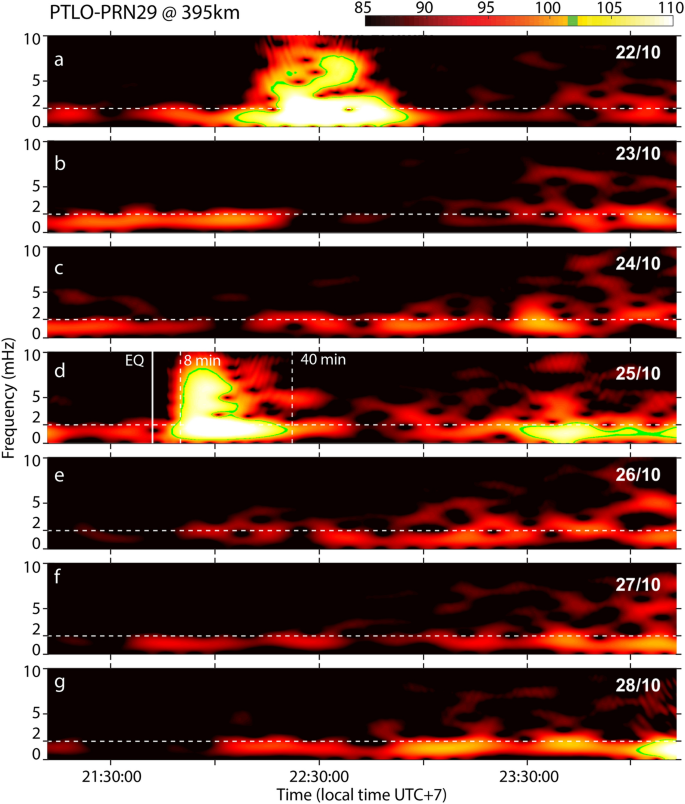
Spectrograms of the filtered TEC signal recorded by the pair of station PTLO and satellite PRN29 at the epicenter of the 2010 M w 7.8 Mentawai earthquake over seven consecutive days. Horizontal dashed lines are the Brünt-Vaïsalla frequency that represents the limit between the gravity and the acoustic domain. Vertical lines in the central panel ( d ) indicate, respectively from left to right: the time of the event (solid line); the first potential arrival of the AGW epi (8 min, dashed line); the minimum time of IGW tsuna observation (40 min, dashed line) in the ionosphere. Green contour lines mark where the intensity of the signal is above a threshold value that represents the mean background level (MBL) calculated on quiet days.
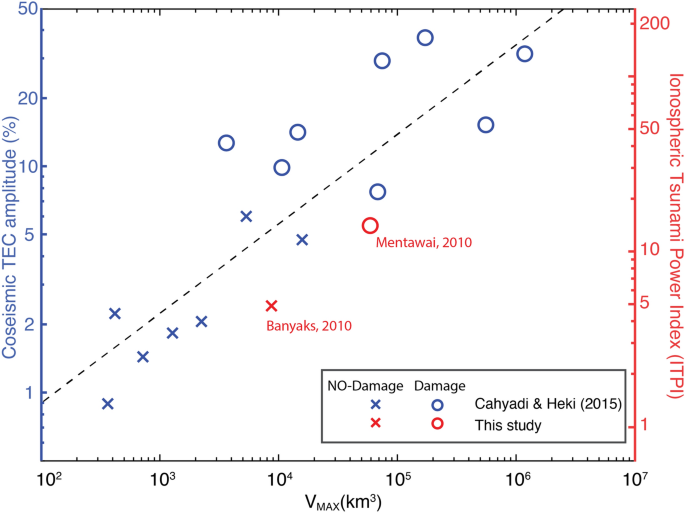
Relation between seafloor maximum volume displaced (V max ), coseismic TEC amplitude and ITPI for 17 events. Blue symbols represent earthquakes from the literature 22 , 24 , 25 . Red symbols represent the Mentawai and Banyak earthquakes discussed in this paper for which both the coseismic TEC amplitude and the ITPI have been computed. The left axis represents the TEC coseismic amplitudes normalized by background vertical TEC estimated from Global Ionospheric Maps (GIM) as defined by Cahyadi and Heki 22 . The right axis represents the ITPI for the Mentawai and Banyak events scaled proportionally to the computed coseismic TEC amplitude. X symbols represent events that generated a very small tsunami that did not cause damage. O symbols represent events that did generate a tsunami and damage.
Coupled with early seismic observations of an earthquake (estimating magnitude and area of slip), the detection of the AGW epi by GNSS-TEC could provide valuable additional information—at the optimistic scenario of 8 min following the initiation of earthquake rupture—on the level of seafloor uplift and thus the potential of a significant tsunami. We highlight that the amplitude of the ionospheric TEC perturbation induced by the AGW epi is directly related to the seafloor maximum volume displaced (V max ) (Fig. 5 ) and we do not need to intermediately evaluate the magnitude of the event or the source parameters to estimate the tsunami risk. Additionally, estimation of GNSS high-rate position has been demonstrated as a valid technique for source estimation and tsunami risk evaluation to complement seismic networks (e.g., 5 ). Thus, we can use the same GNSS receivers for the source estimation and the consequent early tsunami potential evaluation using positioning techniques and validate it using the ITPI analysis. We can confirm the uplift and related tsunami risk by looking for the TEC perturbation induced by the AGW epi within 8 min after the mainshock. After 40 min the TEC perturbation induced by the IGW tsuna gives a direct estimate of the oceanic displacement induced by the tsunami 17 propagating in the open ocean. The IGW tsuna signature in the TEC has been already observed in real-time 23 , proving the potential role of ionospheric monitoring in real-time early warning.
With this work, we prove the maturity of ionospheric observations for evaluating tsunami potential, further showing the capability to discriminate the tsunamigenic nature of slow rupture events characterized by a low level of seismic energy radiation. In particular, estimations of ITPI could be fed into existing tsunami warning systems as an additional piece of information to alert for the presence of anomalous tsunami earthquakes and/or guard against false warnings. We hope to open a new paradigm in tsunami warning systems, based on the redundancy of information from the synergy of classic and new techniques to reduce damages and loss of life.
Material and methods
To investigate the TEC variations caused by the 2010 Mw 7.8 Mentawai and Banyaks earthquakes, we analyzed 15-s data from 29 and 25, respectively, continuous GNSS stations of the SuGAr network that were in operation on the day of each earthquake (Fig. 2 ). We analyzed the GNSS data for the week centered on the day of each earthquake to obtain the ionospheric perturbations before, during, and after the events.
We calculated the TEC by applying a method similar to the one described by Calais and Minster 26 . To obtain a more accurate measure of the apparent distance between a satellite and receiver, we used the carrier phase (L1 & L2), neglecting the less precise pseudo-range measurements (P1 & P2):
where, f 1 and f 2 are, respectively, the corresponding high and low GPS frequency and TEC is the total electron content measured in TEC units (1 TECU = 10 16 el/m 2 ). We detected and corrected the cycle slips using the program Ninja within the GPS-Inferred Positioning System and Orbit Analysis Simulation Software (GIPSY-OASIS) version 6.2 27 .
As a consequence of the integrated nature of TEC, the observed ionospheric perturbations result from a large range of altitudes from the satellite to the receiver. However, the main contribution to the observed TEC variations is located around the height of the maximum of ionosphere ionization (the F2 layer). For this study, we fixed the altitude of the F2 layer at 300 km, which can be considered as the average altitude for day and night for the Equator. The intersection of the line of sight between each GNSS satellite-receiver pair at this altitude is termed the ionospheric pierce point (IPP), which we use to spatially visualize the observed TEC perturbations. In order to identify perturbations caused by the earthquake and the subsequent tsunami, we filtered all the initial TEC time series on a defined range of frequencies following the same methodology as Rolland et al. 28 . We applied a 1 to 10 mHz bandpass finite impulse response (FIR) Butterworth filter in order to remove the contributions from daily ionospheric variabilities, satellite motions, and instrumental biases.
We introduce for the first time the Ionospheric Tsunami Power Index (ITPI) to rapidly identify tsunamigenic earthquakes:
The ITPI corresponds to the ratio between the maximum level of power spectral density of the TEC (PSD MAX ) recorded during the event, and the mean background level (MBL; defined as the maximum level of PSD recorded during the 6 days preceding the earthquake, averaged by the number of days). By averaging the background noise for several days prior to the event, we are able to remove outliers and smooth the noisy parts related to other perturbations traveling in the ionosphere. This new parameter could allow us to automatically identify the seafloor uplift and the consequent tsunamigenic potential of an event (Fig. 5 ). The relation for the Mentawai and Banyak events can be observed in Fig. 5 , which shows, on the right side, the ITPI scale for the two events. The same relation with the seafloor maximum volume displaced V max can be observed for the Coseismic Ionospheric Disturbance (CID), which is shown on the left side for both the Mentawai and Banyak events and 17 earthquakes described in the literature 22 and listed in Table 1 . To calculate the seafloor maximum volume displaced (V max ), we multiply the maximum uplift value for the rupture area (A). Values of uplift are provided by the US Geological Survey (USGS, https://earthquake.usgs.gov/earthquakes/ ) resulting from finite fault, calculated using Okada-style deformation codes 29 . We highlight that the ITPI is a more accurate way to estimate the ionospheric perturbation as it is calculated in the spectral domain and it is consequently free of eventual pass-band filter applied to CID.
Tsunami warning response to the two Mw 7.8 Mentawai and Banyaks earthquakes
We analyzed two M w 7.8 earthquakes (Fig. 2 ) that occurred in 2010 along the Sunda megathrust (Indonesia): The 6 April Banyaks earthquake 18 , 19 and the 25 October Mentawai earthquake 7 , 20 , 21 . Although they had the same magnitude, only the Mentawai earthquake produced an anomalously large tsunami.
The Banyaks earthquake ruptured a deeper portion (20–30 km) of the megathrust, producing relatively small uplift of the seafloor and thus only a small tsunami (max water height: 44 cm, max run up: 6 m, source NOAA database). On the other hand, the Mentawai earthquake was a shallow event with estimated slip concentrated at depths of < 6 km, no more than ~ 50 km away from the trench. Given the lower rigidity of the shallow sediments at this location, the Mentawai event generated considerably higher levels of slip 20 , 30 than the Banyaks event 18 while maintaining the same moment magnitude. The reported shake intensity at 150 km distance from the epicenter was MMI 5 and MMI 6 for the Mentawai, and for the Banyaks events, respectively. The high slip at shallow depths resulted in seafloor uplifts of up to several meters, producing the outsize tsunami with a reported maximum run up > 16 m 20 .
For the Mentawai event, the Badan Meteorologi Klimatologi Dan Geofisika (BMKG) of Indonesia initially estimated the magnitude as 7.2 and issued a tsunami warning within 5 min of the earthquake. The warning was afterward cleared without receiving information of tsunami damage 21 . The German Indonesian Tsunami Early Warning System (GITEWS) recorded the tsunami on a surface GPS buoy located in the Mentawai islands (GITEWS SUMATRA-03) within 10 min of the earthquake, with amplitudes of ~ 15 cm and a period of a few minutes 21 , 30 . Later the tsunami arrival was recorded by the Padang tide gauge about 1 h after the earthquake, and by the DART station 56,001 located around 1,600 km southeast of the epicenter about 2 h after the earthquake 21 , 30 . However, no tsunami warning was issued because some buoys had been vandalized 31 and were not in operation. For the Banyaks event, BMKG also recognized a tsunami potential and immediately issued a tsunami warning that was canceled later, as only a minor tsunami was generated. For both events, the Pacific Tsunami Warning Center (PTWC) did not issue a warning, but a less severe tsunami watch, which was also canceled later (~ 2 h after). The GEOFON global seismological broadband network operated by the German GeoForschungsZentrum (GFZ) produced a real-time model for the two earthquakes, estimating a magnitude of M w 7.6 for the Banyaks event and a magnitude of M w 7.8 for the Mentawai event.
The Mentawai event did not provide a natural warning: witnesses reported feeling only weak, long-period shaking 20 . While many of the people on the islands knew to evacuate to higher ground on feeling strong earthquake shaking, they did not recognize this event was so dangerous. The characteristics of tsunami earthquakes make them particularly dangerous and exemplify the need for additional warning systems.
The current state of the art for tsunami early warning
Due to difficulties in accuractely estimating the source extent, conventional approaches – such as seismological methods—encounter difficulties in estimating the tsunamigenic potential of an earthquake. This problem has been addressed by various studies. Convers and Newman 32 developed a new method to rapidly discriminate between normal and slow ruptures events, such as those of tsunami earthquakes 3 , 7 . Kanamori and Rivera 2 developed a method based on W-phases to determine seismic source parameters for tsunami warning purposes. This method can be used to reliably determine magnitude within ~ 20 min of the event 33 . Recent studies have shown that real-time GPS and seismic data can also be used in a combined approach 5 . This technique allows for rapid determination of the size of the source within few minutes from the rupture 34 , resulting in a more effective analysis compared to the seismic analysis alone, which suffers from saturation at large magnitudes. Melgar et al. 34 tested their algorithm for rapid magnitude estimation with high-rate GPS data for a large number of events, with the Mentawai event being the least successful one. The uncertainties in the magnitude estimation for this particular event highlight again that additional techniques for rapid identification of tsunami earthquakes could improve current warning systems.
Several techniques that imply direct approaches, such as the use of offshore instruments, have been extensively applied for tsunami detection. NOAA developed and deployed the Deep-ocean Assessment and Reporting of Tsunami (DART). DART consists of a sea floor bottom pressure recorder and a moored surface buoy ( https://www.ndbc.noaa.gov/dart/dart.shtml ). Nevertheless, a DART buoy is primarily suitable to detect a far-field tsunami, as they are deployed and anchored at least 250 miles away from the shore 33 thus being less useful for near-field events. GPS buoys and tide gauges, such as these deployed by the Nationwide Ocean Wave information network for Ports and HArbourS (NOWPHAS) near the Japanese coastline, and by the GITEWS, which was operating along the Sumatran coast between 2005 and 2011 35 , have the potential to provide short time tsunami warning within 10 min of the earthquake. However, in addition to damage caused by weather conditions, floating buoys may be also subject to vandalism, particularly in marine water that is favorable to fishing 36 . Servicing damaged buoys can be challenging and costly.
Other ocean-bottom seismographic and tsunami observation systems, based on fiber-optics submarine cables, have also been developed for tsunami detection 37 . These techniques are based on monitoring seafloor earthquakes to detect tsunami. However, these systems come with deployment costs that are usually prohibitively high. More recently, Xerandy et. al. 38 proposed a new method based on an underwater communication system composed of fiber optic cables and an undersea network of sensors that could give a 20-min warning time.
It is clear that any additional information that gives rapid identification of the tsunami potential resulting from an earthquake would be useful for the success of tsunami early warning systems. In this study, therefore, we analyze the ionospheric response to two test events of the same seismic magnitude, which provide a unique natural experiment to test if the ionosphere is sensitive to the rupture properties and tsunami genesis, and whether GNSS-TEC observations are able to identify the events that have a high level of tsunami potential.
Data availability
The datasets generated during and/or analysed during the current study are available in the DR-NTU repository, https://doi.org/10.21979/N9/3KUEM5 .
Kanamori, H. Mechanism of tsunami earthquakes. Phys. Earth Planet. Int. 6 , 346–359 (1972).
Article ADS Google Scholar
Kanamori, H. & Rivera, L. Source inversion of W phase: Speeding up seismic tsunami warning. Geophys. J. Int. 175 , 222–238 (2008).
Convers, J. A. & Newman, A. V. Global evaluation of large earthquake energy from 1997 through mid-2010. J. Geophys. Res. 116 , B08304 (2011).
Duputel, Z., Rivera, L., Kanamori, H. & Hayes, G. W phase source inversion for moderate to large earthquakes (1990–2010). Geophys. J. Int. 189 , 1125–1147 (2012).
Melgar, D., Bock, Y. & Crowell, B. W. Real-time centroid moment tensor determination for large earthquakes from local and regional displacement records. Geophys. J. Int. 188 , 703–718 (2012).
Lay, T. et al. The 25 October 2010 Mentawai tsunami earthquake (Mw 7.8) and the tsunami hazard presented by shallow megathrust ruptures. Geophys. Res. Lett. 38 , L06302 (2011).
ADS Google Scholar
Newman, A. V., Hayes, G., Wei, Y. & Convers, J. The 25 October 2010 Mentawai tsunami earthquake, from real-time discriminants, finite-fault rupture, and tsunami excitation. Geophys. Res. Lett. 38 , L05302 (2011).
Occhipinti, G., Rolland, L., Lognonné, P. & Watada, S. From Sumatra 2004 to Tohoku-Oki 2011: The systematic GPS detection of the ionospheric signature induced by tsunamigenic earthquakes. J. Geophys. Res. Space Phys. 118 , 3626–3636 (2013).
Occhipinti, G., Kherani, E. A. & Lognonné, P. Geomagnetic dependence of ionospheric disturbances induced by tsunamigenic internal gravity waves. Geophys. J. Int. 173 , 753–765 (2008).
Article ADS CAS Google Scholar
Occhipinti, G. The seismology of the planet Mongo: The 2015 ionospheric seismology review. Subduction dynamics: From mantle flow to mega disasters. Geophys. Monogr. 211 , 169–182 (2016).
Google Scholar
Occhipinti, G. et al. Three-dimensional numerical modeling of tsunami-related internal gravity waves in the Hawaiian atmosphere. Earth Planets Space 63 , 847–851 (2011).
Makela, J. J. et al. Imaging and modeling the ionospheric airglow response over Hawaii to the tsunami generated by the Tohoku earthquake of 11 March 2011. Geophys. Res. Lett. 38 , L00G02 (2011).
Article Google Scholar
Astafyeva, E., Lognonné, P., & Rolland, L. First ionospheric images of the seismic fault slip on the example of the Tohoku-oki earthquake. Geophys. Res. Lett. 38 , L22104 (2011).
Astafyeva, E., Rolland, L., Lognonné, P., Khelfi, K. & Yahagi, T. Parameters of seismic source as deduced from 1 Hz ionospheric GPS data: Case study of the 2011 Tohoku-oki event. J. Geophys. Res. Space Phys. 118 , 5942–5950 (2013).
Occhipinti, G., Lognonné, P., Kherani, E. A. & Hébert, H. Three-dimensional waveform modeling of ionospheric signature induced by the 2004 Sumatra tsunami. Geophys. Res. Lett. 33 , L20104 (2006).
Rakoto, V., Lognonné, P. & Rolland, L. Tsunami modeling with solid Earth–ocean–atmosphere coupled normal modes. Geophys. J. Int. 211 , 1119–1138 (2017).
Rakoto, V., Lognonné, P., Roll, L., & Coïsson, P. Tsunami wave height estimation from GPS-derived ionospheric data. J. Geophys. Res. Space Phys. 123 (5), 4329–4348 (2018).
Feng, L. et al. A unified GPS-based earthquake catalog for the Sumatran plate boundary between 2002 and 2013. J. Geophys. Res. Solid Earth. 120 , 3566–3598 (2015).
Morgan, P. M. et al. The diverse slip behavior of the Banyak Islands section of the Sunda Megathrust offshore Sumatra. Am. Geophys. Union, Fall Meet. 2015, Abstr. id. T21D-2858 (2015).
Hill, E. M. et al. The 2010 Mw7.8 Mentawai earthquake: Very shallow source of a rare tsunami earthquake determined from tsunami field survey and near-field GPS data. J. Geophys. Res. Solid Earth . 117 , B06402 (2012).
Satake, K. et al. Tsunami source of the 2010 Mentawai, Indonesia earthquake inferred from tsunami field survey and waveform modeling. Pure Appl. Geophys. 170 , 1567–1582 (2013).
Cahyadi, M. N. & Heki, K. Coseismic ionospheric disturbance of the large strike-slip earthquakes in North Sumatra in 2012: Mw dependence of the disturbance amplitudes. Geophys. J. Int. 200 , 116–129 (2015).
Savastano, G. et al. Real-time detection of tsunami ionospheric disturbances with a stand-alone GNSS receiver: A preliminary feasibility demonstration. Sci. Rep. 7 , 46607 (2017).
Astafyeva, E., Shalimov, S., Olshanskaya, E. & Lognonné, P. Ionospheric response to earthquakes of different magnitudes: Larger quakes perturb the ionosphere stronger and longer. Geophys. Res. Lett. 40 , 1675–1681 (2013).
Astafyeva, E., Rolland, L. M. & Sladen, A. Strike-slip earthquakes can also be detected in the ionosphere. Earth Planet. Sci. Lett. 405 , 180–193 (2014).
Calais, E. & Minster, J. B. GPS detection of ionospheric perturbations following the January 17, 1994, Northridge Earthquake. Geophys. Res. Lett. 22 , 1045–1048 (1995).
Zumberge, J. F., Heflin, M. B., Jefferson, D. C., Watkins, M. M. & Webb, F. H. Precise point positioning for the efficient and robust analysis of GPS data from large networks. J. Geophys. Res. Solid Earth. 102 , 5005–5017 (1997).
Rolland, L. M. et al. The resonant response of the ionosphere imaged after the 2011 off the Pacific coast of Tohoku Earthquake. Earth Planets Space 63 , 853–857 (2011).
Okada, Y. Internal deformation due to shear and tensile faults in a half-space. Bull. Seismol. Soc. Am. 82 (2), 1018–1040 (1992).
Yue, H. et al. Rupture process of the 2010 Mw 7.8 Mentawai tsunami earthquake from joint inversion of near-field hr-GPS and teleseismic body wave recordings constrained by tsunami observations. J. Geophys. Res. Solid Earth. 119 , 5574–5593 (2014).
Suppasri, A. et al. A decade after the 2004 Indian Ocean tsunami: The progress in disaster preparedness and future challenges in Indonesia, Sri Lanka, Thailand and the Maldives. Pure Appl. Geophys. 172 , 3313–3341 (2015).
Convers, J. A. & Newman, A. V. Rapid earthquake rupture duration estimates from teleseismic energy rates, with application to real-time warning. Geophys. Res. Lett. 40 , 5844–5848 (2013).
Comfort, L. K., Znati, T., Voortman, M. & Freitag, L. E. Early detection of near-field tsunamis using underwater sensor networks. Sci. Tsunami Hazards. 31 , 231–243 (2012).
Melgar, D. et al. Earthquake magnitude calculation without saturation from the scaling of peak ground displacement. Geophys. Res. Lett. 42 , 5197–5205 (2015).
Münch, U., Rudloff, A. & Lauterjung, J. Postface “The GITEWS Project - results, summary and outlook”. Nat. Hazards Earth Syst. Sci. 11 , 765–769 (2011).
C.-C. Teng et al. , Buoy vandalism experienced by NOAA National Data Buoy Center. Ocean. 2009 MTS/IEEE Biloxi Mar. Technol. Futur. Glob. Local Challenges . 1 , 26–29 (2009).
T. Schmitz, W. Rutzen, W. Jokat, In International Symposium on Underwater Technology, UT 2007—International Workshop on Scientific Use of Submarine Cables and Related Technologies 2007 , 301–304 (IEEE, New York, 2007).
Xerandy, X., Znati, T. & Comfort, L. K. Cost-effective, cognitive undersea network for timely and reliable near-field tsunami warning. Int. J. Adv. Comput. Sci. Appl. 6 , 224–233 (2015).
Download references
Acknowledgements
Some of the results presented here are reported in the PhD thesis of Dr. Fabio Manta ( https://hdl.handle.net/10356/107577 ), archived by Nanyang Technological University, Singapore. We are grateful to the many scientists and field technicians who have kept the SuGAr network in operation. These include Paramesh Banerjee, Iwan Hermawan, Danny Natawidjaja, Jeffrey Encillo, Imam Suprihanto, Dudi Prayudi, and Bambang Suwargadi. SuGAr GPS data are available for download from ftp://ftp.earthobservatory.sg/SugarData . This research was supported by the National Research Foundation Singapore under its Singapore NRF Investigatorship scheme (Award No. NRF-NRFI05-2019-0009) and by the EOS and the National Research Foundation Singapore and the Singapore Ministry of Education under the Research Centres of Excellence initiative. G.O. is supported by Programme National de Télédétection Spatiale (PNTS), grant n PNTS-2014-07; by the CNES, grant GISnet&Back; and by the Institut Universitaire de France (IUF). We thank Benoit Taisne for useful discussions, and the reviewers for constructive comments. This is EOS contribution 300 and IPGP contribution 4054.
Author information
Fabio Manta
Present address: Université de Paris, Institut de Physique du Globe de Paris, CNRS, 75005, Paris, France
Authors and Affiliations
Earth Observatory of Singapore, Nanyang Technological University, Singapore, Singapore
Fabio Manta, Lujia Feng & Emma M. Hill
Asian School of the Environment, Nanyang Technological University, Singapore, Singapore
Fabio Manta & Emma M. Hill
Institut Universitaire de France, Paris, France
Giovanni Occhipinti
Université de Paris, Institut de Physique du Globe de Paris, CNRS, 75005, Paris, France
You can also search for this author in PubMed Google Scholar
Contributions
F.M. analyzed and interpreted data, made all the figures, and took the lead in drafting the manuscript. G.O. and E.H. designed the research. L.F. helped to analyze the GNSS data. All authors contributed to drafting of the manuscript.
Corresponding author
Correspondence to Fabio Manta .
Ethics declarations
Competing interests.
The authors declare no competing interests.
Additional information
Publisher's note.
Springer Nature remains neutral with regard to jurisdictional claims in published maps and institutional affiliations.
Supplementary information
Supplementary file1, rights and permissions.
Open Access This article is licensed under a Creative Commons Attribution 4.0 International License, which permits use, sharing, adaptation, distribution and reproduction in any medium or format, as long as you give appropriate credit to the original author(s) and the source, provide a link to the Creative Commons license, and indicate if changes were made. The images or other third party material in this article are included in the article’s Creative Commons license, unless indicated otherwise in a credit line to the material. If material is not included in the article’s Creative Commons license and your intended use is not permitted by statutory regulation or exceeds the permitted use, you will need to obtain permission directly from the copyright holder. To view a copy of this license, visit http://creativecommons.org/licenses/by/4.0/ .
Reprints and permissions
About this article
Cite this article.
Manta, F., Occhipinti, G., Feng, L. et al. Rapid identification of tsunamigenic earthquakes using GNSS ionospheric sounding. Sci Rep 10 , 11054 (2020). https://doi.org/10.1038/s41598-020-68097-w
Download citation
Received : 22 October 2018
Accepted : 27 May 2020
Published : 06 July 2020
DOI : https://doi.org/10.1038/s41598-020-68097-w
Share this article
Anyone you share the following link with will be able to read this content:
Sorry, a shareable link is not currently available for this article.
Provided by the Springer Nature SharedIt content-sharing initiative
This article is cited by
Machine learning-based detection of tec signatures related to earthquakes and tsunamis: the 2015 illapel case study.
- Federica Fuso
- Laura Crocetti
- Benedikt Soja
GPS Solutions (2024)
Ionospheric disturbance analysis of the January 15, 2022 Tonga eruption based on GPS data
- Mingzhe Lyu
Science China Earth Sciences (2023)
The GUARDIAN system-a GNSS upper atmospheric real-time disaster information and alert network
- Léo Martire
- Siddharth Krishnamoorthy
- Yoaz E. Bar-Sever
GPS Solutions (2023)
Ahead-of-Tsunami Magnetic Disturbance Detection Using Intrinsic Mode Functions: Tohoku-Oki Earthquake Case Study
- V. Klausner
- H. G. Macedo
Pure and Applied Geophysics (2022)
Tsunami detection by GPS-derived ionospheric total electron content
- Mahesh N. Shrivastava
- Ajeet K. Maurya
- Rafael Aranguiz
Scientific Reports (2021)
By submitting a comment you agree to abide by our Terms and Community Guidelines . If you find something abusive or that does not comply with our terms or guidelines please flag it as inappropriate.
Quick links
- Explore articles by subject
- Guide to authors
- Editorial policies
Sign up for the Nature Briefing newsletter — what matters in science, free to your inbox daily.

- Open access
- Published: 11 October 2023
Connecting community’s perspectives on tsunami risk to anticipated future tsunamis: a reflection from a progress of tsunami preparedness from a coastal community in Aceh-Indonesia after 19 years of the 2004 Indian Ocean Tsunami
- Benazir 1 ,
- Syamsidik 2 , 3 ,
- Yunita Idris 2 , 3 &
- Nadri Pratama Putra 2
Geoenvironmental Disasters volume 10 , Article number: 21 ( 2023 ) Cite this article
1738 Accesses
1 Citations
Metrics details
This paper reflects on the progress of tsunami preparedness in a coastal community in Aceh, Indonesia, nearly two decades after the catastrophic 2004 Indian Ocean Tsunami. The research employs a comprehensive approach to thoroughly evaluate and comprehend the community’s preparedness, its correlation with local perceptions of tsunami risk, and delves into the prevalence of tsunamis in the area, with a specific emphasis on the significant impact of the 2004 Indian Ocean Tsunami on the coastal community of Aceh. To investigate the community’s readiness and the potential impacts of tsunamis at the study site, tsunami simulations were performed using the shallow water equation within the COMCOT (Cornell Multi-grid Coupled Tsunami) model. These simulations assessed run-up and inundation scenarios, thereby providing justification for the potential tsunami impact in the area. Modelling the scenario of tsunami in the region is important to measure the potential impact and estimation time for community to prepare the evacuation plan. In addition to the numerical modeling, a mixed-method approach was employed, involving the distribution of questionnaires and conducting in-depth interviews with 150 respondents directly on-site. These assessments yielded valuable insights into community perspectives on tsunami risk and their preparedness measures. The findings contribute to the development of effective strategies for disaster management by integrating local knowledge, experiences, and socialization programs. The study emphasizes the significance of ongoing endeavors to enhance community preparedness and mitigate the consequences of tsunamis.
Introduction
Seismic activity in the waters off western Sumatra occurs quite intensively. This can be attributed to its complex tectonic setting resulting from the convergence of the Indian Plate with the Eurasian/Indian and Australian Plates (McCaffrey 2009 ). The Sumatra fault, spanning 1900 km with multiple segments, is formed due to the interaction between these plates (Sieh and Natawidjaja 2000 ). This dynamic interaction generates tectonic earthquakes, some of which trigger tsunamis (Haridhi et al. 2018 ; Lange et al. 2018 ; Weller et al. 2012 ).
Over the past two decades, Sumatra has been a focal point of tsunami occurrences in Indonesia, with 60% of all tsunamis impacting the region and inflicting significant land damage. This era of increased tsunamigenic events commenced with the devastating Indian Ocean Tsunami (IOT) on December 26, 2004. The IOT was initiated by an earthquake ranging in magnitude from 9.1 to 9.3 Mw (Ammon et al. 2005 ; Lambotte et al. 2006 ; Park et al. 2005 ; Vallée 2007 ), the variation in magnitude being attributed to varying assumptions about fault geometry. Chlieh et al. ( 2007 ) calculated a magnitude of 9.15 Mw using a coseismic model with a fault dimension of 1500 km in length and 150 km in width. Subsequently, an 8.7 Mw earthquake struck the southeastern side of the IOT source, leading to another destructive tsunami affecting Nias and Simeulue Islands (Hsu et al. 2006 ; Subarya et al. 2006 ). This rupture occurred precisely in the location of an 8.5 Mw earthquake in 1861 (Gahalaut and Catherine 2006 ). In addition to these events, other tsunamis, such as the one in 2005 triggered by a 7.8 Mw earthquake off the Mentawai Islands (Newman et al. 2011 ), have impacted the region. The waters off West Sumatra Province have also witnessed numerous significant earthquakes (exceeding 8 Mw) in the past, as meticulously analyzed by Philibosian et al. ( 2017 ), who studied eight rupture events spanning from 1520 to 2000. Notably, two out of these three major tsunamis directly affected the Aceh mainland in northern Sumatra. Further underscoring the region’s seismic vulnerability, April 11, 2012, marked a historic moment with the occurrence of the largest recorded strike-slip earthquake (8.6 Mw and 8.2 Mw) in history. With only a 2-hour interval between the two events, this unprecedented seismic activity (Delescluse et al. 2012 ; Duputel et al. 2012 ; Lay et al. 2005 ; Wang et al. 2012 ; Yue et al. 2012 ). Geodetic and geomorphic studies assessing the distribution of slip/locking on the Sumatra fault, as conducted by Tabei et al. ( 2017 ), suggest that the potential for significant earthquakes in Aceh remains considerable.
Notably, the historical record extends far into the past, with tsunamis having left their mark on Aceh’s shores for centuries. Paleo-tsunami studies by Monecke et al. ( 2008 ) along the west coast of Aceh (Meulaboh) have unveiled evidence of recurring tsunami damage dating back a millennium. Similarly, investigations by Sieh et al. ( 2015 ) along the Lambaro coast have identified two distinct tsunamis occurring between 1394 and 1396 and 1450–1453. Also, Wassmer et al. ( 2015 ) applied the anisotropy of magnetic susceptibility (AMS) technique to analyze a historical tsunami deposit, uncovering three distinct uprush pulsations and offering crucial insights into the tsunami’s orientation, energy, and flow patterns. A comparative analysis between the 4220 BP tsunami and the 2004 IOT revealed behavior similarities in the Lampuuk coastal plain, attributed to bay topography influencing uprush flow direction. Even more intriguingly, Rubin et al. ( 2017 ) have uncovered ancient tsunamis dating back 7400 years before the IOT through paleo-tsunami studies in a cave on Aceh’s west coast. These studies, complemented by archaeological surveys along a 40 km stretch of the northern coast, which provide evidence of a devastating tsunami around 1391 (Daly et al. 2019 ), underscore the enduring and cyclical nature of tsunamis in Aceh.
Understanding and reconstructing these past events is pivotal in deciphering tsunami characteristics, often accomplished through advanced simulations (Kim et al. 2013 ; Koshimura et al. 2009 ; Martin et al. 2019 ). This knowledge serves as the foundation for regional assessments of tsunami disaster probability, a crucial component of early warning and preparedness efforts (Horspool et al. 2014 ; Mulia et al. 2020 ; Selva et al. 2021 ), aligning with the principles outlined in the Sendai Framework, which emphasize disaster understanding and preparedness enhancement (United Nations Office for Disaster Risk Reduction 2015 ).
The magnitude of the 2004 IOT and its far-reaching impacts classify it as a mega-hazard (Synolakis and Bernard 2006 ). Notably, the coastal population’s experience with tsunamis significantly influences the number of casualties (Imamura et al. 2019 ). The consequences of tsunamis extend to coastal erosion, which affects livelihoods, including damage to fishponds (Griffin et al. 2013 ), creating a prolonged cycle of recovery for survivors. Consequently, the post-disaster period has ushered in a new era in Indonesia’s tsunami mitigation efforts, characterized by the development of disaster management regulations and the implementation of technology as tangible components of tsunami mitigation. Nevertheless, despite these strides, enhancing mitigation systems remains a priority, particularly in light of the impacts of the Palu-Donggala Tsunami and the Anak Krakatau Tsunami in late 2018. Indonesia’s status as a developing country, with limitations in acquiring adequate mitigation systems, presents ongoing challenges.
This research is fundamentally rooted in the need to learn from previous tsunami events in Indonesia, which continue to exact a toll on human lives and land infrastructure. Employing a case study approach, this research aims to evaluate the impact of tsunamis based on historical seismic potential. Furthermore, it seeks to map the level of community preparedness in facing potential tsunamis in the study area.
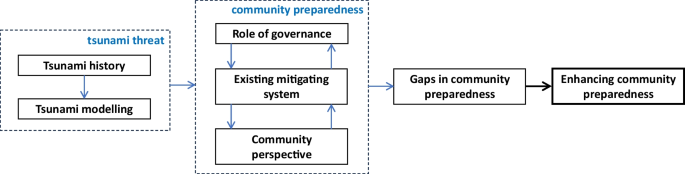
The framework of the idea and research flow
In Fig. 1 , a detailed depiction is provided, showcasing the intricate relationship between tsunami simulation and the assessment of community preparedness. This comprehensive research framework encompasses several interconnected components. It begins with an exploration of Tsunami History, delving into historical seismic and tsunami data, including the 2004 Indian Ocean Tsunami, while identifying recurring tsunami events and their impact on Aceh. The framework then extends to the utilization of a Tsunami Model, where potential scenarios, encompassing run-up and inundation, are simulated. Concurrently, the study evaluates Existing Mitigating Systems, assessing their work and effectiveness in mitigating tsunami impacts. The research goes further by delving into the Community Perspective, conducting an in-depth analysis of community viewpoints regarding tsunami risk. This aspect also scrutinizes how local knowledge and experiences shape these perceptions. Simultaneously, the investigation identifies Gaps in Community Preparedness, discerning weaknesses in the community’s preparedness efforts. It considers the multifaceted factors contributing to these gaps, encompassing cultural, social, and economic dimensions. Finally, the framework addresses the Role of Governance, evaluating the involvement of local governance and authorities in disaster management and scrutinizing the policies and initiatives aimed at enhancing community preparedness.
The theoretical implications of this research extend to the development of an integrated model for coastal zone management, encompassing buffer zones to mitigate tsunami damage on land. On a practical level, the research lays the groundwork for coastal settlement planning and the establishment of Temporary Evacuation Sites (TES) and evacuation routes. For policymakers, implementing this study can serve as a reference in formulating or updating Regional Spatial Planning (RTRW), Coastal and Small Island Zoning Plans (RZWP-3-K), and coastal boundary demarcation.
Study location
The research is focused on the coastal area of Teluk Ulee Lheue, Peukan Bada Subdistrict, Aceh Besar Regency, Aceh Province. Administratively, it is located in the Gampong (village) of Lam Guron, Lambaro Neujid, and Lambaro. These three Gampongs are part of the Lam Pageu Mukim, Peukan Bada Subdistrict, Aceh Besar Regency, Aceh Province. The study location is shown in Fig. 2 , facing the open sea. Lam Pageu Mukim was selected as the study location based on several considerations. This area is vulnerable to direct tsunami attacks, as evidenced by the impact of the 2004 tsunami and the absence of physical mitigation systems in the field. Additionally, there is available open space to support implementing tsunami mitigation systems. Generally, the local population’s livelihoods are in the agricultural and fishing sectors, as farmers and fishermen (Badan Pusat Statistik 2020 ). The selection of this location is based on the vulnerability to tsunamis, both historically and the potential assessed through tectonic order assessments in the Indian Ocean. Furthermore, after the 2004 Indian Ocean Tsunami, there has been no physical mitigation implementation except for socialization and evacuation signage efforts (Syamsidik et al. 2019 ).
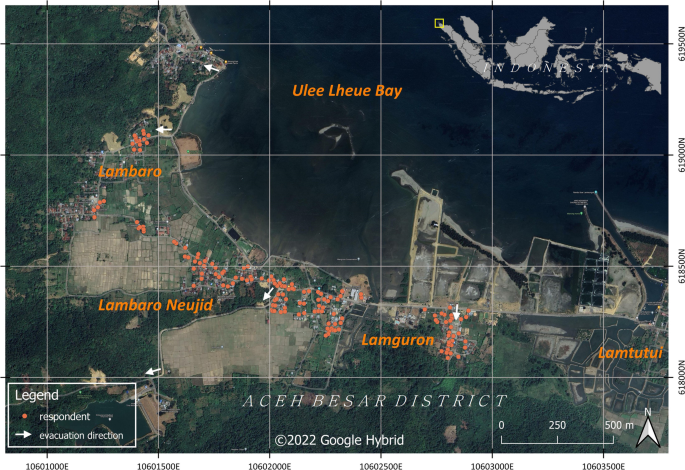
Aerial view of a portion of the study location (Gampong Lambaro Neujid and Lamguron) post-tsunami (left) and after recovery (right)
The area’s topography is relatively flat, with elevations ranging from 2 to 4 m above sea level for the coastal mainland. This coastal area is used for fishing and agricultural purposes, including ponds and rice fields. The settlements are located along the coastal fringe, with several public facilities such as mosques, health centers, and schools. Approximately 500 m from the coast, the topography becomes steeper, characterized by hills surrounding the area. An aerial view of the study area is shown in Fig. 3 . The aerial perspective in the photo also reveals the spatial arrangement of the Lam Pageu Mukim post-tsunami and after recovering from the impacts of the 19-year-old Indian Ocean Tsunami.
The 2004 Indian Ocean Tsunami significantly affected Aceh’s coastal areas, including coastline changes, land use, community settlements, and infrastructure (Syamsidik et al. 2019 ). In addition to the direct impacts on these sectors, there were significant changes in the social aspects of coastal communities following the tsunami. The characteristics of the tsunami that struck the study area were mega hazards, with recorded tsunami heights of 20 m and 13.7 m on the hill slopes of Gampong Lambaro Neujid (Lavigne et al. 2009 ). The recorded wave heights were influenced by the proximity of the tsunami source to the Aceh coast, categorizing it as a near-field tsunami. Thus, the large-scale waves caused significant damage to the mainland, particularly in the agricultural and fishing sectors. The presence of mangrove greenbelts in the study location did not play a significant role in reducing the impacts of the tsunami. On the contrary, positive effects were observed in the coastal areas of India and Sri Lanka, where the presence of coastal forests was able to reduce the damage caused by a tsunami with heights less than 7 m (Dahdouh-Guebas et al. 2005 ; Danielsen et al. 2005 ; Griffin et al. 2013 ).
The damage to the fishing and agricultural sectors subsequently impacted the livelihoods of the local communities. In the Ulee Lheue Bay coastal area, significant damage occurred to the pond and rice field areas along the coastal mainland. Out of the 149 hectares of damaged pond areas, only 28 hectares (19%) have been successfully recovered ten years since the tsunami (Fahmi et al. 2017 ). The slow recovery rate in the pond fishery sector is due to substantial damage (erosion) and salinity factors in some ponds (Syamsidik et al., 2015 ). The recovery process in the agricultural sector, particularly rice fields, contrasts with the situation in the pond fishery sector. Out of the pre-tsunami 189 hectares of rice fields, 80% of these fields have gradually recovered (Fahmi et al. 2017 ). These data indicate that the agricultural sector has experienced more significant recovery than the pond fishery sector, suggesting a dominant shift in the local community’s livelihoods towards post-tsunami farming. The mobilization efforts for changing livelihoods after the 2004 Indian Ocean Tsunami are post-disaster adaptation conditions (Ismail et al. 2018 ).
The level of preparedness of coastal communities within a broader area has been assessed by Syamsidik et al. (2021). Their study results for 9 districts in Aceh showed that community preparedness in facing tsunamis falls under the “good” category. Oktari et al. ( 2020 ) also mapped the level of community preparedness at the city/district level, specifically in Banda Aceh City. Based on their findings, the knowledge about coastal disasters and emergency response plans falls under the “moderate” category, while the parameters of early warning systems and resource mobilization fall under the “low” category.
In general, the research framework, as depicted in Fig. 1 , encompasses both primary data collection and mathematical modeling as its data acquisition methods. Primary data consists of input data for the model and a description of the study location. Primary data for simulation purposes are obtained through measurements and field observations at the study location. Inventory and investigation are conducted to assess the existing topography and land use. Contour elevation mapping is performed to obtain surface elevation data, while bathymetry data is obtained from secondary sources. Land use mapping is carried out by capturing aerial photographs using a drone. On the other hand, a study of social aspects is necessary for assessing the level of community preparedness. This data is obtained through the distribution of questionnaires and direct interviews with the local community.
Numerical Tsunami modeling
The Cornell Multigrid Coupled Tsunami (COMCOT) model by Liu et al. ( 1995 , 1998 ) is used to simulate the generation, propagation, and inundation of tsunamis in Ulee Lheue Bay. The latest version of COMCOT published can be referenced in Wang ( 2009 ). This model solves the nonlinear long wave equations coupled with bottom friction using the Finite Difference Method with an explicit leapfrog scheme. This model has been widely used in simulating both historical and modern tsunami events (Li et al. 2015 ; Sepúlveda et al. 2020 ; Wang and Liu 2006 ).
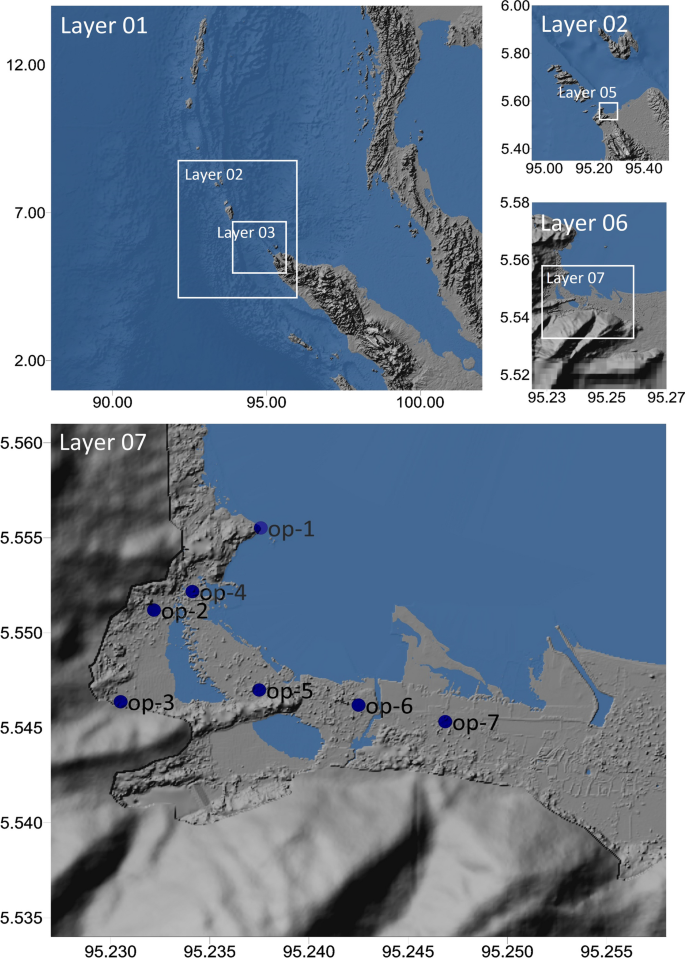
Seven-layer computational domain
The numerical computation domain includes the tsunami generation initiation location, which is the Aceh-Andaman fault segment, and the study location in Aceh, Sumatra Island. To obtain wave propagation details on land and computational duration, a nested grid configuration is implemented. The computational domain is categorized into seven layers, as shown in Fig. 4 . Bathymetry data for Layers 1 and 2 are sourced from Topex and downloaded through https://topex.ucsd.edu/cgi-bin/get_srtm30.cgi . The National Bathymetry Data (BATNAS), downloaded through https://tanahair.indonesia.go.id/demnas/#/batnas , is used for Layers 3 and 4. Layers 5–7 combine BATNAS and topographic data obtained from field measurements. Further information on the entire computational domain is summarized in Table 1 .
The initial conditions for tsunami generation are simulated using the models by Mansinha and Smylie ( 1971 ) and Okada ( 1985 ). The determination of earthquake magnitude scenarios is based on investigating earthquake scales and past tsunami-generating sources that have impacted the study location. Three earthquake magnitude scenarios are defined to simulate potential tsunamis based on their potential and previous tsunami history: 8.2, 9.15, and 9.2 Mw. The 8.2 Mw earthquake scenario represents a smaller tsunami than the latest event. This magnitude is based on the last tsunami threat in the southwest waters of Aceh on April 11, 2012. On the same day, two major earthquakes occurred, with the first one measuring 8.6 Mw (330 km southwest of the 2004 event’s epicenter) and the second one occurring two hours later with a magnitude of 8.2 Mw, located approximately 180 km south of the first location (Delescluse et al. 2012 ). This scenario is referred to by Tursina et al. ( 2021 ), projecting tsunami inundation and sea level rise in the Banda Aceh region, which is located east of this study location. The second scenario with a 9.15 Mw earthquake represents a reconstruction of the 2004 tsunami with rupture parameters proposed by Koshimura et al. ( 2009 ). In addition to these two scenarios, the National Earthquake Study Center updated the earthquake zoning map 2017. Geodetic measurements obtained a slip rate of 4 mm/year (Pusgen 2017 ). The Aceh-Andaman Segment rupture 2004 was 1300 km long (Lay et al. 2005 ). Using the equations (Hanks and Kanamori 1979 ) and (Wells and Coppersmith 1994 ), the maximum magnitude of this segment was estimated to be 9.2 Mw. Thus, based on this assessment, the third scenario is determined as a potential tsunami with a larger scale (hypothetical model) affecting the study location. Further details of the tsunami initiation scenarios and their parameters are presented in Table 2 .
Survey of community preparedness level
The knowledge and response of the community play a crucial role in developing a mitigation system. The role of the local community is not only considered as the object of study but also as pioneers in conducting self-evacuation and preserving the evacuation system for future generations. In this research, the assessment of community knowledge is conducted through direct interviews with several respondents in the field. This assessment activity is carried out at the initial stage of the research to determine the study location, apart from other technical aspects. The number of respondents is determined based on Eq. 1 , which applies the Slovin Formula.
where n is the number of respondents, N is the population size, and e represents the percent of allowable non-sampling error or desired tolerance. Referring to the data from the Central Statistics Agency (Badan Pusat Statistik 2020 ), the population size in the study location, which includes three villages, is 1390 individuals (Table 3 ). With a selected e value of 10%, the number of respondents is calculated to be 93 individuals. To obtain better results and consider data integrity, 150 respondents were assessed in this study.
The data collection method uses probability sampling, a sampling technique where each member of the population has an equal chance of being selected as a sample. In other words, all individual members of the population have a non-zero probability. The criteria for respondents include being residents of the study location, whether native or migrants (as long as they are not visitors), at least 17 years old (adolescents), no distinction between 2004 tsunami victims or non-victims, no distinction based on educational background and occupation, the respondents consist of both males and females and each individual does not represent a household (multiple individuals from one household are allowed as long as the previous criteria are met). The exclusion criteria are limited to visitors (tentative residents) and individuals below 17. The assessment material is revolved around understanding how coastal communities in the study area perceive, prepare for, and respond to the ever-present threat of tsunami hazards, with a particular emphasis on assessing their knowledge, emergency preparedness plans, awareness of early warning systems, and their viewpoints concerning mitigation strategies.
In this multifaceted inquiry, key questions have been formulated to delve into diverse facets of coastal community dynamics. Primarily, the investigation seeks to uncover the extent of community awareness regarding tsunami hazards, particularly in relation to the 2004 incident. This encompasses the recognition of indicators, understanding of tsunami effects, and comprehension of unique characteristics or indications. Furthermore, the sources from which individuals and communities typically obtain their tsunami-related information are of interest. Secondly, the examination focuses on exploring the extent to which local communities have created and executed emergency response strategies. This includes the preparation of first aid supplies and kits, participation in evacuation training, procurement of essential resources, protection of documents, and retention of contact information for vital institutions. Thirdly, the investigation aims to gauge community knowledge of tsunami early warning systems, including sirens and means of obtaining warnings, as well as their awareness of government-issued alerts. Finally, the perspective of the local community on mitigation concepts, such as reducing the impact of tsunamis and preserving coastal areas, is explored. This exploration encompasses a wide range of aspects, from the complexities and costs involved to environmental considerations and the appeal to tourists.
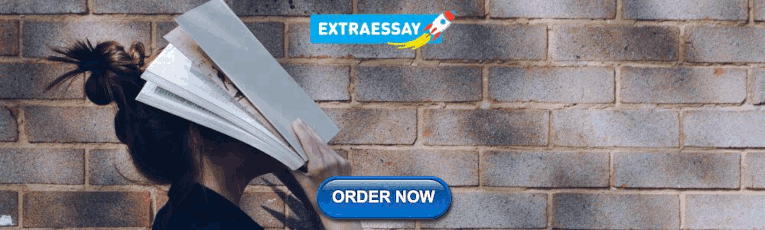
Results and discussion
Tsunami potential.
Numerical modeling of tsunamis triggered by earthquakes involves approximating the initial wave shape using analytical solutions proposed by Mansinha and Smylie ( 1971 ) and Okada ( 1985 ). These solutions represent the surface water disturbance resulting from fault displacement at the seafloor for strike-slip and dip-slip faults, respectively. The length and width of the fractured area on the fault plane influence the scale of the generated tsunami. In the case of mega-tsunamis, such as the 2004 event, a multi-fault approach is employed to better represent the field conditions by dividing the fault into fault segments. However, for the 8.2 Mw scenario, a single fault is considered due to the rupture length being only 217 km.
Conversely, a six-segment fault approach is adopted for the other two scenarios with Mw > 9 (rupture length > 1000 km). The initial conditions of the tsunami formation for the three scenarios are shown in Fig. 5 , positive wave heights are illustrated in light blue, and negative wave heights are depicted in dark blue. These wave heights are measured in meters and represent the variations in wave elevations for the three scenarios considered in our study. Specifically, in the 8.2 Mw scenario, the positive wave height is recorded at 2.06 m, and the negative wave height is registered at − 1.30 m. In the 9.15 Mw scenario, these values are measured at 10.78 m and − 3.53 m, respectively. Finally, in the 9.2 Mw scenario, the positive wave height is observed to reach 11.29 m, while the negative wave height is documented at − 3.62 m. All scenarios also indicate a pre-tsunami receding of the sea level in the waters off Sumatra. Numerous reports from witnesses and victims indicate that the arrival of the tsunami was preceded by a sea level retreat during the 2004 Indian Ocean Tsunami (IOT) in Aceh, Thailand, and surrounding areas (Satake 2014 ; Tsuji 2006 ).
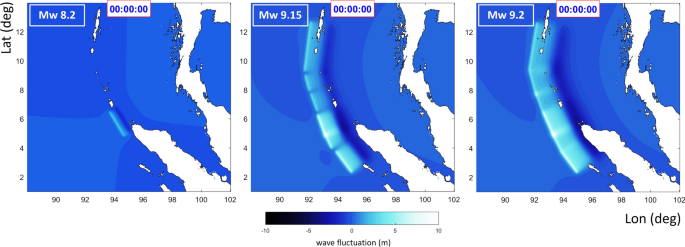
Initial conditions for the three simulated scenarios
The inundation impacts of the tsunami on land are depicted in Fig. 6 for all three scenarios. In the case of an 8.2 magnitude earthquake, flooding occurs in all villages within the study area. The extent of inundation in Lambaro Neujid village reaches 400 m. Despite the smaller scale of the tsunami event compared to the previous one, the reach of the waves is influenced by the topographic conditions. Paddy fields in this village lead to a wider inundation due to the lower elevation of the fields relative to the sea level (see Fig. 3 ). The change in topography from flat to steep, represented by the hill behind Lambaro and Lamguron villages, results in a shorter inundation distance in these villages compared to Lambaro Neujid. The widest extent of inundation is observed in Lamteungoh village due to its proximity to the sea and relatively gentle topography. Additionally, the influence of the tsunami inundation from the east (Peukan Bada) also affects this village.
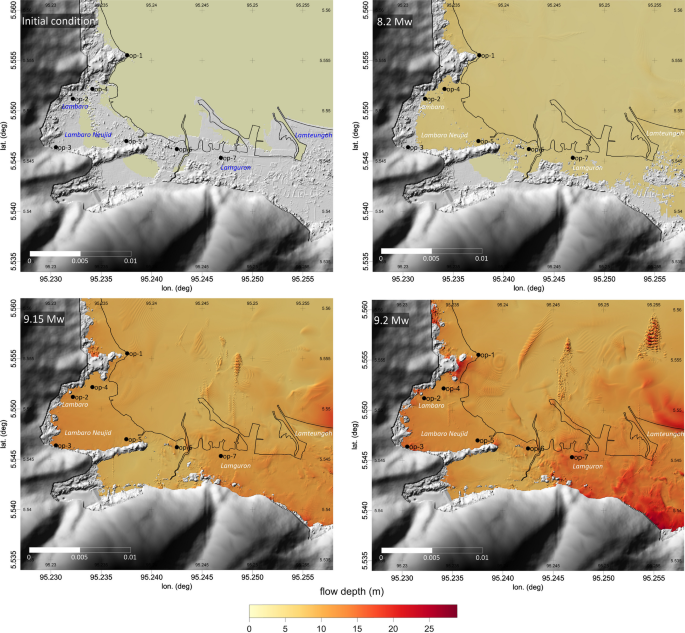
Maximum inundation depth for the three scenario conditions
The tsunami’s impact becomes much more remarkable for scenarios generated by a 9.15 Mw earthquake (scenario 2). The inundation length in Lambaro Neujid reaches 493 m from the shoreline. This distance would be even greater if there were no hills behind the settlement, as observed in the area behind Lamteungoh village, where the inundation reaches a length of 675 m. The inundation areas also starkly contrast the previous scenario, particularly in the eastern part of Lambaro Neujid. The tsunami inundation even reaches Embung Lambadeuk. The 9.15 magnitude tsunami scenario aims to represent the tsunami’s strength in 2004 considering the current land use conditions (19 years after the tsunami). However, this scenario is not intended to reconstruct the 2004 IOT due to the different topographic and land use conditions.
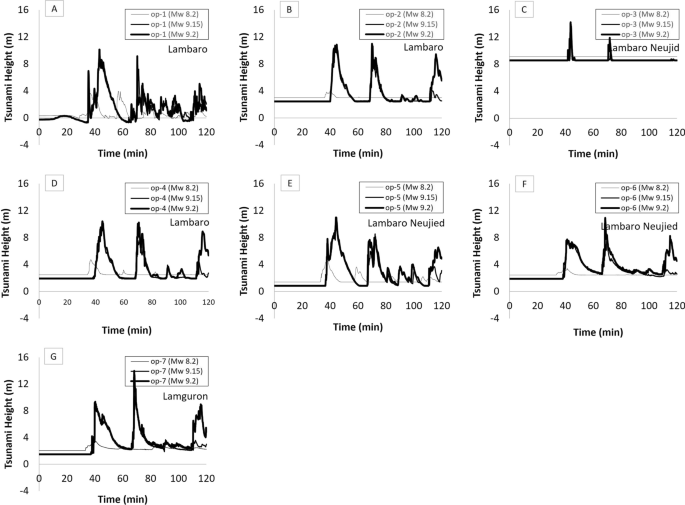
The height of tsunami fluctuations at observation points op-1 to op-7
In scenario 3, with a magnitude of 9.2 Mw, there is no significant difference compared to the scenario representing the earthquake scale during the previous tsunami, as proposed by Koshimura et al. ( 2009 ). However, the height and extent of inundation are slightly larger than those for the 9.15 Mw scale. A comparison of the maximum inundation achieved for the 9.15 Mw and 9.2 Mw scenarios is presented in Fig. 7 .
The heights of the tsunamis on the land were observed at several locations (observation points, op) spread across 7 points within the study area (indicated by black dots in Fig. 6 ). Three observation stations in Fig. 6 correspond to the same reference points as the post-survey conducted by Lavigne et al. ( 2009 ). The post-survey results recorded inundation depths of 12.3 m, 20 m, and 13.7 m for op-1, op-2, and op-3, respectively. The modeling results for the 2004 IOT, represented by the orange lines in Fig. 7 a–c, indicate differences in the elevation of inundation compared to field data. As previously discussed, scenario 2 (9.15 Mw) cannot fully represent the 2004 Tsunami reconstruction due to the changed topographic and land use conditions. Furthermore, bathymetry data, serving as secondary data, play a significant role in determining tsunami characteristics, especially in shallow waters, including the influence of seabed roughness. The delay in the arrival of tsunami waves to the coast, linked to both wave elevation and celerity, can be ascribed to the geographical location of the area and the influence of the local bathymetry, which has the capability to either amplify or diminish the magnitude of the waves as they propagate towards the shoreline. These conclusions align with previous scientific investigations that underscore the significance of the local bathymetry in shaping the characteristics of tsunamis (Murotani et al. 2015 ).
Figure 7 d–g present the measurements of tsunami inundation in each village ( gampong ) within the study area for all modeling scenarios. These figures also reveal two primary waves arriving at the shore with a time difference of 20 min. Lambaro, located furthest to the west, experiences a tsunami of approximately 3 m for the small earthquake scenario and around 7–8 m for earthquakes more significant than 9 Mw. A tsunami with a depth of 4.3 m (8.2 Mw) and 9–10 m (> 9 Mw) inundates the western side of Lambaro Neujid. On the eastern side of the area, wave heights range from 3 m for the 8.2 Mw generation to 6.3–8 m for earthquakes more significant than 9 Mw, influenced by the presence of hills that obstruct waves from the west. In Lamguron, a tsunami inundation of 3.7 m occurs for scenario 1, while it ranges from 9 to 10 m for scenarios 2 and 3.
Knowledge and community preparedness
This study aims to assess the knowledge and perceptions of coastal communities to understand and evaluate their preparedness for tsunami disasters. The study area is a vulnerable region directly impacted by a tsunami two decades ago. The experiences of these coastal communities play a significant role in realizing their preparedness and resilience in mitigating future tsunami events. The distribution of respondents interviewed can be seen in the previously mentioned Fig. 2 (marked with orange dots). The parameters evaluated in this study include the basic knowledge of the community about tsunami phenomena and their impacts, emergency response plans as preparedness efforts for disasters, and the adaptation of early warning systems as a manifestation of mitigation efforts.
The assessment results based on the parameter of basic knowledge about tsunami phenomena are presented in Fig. 8 . The local community has a good understanding that earthquakes generate tsunamis. However, only 42% are aware that underwater landslides can also trigger tsunamis. The rarity of tsunamis caused by landslides has resulted in a need for more information for the community. Based on records from 1666 to 1999, tsunamis in Indonesia were primarily triggered by earthquakes (90%), followed by volcanic eruptions accounting for 8%, and only 1% were caused by landslides (Latief et al. 2000 ). The recent tsunamis that struck the coastal areas of the Sunda Strait less than four years ago did not receive much attention from the local population. However, the experience of experiencing a disaster serves as valuable knowledge for the community. After an earthquake, most of the community understands that the receding of sea waters is a sign of an impending tsunami (88%). The 2004 Indian Ocean Tsunami experience has become the basis of knowledge for the community that the receding of sea waters always precedes a tsunami phenomenon. However, it should be noted that not all tsunami events (whether triggered by earthquakes or non-earthquake factors) are preceded by sea water receding, as was the case in the 2010 Mentawai Tsunami. Other tsunami arrival indicators, such as the roaring sound from the sea before the tsunami inundates the land, are also known by the community (85%) and changes in animal behavior (52%).
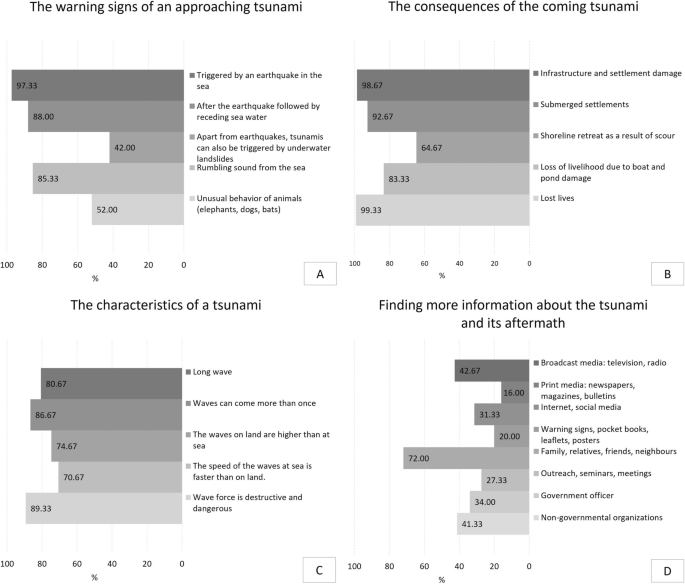
Assessment results regarding general knowledge of tsunami disasters
The community is well aware that the impacts of tsunamis result in loss of life, damage to settlements and other infrastructure, and loss of livelihoods. The severity of past tsunami events remains ingrained in the community’s memories, especially for respondents who were direct victims of the 2004 Indian Ocean Tsunami, experiencing the loss of family members or material possessions. 75.33% or 113 out of 150 respondents interviewed for this study were victims of the 2004 Indian Ocean Tsunami. However, knowledge of shoreline retreat due to tsunami-induced erosion is only understood by a portion of the community (64.67%). The recovery process of the Ulee Lheue Bay shoreline has been relatively successful after 19 years. However, there are still areas, such as abandoned fishponds, where the shoreline recovery has yet to be fully achieved. The younger generation of respondents needs to be fully aware of the changes in the shoreline that occurred after the tsunami. Monitoring shoreline recovery stages in the study area can be referred to in Syamsidik et al. (2015).
The community possesses a reasonably good understanding of tsunami characteristics, with an approximate 75–89% range. They agree that tsunamis are long waves, waves can arrive more than once, wave height is much greater when it reaches the shore compared to when it is in the sea, wave velocity is faster at sea than when it reaches the land, and the force carried by a tsunami is highly destructive and dangerous.
The community’s primary source of tsunami information is primarily obtained through interactions among family members, relatives, friends, and neighbors, accounting for 72%. This factor is influenced by the local culture of gathering and discussing in coffee shops and other public places. Information media such as television and radio also increase community knowledge (42.67%). Socialization efforts carried out by non-governmental organizations or community-based organizations also impact local community knowledge. Additionally, information is received by the community through government socialization, print media, and interactions with the internet and social media. In recent years, social media has experienced rapid development and is favored by the public as a means of information dissemination. Approximately 31% of the community accesses disaster-related information through social media, influenced by the generational differences among the respondents. Social media has the potential for widespread dissemination of disaster conditions, whether providing real-time information during a disaster or conveying lessons learned from past disasters (Simon et al. 2015 ). However, it should be noted that there is also the potential for misinformation. It was also observed in the field that meetings or seminars involving the community have a positive role in enhancing knowledge, such as knowledge dissemination forums conducted by several tsunami researchers in the field.
The form of emergency response plans as community preparedness efforts for future tsunami threats is shown in Fig. 9 . Almost 95% of the populace has successfully established designated assembly areas and routes for evacuation in case of calamities. The study area incorporates evacuation indicators, depicted by white arrows in Fig. 2 , which delineate the path to shelters. All the shelters are strategically positioned in the vicinity of the hill situated behind the villages. The local residents have a comprehensive understanding of the evacuation signage that has been well-established and acknowledged within the study area. The study area has well-recognized and understood evacuation signs for the local residents. Other preparedness efforts include around 43.33% and 41.33% of the community participating in training or evacuation drills (tsunami drills) and formulating evacuation plans and shelter locations. The community also prepares necessary supplies for emergencies, such as clothing, cash, important telephone numbers, and essential documents (24–30%). However, only a small portion of the community (less than 15%) implements household-level emergency protocols, such as preparing first aid kits or emergency medications, identifying safe spots within their homes, and practicing evacuation plans with their families. The low awareness of building a culture of preparedness at the household level is of particular concern. The short evacuation time during a disaster highlights that the success of mitigation efforts depends on the community’s swift response in self-evacuation. Therefore, self-rescue success depends on planning with various scenarios (such as the timing of the disaster, family members present at home during the event, and others) and practicing evacuation drills together with family members. There is an initiative to create Standard Operating Procedures (SOPs) at the household level related to emergency preparedness. These SOPs allocate specific tasks or roles to each family member during a disaster event, aiming to enhance preparedness and response.
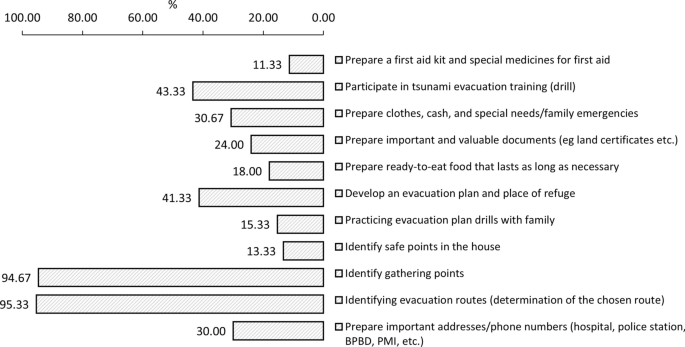
The assessment results regarding the emergency response plan
The significance of this implementation can be observed in the case of the 2012 double earthquake event. On that day, earthquakes with magnitudes of 8.6 Mw and 8.2 Mw occurred, triggering evacuation orders for coastal areas. Nevertheless, timely siren activation was prevented by an electrical outage and the absence of an available power backup system (Syamsidik et al. 2021 ). Additionally, the lack of trained personnel to operate the siren towers further complicated the situation, resulting in delays in evacuation. Despite these challenges, it is noteworthy that a spontaneous evacuation process initiated by the people played a significant positive role. This spontaneous response represented a marked improvement compared to the 2004 Indian Ocean Tsunami, highlighting the importance of community-level preparedness and the need for further improvement in emergency protocols.
Figure 10 displays the local community’s knowledge of early warning systems. This parameter represents the community’s awareness of the existing early warning system. Following the 2004 tsunami, sirens were installed in the Aceh region as part of the tsunami early warning system and the alerts issued by the Meteorology, Climatology, and Geophysics Agency (BMKG) government agency. Most of the community is familiar with the tsunami sirens (93.33%) and knows they are sounded on the 26th of each month and can be heard in people’s homes (86.67%). These data indicate that while some individuals are unaware of the existence of tsunami sirens (16.67%), there are also those who are aware of the sirens but not of their monthly periodic sounding (6.66%). Knowledge about early warnings is predominantly obtained from community leaders and intercommunity stories (76%), compared to religious institutions (9.33%), community organizations’ outreach (8.67%), and print media (4%). The community is also aware of the tsunami alerts issued by the BMKG after an earthquake (78.67%). This information is primarily acquired by the community through influential figures and cross-community stories (71.33%), as well as through local government district/village outreach (57.33%) and community organizations’ outreach (28%).
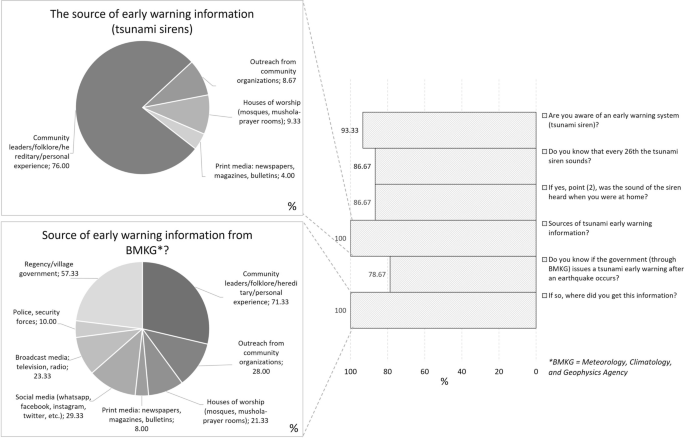
The assessment results regarding the community’s response to early warnings
The relationship between community preparedness and tsunami potential
The inventory of knowledge and community response towards tsunamis indicates the level of community preparedness. The level of preparedness is excellent concerning knowledge about tsunamis. Most of the community had firsthand experience with the tsunami 19 years ago, while others were indirectly affected. The knowledge gained through these experiences has become a strong memory that can be implemented as an asset in facing future disasters. Additional knowledge regarding tsunami characteristics must be shared with the community to enhance their resilience. Knowledge has been disseminated among the community through socialization efforts, stakeholder involvement, and community leaders. However, encouraging direct participation in disaster preparedness activities would positively impact disaster mitigation (Witvorapong et al. 2015 ).
The concept of a systematic emergency response plan plays a crucial role in responding to actions that need to be taken during a limited timeframe when a disaster occurs. According to the tsunami simulation scenario corresponding to each village, the projected duration for the waves to arrive at the coastline amounts to a total of 20 min. In the event of a minor earthquake, the anticipated height of the waves may descend to roughly 3 m in the westernmost region, whereas a more substantial earthquake could result in a wave height of approximately 7–8 m. Consequently, given the limited timeframe available for self-preservation, it is imperative for the community to accurately ascertain the most suitable routes for evacuation as well as identify appropriate locations for vertical evacuation. The application of emergency response protocols at the household level needs to be higher. Implementing protocols within households is essential for self-evacuation. It begins with determining the response each family member needs to take when an earthquake occurs and extends to assigning tasks among family members during self-evacuation. Scenarios can be incorporated into household-scale emergency response plans, such as considering the presence of family members outside the house when a disaster strikes, determining meeting points, and other relevant factors.
Early warning parameters indicate the level of community preparedness in making evacuation decisions. The existence of tsunami sirens serves as a device that emphasizes the limited time available for evacuation during damage or human error (Oktari et al. 2014 ). Local wisdom within the community is critical to saving lives during tsunamis. The Simeulue community exemplified this during the 2004 event, where the continuous narration of Smong across generations has built disaster awareness and serves as a form of early warning knowledge (Rahman et al. 2018 ).
It is important for local authorities to inform residents about the tsunami arrival time and how to recognize the potential ground shaking that could lead to a tsunami. The emphasis placed by local authorities on evacuation preparation time can be reduced by preparing grab-and-go kits well before an earthquake strikes (Chen et al. 2022 ).
The study area in the Peukan Bada District remains highly vulnerable to tsunamis along the Ulee Lheue Bay coast. Previous simulation results showed that the inundation distance in the Lampageu area extends up to 1 km inland. For small-scale earthquakes compared to the 2004 event, the tsunami height in this area ranges from 3 to 4 m, with the run-up reaching 500 m towards residential areas. Therefore, in addition to strengthening community preparedness, implementing an integrated mitigation system that considers the environment in the area is crucial. A mitigation system provides additional time for the community to conduct self-evacuation.
A connection between the community and the theoretical and practical implications based on the findings could be suggested for each stakeholder. For the community, the research holds the potential to inform and guide various actions, including raising awareness about tsunami risks, organizing regular evacuation drills, and promoting community-driven disaster preparedness efforts. In the governmental and regulatory context, the implications encompass recommendations for measures such as implementing regulations to manage coastal development, formulating comprehensive coastal zone management plans, and strategically constructing tsunami shelters in vulnerable areas.
Furthermore, the development of mitigation concepts in the Ulee Lheue Bay coastal area should consider the social aspects of the community and the environmental conditions. The mitigation concept should also be integrated with the existing mitigation system, such as evacuation routes. The damaged aquaculture ponds along the coastline resulting from previous tsunamis can be utilized as buffer zones. These buffer zones can be established by planting coastal vegetation along the shoreline, with Casuarina equisetifolia (coastal casuarina) being a recommended vegetation species compared to mangroves due to the limited success of mangrove species in this location (Syamsidik et al., 2015). Similar coastal characteristics found in locations such as Lembah Geuruete (Benazir et al., 2017) and Pacitan Bay (Muhari et al. 2012 ) are also present in the study area. However, to allow coastal vegetation to grow and provide protection against wave impacts, the damaged aquaculture ponds from 2004 need to be reclaimed. These practical suggestions facilitate the translation of theoretical insights into real-world applications, ultimately strengthening tsunami preparedness and mitigation efforts within the community and enhancing overall resilience.
Nevertheless, this study has limitations associated with its close association with local geographic conditions, requiring adjustments in geographical settings when applied to other areas. Additionally, from a modeling perspective, limitations exist regarding bathymetric data, which are mainly secondary, and the number of scenarios conducted, as only the two most recent tsunamis (2012 and 2004) and one worst-case scenario with potential were modeled. Furthermore, the modeled tsunami sources were based solely on tectonic earthquake mechanisms, while the waters north of Sumatra also have the potential for tsunamis generated by submarine landslides (Haridhi et al. 2022 ).
This investigation examining the simulation of a tsunami has yielded valuable insights into the potential impact of earthquakes of varying magnitudes on the formation of tsunamis and the resulting flooding of coastal areas. The modeling approach employed in this study took into consideration critical factors such as fault displacement, rupture length, and the topographical characteristics of the region under investigation. Importantly, the findings of this study demonstrate that even a relatively modest earthquake with a magnitude of 8.2 Mw can lead to significant flooding in the affected villages, highlighting the crucial role played by local topography in determining the extent of inundation. As earthquake magnitudes exceed 9 Mw, the impact of the resulting tsunami escalates significantly, resulting in higher wave heights and more extensive areas being flooded. This research also highlights notable differences in the scale and depth of flooding depending on the magnitude of the earthquake and the unique topographic features of each village. Notably, the scenario with an earthquake magnitude of 8.2 Mw resulted in flooding across all villages in the study area, with Lamteungoh experiencing the most extensive inundation due to its proximity to the sea and relatively gentle topography. These findings underscore the need to consider dynamic changes in topography and land use over time when conducting assessments of tsunami risk. Furthermore, the study emphasizes the complexity of tsunami modeling and the requirement for comprehensive data, particularly in relation to the influence of local bathymetry on tsunami characteristics and the observable delay in the arrival of waves. This study has made a significant contribution to our understanding of the hazards posed by tsunamis and has emphasized the importance of effective disaster preparedness and measures to mitigate the impact of tsunamis in regions that are vulnerable to such events.
Moreover, this study provides insight into the critical factors that influence the preparedness of communities and the potential for reducing the impact of tsunamis. The findings illustrate the significant roles played by community knowledge and direct experiences of tsunamis in shaping the preparedness levels of communities. Ongoing efforts to disseminate knowledge through socialization programs and the active involvement of stakeholders have contributed to the development of increased community awareness. However, the study highlights the need to further enhance preparedness by actively encouraging direct participation in disaster preparedness activities.
Furthermore, this study highlights the importance of establishing a systematic emergency response plan at the household level to enable effective and timely actions during a tsunami event. Clearly defining roles and responsibilities for each family member and incorporating various scenarios into the emergency response plan are considered essential steps to improve preparedness.
In addition, early warning systems, including tsunami sirens, are identified as crucial tools for alerting communities and providing limited time for evacuation. The integration of local wisdom, which includes traditional knowledge passed down through generations, along with modern early warning systems, has been shown to enhance community preparedness and response capabilities.
Nevertheless, it is important to acknowledge the limitations of this study. The findings are inherently associated with the specific geographical conditions of the study area, and adjustments would be necessary when extrapolating them to different regions. Furthermore, enhancing the comprehensiveness of bathymetric data and expanding the range of modeling scenarios, such as incorporating submarine landslides, would undoubtedly strengthen the findings of this study.
In conclusion, this study serves as a valuable asset for advancing the preparedness of communities and mitigating the adverse effects of tsunamis. It highlights the importance of disseminating knowledge, actively involving communities, implementing early warning systems, and combining traditional wisdom with modern approaches. By implementing comprehensive mitigation systems and utilizing appropriate coastal vegetation, vulnerable coastal regions can strengthen their ability to withstand the imminent threat of tsunamis. Future research efforts should prioritize adapting these findings to various geographical contexts and expanding the range of modeling scenarios to further enhance preparedness and mitigation endeavors.
Ammon CJ, Ji C, Thio HK, Robinson D, Ni S, Hjorleifsdottir V, Kanamori H, Lay T, Das S, Helmberger D, Ichinose G, Polet J, Wald D (2005) Rupture process of the 2004 Sumatra-Andaman earthquake. Science 308(5725):1133–1139. https://doi.org/10.1126/science.1112260
Article CAS Google Scholar
Badan Pusat Statistik (2020) Kecamatan Peukan Bada dalam Angka 2020. BPS Kabupaten Aceh Besar
Triatmadja R, Rahardjo AP, Yuwono N (2017) Comparison of methods for simulating Tsunami Run-up through Coastal forests. Sci Tsunami Hazards 36(3):167–182
Google Scholar
Chen C, Wang H, Lindell MK, Jung MC, Siam MRK (2022) Tsunami preparedness and resilience: evacuation logistics and time estimations. Trans Res D Trans Environ 109:103324
Article Google Scholar
Chlieh M, Avouac JP, Hjorleifsdottir V, Song TRA, Ji C, Sieh K, Sladen A, Hebert H, Prawirodirdjo L, Bock Y, Galetzka J (2007) Coseismic slip and afterslip of the great Mw 9.15 Sumatra-Andaman earthquake of 2004. Bull Seismol Soc Am. https://doi.org/10.1785/0120050631
Dahdouh-Guebas F, Jayatissa LP, di Nitto D, Bosire JO, Seen D, lo, Koedam N, (2005) How effective were mangroves as a defence against the recent tsunami? Curr Biol. https://doi.org/10.1016/j.cub.2005.06.008
Daly P, Sieh K, Seng TY, McKinnon EE, Parnell AC, Ardiansyah, Feener RM, Ismail N, Nizamuddin, Majewski J (2019) Archaeological evidence that a late 14th-century tsunami devastated the coast of northern Sumatra and redirected history. Proc Natl Acad Sci USA 116(24):11679–11686. https://doi.org/10.1073/pnas.1902241116
Danielsen F, Sørensen MK, Olwig MF, Selvam V, Parish F, Burgess ND, Hiraishi T, Karunagaran VM, Rasmussen MS, Hansen LB, Quarto A, Suryadiputra N (2005) The Asian tsunami: a protective role for coastal vegetation. Science 310(5748):643. https://doi.org/10.1126/science.1118387
Delescluse M, Chamot-Rooke N, Cattin R, Fleitout L, Trubienko O, Vigny C (2012) April 2012 intra-oceanic seismicity off Sumatra boosted by the Banda-Aceh megathrust. Nature 490(7419):240–244. https://doi.org/10.1038/nature11520
Duputel Z, Kanamori H, Tsai VC, Rivera L, Meng L, Ampuero JP, Stock JM (2012) The 2012 Sumatra great earthquake sequence. Earth Planet Sci Lett 351–352:247–257. https://doi.org/10.1016/j.epsl.2012.07.017
Fahmi M, Syamsidik Fatimah E, Al’Ala M, (2017) A decade process of coastal land use changes in Peukan Bada-Aceh after the 2004 Indian Ocean Tsunami. IOP Confer Ser Earth Environ Sci. https://doi.org/10.1088/1755-1315/56/1/012012
Gahalaut VK, Catherine JK (2006) Rupture characteristics of 28 March 2005 Sumatra earthquake from GPS measurements and its implication for tsunami generation. Earth Planet Sci Lett 249(1–2):39–46. https://doi.org/10.1016/j.epsl.2006.07.015
Griffin C, Ellis D, Beavis S, Zoleta-Nantes D (2013) Coastal resources, livelihoods and the 2004 Indian Ocean tsunami in Aceh, Indonesia. Ocean Coast Manag 71:176–186. https://doi.org/10.1016/j.ocecoaman.2012.10.017
Hanks TC, Kanamori H (1979) A moment magnitude scale. J Geophys Res B: Solid Earth 84(B5):2348–2350. https://doi.org/10.1029/JB084iB05p02348
Haridhi HA, Huang BS, Wen KL, Denzema D, Prasetyo A, Lee CS (2018) A study of large earthquake sequences in the Sumatra subduction zone and its possible implications. Terr Atmos Ocean Sci 29(6):635–652. https://doi.org/10.3319/TAO.2018.08.22.01
Haridhi HA, Shouh-Huang B, Liang-Wen K, Mirza A, Rizal S, Purnawan S, Fajri I, Klingelhoefer F, Shine-Liu C, Lee S, Wilson C, Wu CR, Setiawan T-RI, Phung VB (2022) Tsunami scenario triggered by submarine landslide offshore of northern Sumatra Island and its hazard assessment. Natl Hazards Earth Syst Sci. https://doi.org/10.5194/nhess-2021-375
Horspool N, Pranantyo I, Griffin J, Latief H, Natawidjaja DH, Kongko W, Cipta A, Bustaman B, Anugrah SD, Thio HK (2014) A probabilistic tsunami hazard assessment for Indonesia. Nat Hazards Earth Syst Sci 14(11):3105–3122. https://doi.org/10.5194/nhess-14-3105-2014
Hsu YJ, Simons M, Avouac JP, Galeteka J, Sieh K, Chlieh M, Natawidjaja D, Prawirodirdjo L, Bock Y (2006) Frictional afterslip following the 2005 Nias-Simeulue earthquake. Sumatra Sci 312(5782):1921–1926. https://doi.org/10.1126/science.1126960
Imamura F, Boret SP, Suppasri A, Muhari A (2019) Recent occurrences of serious tsunami damage and the future challenges of tsunami disaster risk reduction. Prog Disaster Sci. https://doi.org/10.1016/j.pdisas.2019.100009
Ismail N, Okazaki K, Ochiai C, Fernandez G (2018) Livelihood changes in Banda Aceh, Indonesia after the 2004 Indian Ocean Tsunami. Int J Disaster Risk Reduct 28:439–449. https://doi.org/10.1016/j.ijdrr.2017.09.003
Kim DC, Kim KO, Choi BH, Kim KH, Pelinovsky E (2013) Three-dimensional runup simulation of the 2004 Indian Ocean tsunami at the Lhok Nga twin peaks. J Coastal Res 65:272–277. https://doi.org/10.2112/si65-047.1
Koshimura S, Oie T, Yanagisawa H, Imamura F (2009) Developing fragility functions for tsunami damage estimation using numerical model and post-tsunami data from banda aceh, Indonesia. Coastal Eng J 51(3):243–273. https://doi.org/10.1142/S0578563409002004
Lambotte S, Rivera L, Hinderer J (2006) Rupture length and duration of the 2004 Aceh-Sumatra earthquake from the phases of the Earth’s gravest free oscillations. Geophys Res Lett https://doi.org/10.1029/2005GL024090
Lange D, Tilmann F, Henstock T, Rietbrock A, Natawidjaja D, Kopp H (2018) Structure of the central Sumatran subduction zone revealed by local earthquake travel-time tomography using an amphibious network. Solid Earth 9(4):1035–1049. https://doi.org/10.5194/se-9-1035-2018
Latief H, Puspito NT, Imamura F (2000) Tsunami catalog and zones in Indonesia. J Nat Disaster Sci 22(1):25–43
Lavigne F, Paris R, Grancher D, Wassmer P, Brunstein D, Vautier F, Leone F, Flohic F, de Coster B, Gunawan T, Gomez C, Setiawan A, Cahyadi R, Fachrizal (2009) Reconstruction of tsunami inland propagation on December 26, 2004 in Banda Aceh, Indonesia, through field investigations. Pure Appl Geophys 166(1–2):259–281. https://doi.org/10.1007/s00024-008-0431-8
Lay T, Kanamori H, Ammon CJ, Nettles M, Ward SN, Aster RC, Beck SL, Bilek SL, Brudzinski MR, Butler R, Deshon HR, Ekström G, Satake K, Sipkin S (2005) The great Sumatra-Andaman earthquake of 26 December 2004. Science 308(5725):1127–1133. https://doi.org/10.1126/science.1112250
Li L, Switzer AD, Wang Y, Weiss R, Qiu Q, Chan CH, Tapponnier P (2015) What caused the mysterious eighteenth century tsunami that struck the southwest Taiwan coast? Geophys Res Lett 42(20):8498–8506. https://doi.org/10.1002/2015GL065567
Liu PLF, Cho YS, Yoon SB, Seo SN (1995) Numerical simulations of the 1960 Chilean Tsunami propagation and inundation at Hilo, Hawaii. In Tsuchiya Y, Shuto N (eds) Tsunami: progress in prediction, disaster prevention and warning. Springer, Netherlands, pp 99–115. https://doi.org/10.1007/978-94-015-8565-1_7
Liu PLF, Woo S-B, Cho Y-S (1998) Computer program for tsunami propagation and inundation
Mansinha L, Smylie DE (1971) The displacement fields of inclined faults. Bull Selsmol Sodety Am 61(5):1433–1440
Martin SS, Li L, Okal EA, Morin J, Tetteroo AEG, Switzer AD, Sieh KE (2019) Reassessment of the 1907 Sumatra tsunami earthquake based on macroseismic, seismological, and tsunami observations, and modeling. Pure Appl Geophys 176(7):2831–2868. https://doi.org/10.1007/s00024-019-02134-2
McCaffrey R (2009) The tectonic framework of the Sumatran subduction zone. In Ann Rev Earth Planet Sci 37:345–366. https://doi.org/10.1146/annurev.earth.031208.100212
Monecke K, Finger W, Klarer D, Kongko W, McAdoo BG, Moore AL, Sudrajat SU (2008) A 1,000-year sediment record of tsunami recurrence in northern Sumatra. Nature 455(7217):1232–1234. https://doi.org/10.1038/nature07374
Muhari A, Mück M, Diposaptono S, Spahn H (2012) Tsunami Mitigation Planning in Pacitan, Indonesia: a review of existing efforts and Ways ahead. Sci Tsunami Hazards 31(4):244
Mulia IE, Ishibe T, Satake K, Gusman AR, Murotani S (2020) Regional probabilistic tsunami hazard assessment associated with active faults along the eastern margin of the Sea of Japan. Earth Planets Space. https://doi.org/10.1186/s40623-020-01256-5
Murotani S, Iwai M, Satake K, Shevchenko G, Loskutov A (2015) Tsunami forerunner of the 2011 Tohoku earthquake observed in the sea of Japan. Pure Appl Geophys 172(3–4):683–697. https://doi.org/10.1007/s00024-014-1006-5
Newman Av, Hayes G, Wei Y, Convers J (2011) The 25 October 2010 Mentawai tsunami earthquake, from real-time discriminants, finite-fault rupture, and tsunami excitation. Geophys Res Lett. https://doi.org/10.1029/2010GL046498
Okada Y (1985) Surface deformation due to Shear and Tensile Faults in a half-space. Bull Seismol Soc Am 75(4):1135–1154
Oktari RS, Munadi K, Ridha M (2014) Effectiveness of dissemination and communication element of Tsunami early warning system in Aceh. Proc Econ Finance 18:136–142. https://doi.org/10.1016/s2212-5671(14)00923-x
Oktari RS, Syamsidik, Idroes R, Sofyan H, Munadi K (2020) City resilience towards coastal hazards: An integrated bottom-up and top-down assessment. Water. https://doi.org/10.3390/w12102823
Park J, Song TRA, Tromp J, Okal E, Stein S, Roult G, Clevede E, Laske G, Kanamori H, Davis P, Berger J, Braitenberg C, van Camp M, Lei X, Sun H, Xu H, Rosat S (2005) Earth’s free oscillations excited by the 26 December 2004 Sumatra-Andaman earthquake. Science 308(5725):1139–1144. https://doi.org/10.1126/science.1112305
Philibosian B, Sieh K, Avouac JP, Natawidjaja DH, Chiang HW, Wu CC, Shen CC, Daryono MR, Perfettini H, Suwargadi BW, Lu Y, Wang X (2017) Earthquake supercycles on the Mentawai segment of the Sunda megathrust in the seventeenth century and earlier. J Geophys Res Solid Earth 122(1):642–676. https://doi.org/10.1002/2016JB013560
Pusgen (2017) Peta Sumber dan Bahaya Gempa Indonesia Tahun 2017. Pusat Studi Gempa Nasional (Indonesia)
Rahman A, Sakurai A, Munadi K (2018) The analysis of the development of the Smong story on the 1907 and 2004 Indian Ocean tsunamis in strengthening the Simeulue island community’s resilience. Int J Disaster Risk Reduct 29:13–23. https://doi.org/10.1016/j.ijdrr.2017.07.015
Rubin CM, Horton BP, Sieh K, Pilarczyk JE, Daly P, Ismail N, Parnell AC (2017) Highly variable recurrence of tsunamis in the 7400 years before the 2004 Indian Ocean tsunami. Nat Commun. https://doi.org/10.1038/ncomms16019
Satake K (2014) Advances in earthquake and tsunami sciences and disaster risk reduction since the 2004 Indian ocean tsunami. Geosci Lett. https://doi.org/10.1186/s40562-014-0015-7
Selva J, Lorito S, Volpe M, Romano F, Tonini R, Perfetti P, Bernardi F, Taroni M, Scala A, Babeyko A, Løvholt F, Gibbons SJ, Macías J, Castro MJ, González-Vida JM, Sánchez-Linares C, Bayraktar HB, Basili R, Maesano FE (2021) Probabilistic tsunami forecasting for early warning. Nat Commun. https://doi.org/10.1038/s41467-021-25815-w
Sepúlveda I, Haase JS, Carvajal M, Xu X, Liu PLF (2020) Modeling the sources of the 2018 Palu, Indonesia, Tsunami using videos from social media. J Geophys Res Solid Earth. https://doi.org/10.1029/2019JB018675
Sieh K, Natawidjaja D (2000) Neotectonics of the Sumatran fault, Indonesia. J Geophys Res Solid Earth 105(B12):28295–28326. https://doi.org/10.1029/2000jb900120
Sieh K, Daly P, Edwards McKinnon E, Pilarczyk JE, Chiang HW, Horton B, Rubin CM, Shen CC, Ismail N, Vane CH, Feener RM (2015) Penultimate predecessors of the 2004 Indian Ocean tsunami in Aceh, Sumatra: stratigraphic, archeological, and historical evidence. J Geophys Res Solid Earth 120(1):308–325. https://doi.org/10.1002/2014JB011538
Simon T, Goldberg A, Adini B (2015) Socializing in emergencies: a review of the use of social media in emergency situations. Int J Inf Manage 35(5):609–619. https://doi.org/10.1016/j.ijinfomgt.2015.07.001
Subarya C, Chlieh M, Prawirodirdjo L, Avouac JP, Bock Y, Sieh K, Meltzner AJ, Natawidjaja DH, McCaffrey R (2006) Plate-boundary deformation associated with the great Sumatra-Andaman earthquake. Nature 440:46–51. https://doi.org/10.1038/nature04522
Syamsidik T, Suppasri A, Al’Ala M, Luthfi M, Comfort LK (2019) Assessing the tsunami mitigation effectiveness of the planned Banda Aceh outer Ring Road (BORR), Indonesia. Nat Hazards Earth Syst Sci 19(1):299–312. https://doi.org/10.5194/nhess-19-299-2019
Syamsidik, Iskandar A, Rasyif TM (2015) Progress of coastal line rehabilitation after the Indian ocean tsunami around Banda Aceh coasts. In Shaw R (ed) Recovery from the Indian ocean tsunami: a ten-year journey. Springer, Japan, pp 175–189. https://doi.org/10.1007/978-4-431-55117-1_13
Syamsidik AN, Oktari RS, Fahmi M (2019) Aceh Pasca 15 Tahun Tsunami: Kilas Balik dan Proses Pemulihan. Tsunami and Disaster Research Center (TDMRC) Universitas Syiah Kuala
Syamsidik Oktari RS, Nugroho A, Fahmi M, Suppasri A, Munadi K, Amra R (2021) Fifteen years of the 2004 Indian Ocean Tsunami in Aceh-Indonesia: mitigation, preparedness and challenges for a long-term disaster recovery process. Int J Disaster Risk Reduct. https://doi.org/10.1016/j.ijdrr.2021.102052
Synolakis CE, Bernard EN (2006) Tsunami science before and beyond Boxing Day 2004. Philos Trans R Soc A Math Phys Eng Sci 364(1845):2231–2265. https://doi.org/10.1098/rsta.2006.1824
Tabei T, Kimata F, Ito T, Gunawan E, Tsutsumi H, Ohta Y, Yamashina T, Soeda Y, Ismail N, Nurdin I, Sugiyanto D, Meilano I (2017) Geodetic and geomorphic evaluations of earthquake generation potential of the northern Sumatran fault, Indonesia. Int Assoc Geodesy Symposia 145:21–28. https://doi.org/10.1007/1345_2015_200
Tsuji Y (2006) Damage and height distribution of Sumatra Earthquake-Tsunami damage and height distribution of Sumatra Earthquake-Tsunami of in Banda Aceh City and its environs. J Disaster Res 1(1):103–115
Tursina S, Kato S, Afifuddin M (2021) Coupling sea-level rise with tsunamis: projected adverse impact of future tsunamis on Banda Aceh city Indonesia. Int J Disaster Risk Reduct. https://doi.org/10.1016/j.ijdrr.2021.102084
United Nations Office for Disaster Risk Reduction (2015) Sendai framework for disaster risk reduction 2015–2030
Vallée M (2007) Rupture properties of the giant Sumatra earthquake imaged by empirical green’s function analysis. Bull Seismol Soc Am. https://doi.org/10.1785/0120050616
Wang X (2009) User manual for COMCOT Version 1.7 (first draft)
Wang X, Liu PLF (2006) An analysis of 2004 Sumatra earthquake fault plane mechanisms and Indian Ocean tsunami. J Hydraul Res 44(2):147–154. https://doi.org/10.1080/00221686.2006.9521671
Wang D, Mori J, Uchide T (2012) Supershear rupture on multiple faults for the mw 8.6 off Northern Sumatra, Indonesia earthquake of April 11, 2012. Geophys Res Lett. https://doi.org/10.1029/2012GL053622
Wassmer P, Gomez C, Iskandasyah TYWM, Lavigne F, Sartohadi J (2015) Contribution of anisotropy of magnetic susceptibility (AMS) to reconstruct flooding characteristics of a 4220 BP tsunami from a thick unconsolidated structureless deposit (Banda Aceh, Sumatra). Front Earth Sci. https://doi.org/10.3389/feart.2015.00040
Book Google Scholar
Weller O, Lange D, Tilmann F, Natawidjaja D, Rietbrock A, Collings R, Gregory L (2012) The structure of the Sumatran Fault revealed by local seismicity. Geophys Res Lett. https://doi.org/10.1029/2011GL050440
Wells DL, Coppersmith KJ (1994) New empirical Relationships among Magnitude, rupture length, rupture width, rupture area, and Surface Displacement. Bull Seismol Soc Am 84(4):974–1002
Witvorapong N, Muttarak R, Pothisiri W (2015) Social participation and disaster risk reduction behaviors in tsunami prone areas. PLoS ONE. https://doi.org/10.1371/journal.pone.0130862
Yue H, Lay T, Koper KD (2012) En échelon and orthogonal fault ruptures of the 11 April 2012 great intraplate earthquakes. Nature 490(7419):245–249. https://doi.org/10.1038/nature11492
Download references
Acknowledgements
The authors would like to express gratitude to The Ministry of Education, Culture, Research, and Technology (Kemendikbudristek) Republic of Indonesia, Directorate General of Higher Education, Research, and Technology and the Directorate of Research and Community Engagement of Syiah Kuala University for their financial support through the National Competitive Research Grant under the Basic Research scheme (SBK Riset Dasar), based on the Decree Number 25/UN11.2.1/PT.01.03/DPRM/2021 and Agreement/Contract Number 154/SP2H/LT/DPRM/2021, with the title “ Mitigasi Tsunami Berbasis Soft-Structure dengan Tinjauan Aspek Lingkungan dan Sosial .“ This publication is a part of the research output funded by this grant. The authors would also like to extend sincere appreciation to all individuals and organizations who have contributed, directly or indirectly, to the successful completion of this research.
Author information
Authors and affiliations.
Civil and Environmental Engineering Department, Faculty of Engineering, Universitas Gadjah Mada, Jl. Grafika, Kampus No.2, Yogyakarta, Indonesia
Tsunami and Disaster Mitigation Research Center (TDMRC), Universitas Syiah Kuala, Jl. Hamzah Fansuri No.8, Darussalam, Banda Aceh, 23111, Indonesia
Syamsidik, Yunita Idris & Nadri Pratama Putra
Civil Engineering Department, Faculty of Engineering, Universitas Syiah Kuala, Jl. Syeh Abdurrauf No.7, Darussalam, Banda Aceh, 23111, Indonesia
Syamsidik & Yunita Idris
You can also search for this author in PubMed Google Scholar
Contributions
B: conceptualization, simulation, data analysis, visualization, writing – original draft, and editing the manuscript. S: reviewing, revising, and improving the quality of the manuscript. YI: reviewing and revising the manuscript. NP: field survey for topography data.
Corresponding author
Correspondence to Benazir .
Ethics declarations
Competing interests.
The authors declare no competing interests.
Additional information
Publisher’s note.
Springer Nature remains neutral with regard to jurisdictional claims in published maps and institutional affiliations.
Rights and permissions
Open Access This article is licensed under a Creative Commons Attribution 4.0 International License, which permits use, sharing, adaptation, distribution and reproduction in any medium or format, as long as you give appropriate credit to the original author(s) and the source, provide a link to the Creative Commons licence, and indicate if changes were made. The images or other third party material in this article are included in the article's Creative Commons licence, unless indicated otherwise in a credit line to the material. If material is not included in the article's Creative Commons licence and your intended use is not permitted by statutory regulation or exceeds the permitted use, you will need to obtain permission directly from the copyright holder. To view a copy of this licence, visit http://creativecommons.org/licenses/by/4.0/ .
Reprints and permissions
About this article
Cite this article.
Benazir, Syamsidik, Idris, Y. et al. Connecting community’s perspectives on tsunami risk to anticipated future tsunamis: a reflection from a progress of tsunami preparedness from a coastal community in Aceh-Indonesia after 19 years of the 2004 Indian Ocean Tsunami. Geoenviron Disasters 10 , 21 (2023). https://doi.org/10.1186/s40677-023-00252-7
Download citation
Received : 28 July 2023
Accepted : 30 September 2023
Published : 11 October 2023
DOI : https://doi.org/10.1186/s40677-023-00252-7
Share this article
Anyone you share the following link with will be able to read this content:
Sorry, a shareable link is not currently available for this article.
Provided by the Springer Nature SharedIt content-sharing initiative
- Tsunami modelling
- Tsunami preparedness
- Community perspectives
- Indian Ocean Tsunami
- Disaster management
- 0 Shopping Cart
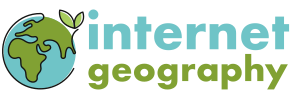
Case Study – The 2011 Japan Earthquake
Cambridge iGCSE Geography > The Natural Environment > Earthquakes and Volcanoes > Case Study – The 2011 Japan Earthquake
Background Information
Location : The earthquake struck 250 miles off the northeastern coast of Japan’s Honshu Island at 2:46 pm (local time) on March 11, 2011.
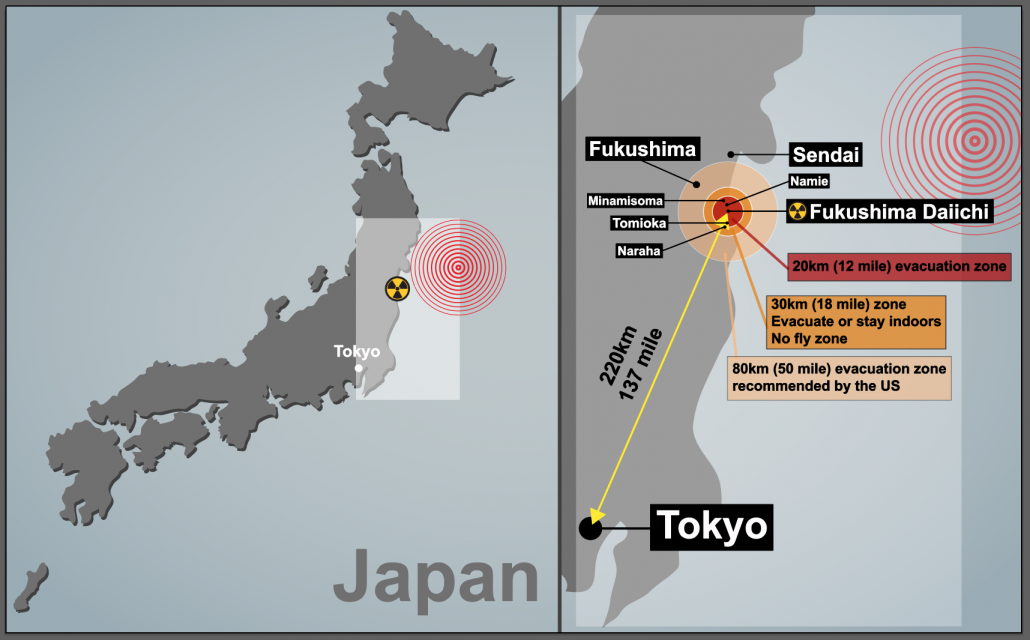
Japan 2011 Earthquake map
Magnitude : It measured 9.1 on the Moment Magnitude scale, making it one of the most powerful earthquakes ever recorded.
Japan is a highly developed country with advanced infrastructure, technology, and a robust economy. The nation has a high GDP, an efficient healthcare system, and extensive education. However, it’s also located in the Pacific Ring of Fire, making it prone to earthquakes.
What caused the 2011 Japan earthquake?
Japan is located on the eastern edge of the Eurasian Plate. The Eurasian plate, which is continental, is subducted by the Pacific Plate, an oceanic plate forming a subduction zone to the east of Japan. This type of plate margin is known as a destructive plate margin . The process of subduction is not smooth. Friction causes the Pacific Plate to stick. Pressure builds and is released as an earthquake.
Friction has built up over time, and when released, this caused a massive ‘megathrust’ earthquake. The enormous tension released as the plates shifted caused the seafloor to uplift, triggering the earthquake and subsequent tsunami .
The amount of energy released in this single earthquake was 600 million times the energy of the Hiroshima nuclear bomb.
Scientists drilled into the subduction zone soon after the earthquake and discovered a thin, slippery clay layer lining the fault. The researchers think this clay layer allowed the two plates to slide an incredible distance, some 164 feet (50 metres), facilitating the enormous earthquake and tsunami.
The earthquake occurred at a relatively shallow depth of 20 miles below the surface of the Pacific Ocean. This, combined with the high magnitude, caused a tsunami (find out more about how a tsunami is formed on the BBC website).
What were the primary effects of the 2011 Japan earthquake?
- Ground Shaking : Extensive damage to buildings and infrastructure.
- Landfall: Some coastal areas experienced land subsidence as the earthquake dropped the beachfront in some places by more than 50 cm.
What were the secondary effects of the 2011 Japan earthquake?
- Tsunami : A giant tsunami wave resulted in widespread destruction along the coast.
- Fatalities : Around 16,000 deaths were reported, mainly resulting from the tsunami.
- Injuries : 26,152 were injured, mainly as a result of the tsunami.
- Nuclear Crisis : The Fukushima Daiichi nuclear power plant was damaged, leading to radiation leaks.
- Economic Loss : Estimated at over $235 billion.
- Displacement : Around 340,000 people were displaced from their homes.
- Damage: The tsunami destroyed or damaged 332,395 buildings, 2,126 roads, 56 bridges, and 26 railways. Three hundred hospitals were damaged, and 11 were destroyed.
- Environmental Damage : Coastal ecosystems were heavily impacted.
- Blackouts: Over 4.4 million households were left without electricity in North-East Japan.
- Transport: Rural areas remained isolated for a long time because the tsunami destroyed major roads and local trains and buses. Sections of the Tohoku Expressway were damaged. Railway lines were damaged, and some trains were derailed.
What were the immediate responses to the 2011 Japan earthquake?
Tsunami Warnings and Prediction :
- The Japan Meteorological Agency issued tsunami warnings three minutes after the earthquake.
- Scientists predicted where the tsunami would hit using modelling and forecasting technology.
Search and Rescue Operations:
- Rescue workers and 100,000 members of the Japan Self-Defence Force were dispatched within hours.
- Some individuals were rescued from beneath rubble with the aid of sniffer dogs.
Radiation Protection Measures:
- The government declared a 20 km evacuation zone around the Fukushima nuclear power plant.
- Evacuees from the area around the nuclear power plant were given iodine tablets to reduce radiation poisoning risk.
International Assistance:
- Japan received help from the US military.
- Search and rescue teams from New Zealand, India, South Korea, China, and Australia were sent.
Access and Evacuation :
- Access was restricted to affected areas due to debris and mud, complicating immediate support.
- Hundreds of thousands were evacuated to temporary shelters or relocated.
Health Monitoring :
- Those near the Fukushima Daiichi nuclear meltdown had radiation levels checked and their health monitored.
- Measures were taken to ensure individuals did not receive dangerous exposure to radiation.
What were the long-term responses to the 2011 Japan earthquake?
Reconstruction Policy and Budget:
- Establishment of the Reconstruction Policy Council in April 2011.
- Approval of a budget of 23 trillion yen (£190 billion) for recovery over ten years.
- Creation of ‘Special Zones for Reconstruction’ to attract investment in the Tohoku region.
Coastal Protection Measures:
- Implementing coastal protection policies like seawalls and breakwaters designed for a 150-year recurrence interval of tsunamis.
Legislation for Tsunami-Resilient Communities:
- Enactment of the ‘Act on the Development of Tsunami-resilient Communities’ in December 2011.
- Emphasis on human life, combining infrastructure development with measures for the largest class tsunami.
Economic Challenges and Recovery:
- Japan’s economy wiped 5–10% off the value of stock markets post-earthquake.
- Long-term response priority: rebuild infrastructure, restore and improve the economy’s health.
Transportation and Infrastructure Repair:
- Repair and reopening of 375 km of the Tohoku Expressway by the 24th of March 2011.
- Restoration of the runway at Sendai Airport by the 29th of March, a joint effort by the Japanese Defence Force and the US Army.
Utility Reconstruction:
- Energy, water supply, and telecommunications infrastructure reconstruction.
- As of November 2011: 96% of electricity, 98% of water, and 99% of the landline network had been restored.
How does Japan prepare for earthquakes, and what was its impact?
Japan has a comprehensive earthquake preparedness program, including:
- Strict Building Codes : Buildings are constructed to withstand seismic activity.
- Early Warning Systems : Advanced technology provides early warnings to citizens.
- Education and Drills : Regular earthquake drills in schools, offices, and public places.
Impact of the 2011 Earthquake
The extensive preparation in Japan likely saved lives and reduced damage during the 2011 earthquake. However, the unprecedented magnitude of the event still led to significant destruction, particularly with the tsunami and nuclear crisis.
The 2011 Japan earthquake illustrates the complexity of managing natural disasters in even the most developed and prepared nations. The event prompted further refinements in disaster preparedness and response in Japan and globally, highlighting the need for continuous assessment and adaptation to seismic risks.
The 2011 earthquake occurred off Japan’s Honshu Island, measuring 9.1 on the Moment Magnitude scale, one of the strongest ever recorded.
Triggered by a ‘megathrust’ in a destructive plate margin, the Pacific Plate subducted the Eurasian Plate, releasing energy equivalent to 600 million Hiroshima bombs.
Primary effects included extensive ground shaking and significant land subsidence in coastal areas.
Secondary effects included a massive tsunami, around 16,000 deaths, 26,152 injuries, a nuclear crisis at Fukushima, over $235 billion in economic loss, displacement of 340,000 people, and widespread damage to infrastructure and the environment.
Immediate responses included rapid tsunami warnings, extensive search and rescue operations, radiation protection measures, international assistance, and evacuation strategies.
Long-term responses focused on reconstruction policies, coastal protection, tsunami-resilient community development, economic recovery, and transportation and utility restoration.
Japan’s extensive earthquake preparedness, including strict building codes and early warning systems, likely reduced damage, but the magnitude still caused significant destruction.
Check Your Knowledge
Coming soon
Test Yourself
The natural environment, share this:.
- Click to share on Twitter (Opens in new window)
- Click to share on Facebook (Opens in new window)
- Click to share on Pinterest (Opens in new window)
- Click to email a link to a friend (Opens in new window)
- Click to share on WhatsApp (Opens in new window)
- Click to print (Opens in new window)
Please Support Internet Geography
If you've found the resources on this site useful please consider making a secure donation via PayPal to support the development of the site. The site is self-funded and your support is really appreciated.
Search Internet Geography
Top posts and pages.

Latest Blog Entries

Pin It on Pinterest
- Click to share
- Print Friendly
Increasing resilience to catastrophic near-field tsunamis: systems for capturing, modelling, and assessing vertical evacuation practices
- Original Paper
- Published: 10 January 2023
Cite this article
- Jorge León ORCID: orcid.org/0000-0001-9261-6248 1 ,
- Alonso Ogueda 2 ,
- Alejandra Gubler 3 ,
- Patricio Catalán 4 ,
- Matías Correa 1 ,
- Javiera Castañeda 3 &
- Gianni Beninati 5
448 Accesses
Explore all metrics
Tsunami vertical evacuation (TVE) buildings have the potential to save many human lives in countries exposed to near-field tsunamis. Up to now, TVE research has examined three main topics separately: shelter siting, building benchmarks, and decision-making by evacuees. This study aims to integrate these topics to develop more comprehensive TVE planning frameworks. To this, we examined a catastrophic tsunami evacuation scenario in Viña del Mar, Chile. First, we developed an agent-based model to estimate potential human fatalities in the case of a fully horizontal evacuation. Second, we designed an immersive VR experience, which we applied to a sample of 151 people in the city to collect their potential TVE decision-making, which allowed us to identify 11 buildings that could serve as TVE shelters. Lastly, we incorporated this new evacuation system into the former agent-based model to assess the potential impact of vertical evacuation. Our findings showed that while fatalities ranged from 50 to 72% of the population in the fully horizontal scenario, the inclusion of TVE buildings might decrease human losses by 6.5–13.7%. Complementary questionnaires administered to participants highlighted their lack of previous experience in real-world evacuations (only 45.69% had previously experienced a tsunami-related evacuation process), as well as their knowledge about how to proceed in the case of a future emergency (62.91% declared that they knew where to go during an evacuation).
This is a preview of subscription content, log in via an institution to check access.
Access this article
Price includes VAT (Russian Federation)
Instant access to the full article PDF.
Rent this article via DeepDyve
Institutional subscriptions
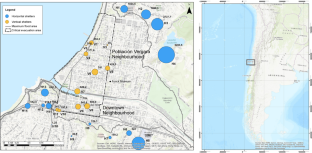
Similar content being viewed by others
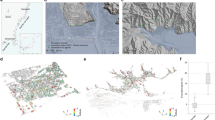
Urban structure reinforces attitudes towards tsunami evacuation
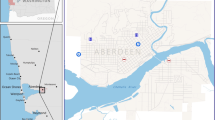
Examining the Impact of Risk Perception on the Accuracy of Anisotropic, Least-Cost Path Distance Approaches for Estimating the Evacuation Potential for Near-Field Tsunamis
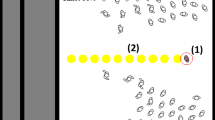
Exploring Determinants of Pre-movement Delays in a Virtual Crowd Evacuation Experiment
Aguirre BE, El-Tawil S, Best E et al (2011) Contributions of social science to agent-based models of building evacuation. Contemp Soc Sci 6:415–432. https://doi.org/10.1080/21582041.2011.609380
Article Google Scholar
Álvarez G, Quiroz M, León J, Cienfuegos R (2018) Identification and classification of urban micro-vulnerabilities in tsunami evacuation routes for the city of Iquique, Chile. Nat Hazards Earth Syst Sci. https://doi.org/10.5194/nhess-18-2027-2018
Ashar F, Amaratunga D, Haigh R (2018a) Tsunami evacuation routes using network analysis: a case study in Padang. In: Procedia engineering. Elsevier Ltd, pp 109–116
Ashar F, Amaratunga D, Sridarran P, Haigh R (2018b) Practices of tsunami evacuation planning in Padang, Indonesia. In: Krishnamurthy RR, Jonathan MP, Srinivasalu S, Glaeser B (eds) Coastal management: global challenges and innovations. Elsevier, Amsterdam, pp 399–433
Google Scholar
Buchmüller S, Weidmann U (2006) Parameters of pedestrians, pedestrian traffic and walking facilities. Zürich
Cabinet Office Government of Japan (2005) Guideline for tsunami evacuation buildings
Carvajal M, Araya-Cornejo C, Sepulveda I et al (2019) Nearly Instantaneous Tsunamis Following the Mw 7.5 2018 Palu Earthquake. Geophys Res Lett 46:5117–5126. https://doi.org/10.1029/2019GL082578
Carvajal M, Cisternas M, Catalán PA (2017) Source of the 1730 Chilean earthquake from historical records: Implications for the future tsunami hazard on the coast of Metropolitan Chile. J Geophys Res Solid Earth 122:3648–3660. https://doi.org/10.1002/2017JB014063
Dall’Osso F, Dominey-Howes D (2010) Public assessment of the usefulness of “draft” tsunami evacuation maps from Sydney Australia – implications for the establishment of formal evacuation plans. Nat Hazards Earth Syst Sci 10:1739–1750
Dewi RS (2012) A-Gis based approach of an evacuation model for tsunami risk reduction. J Integr Disaster Risk Manag 2:108–139
Ding N, Chen T, Zhu Y, Lu Y (2021) State-of-the-art high-rise building emergency evacuation behavior. Phys A Stat Mech Its Appl 561:125168. https://doi.org/10.1016/J.PHYSA.2020.125168
Engstfeld A, Killebrew K, Scott C, Wiser J (2010) Tsunami safe haven project. Report for Long Beach, WA, Seattle, Washington
Fraser S (2014) Informing the development of tsunami vertical evacuation strategies in New Zealand. Massey University, Wellington
Fraser S, Leonard GS, Matsuo I, Murakami H (2012) Tsunami evacuation: lessons from the Great East Japan earthquake and tsunami of March 11th 2011. GNS Science, Avalon
Goda K, Dasiewicz I, Li S, et al (2013) Tsunami casualty mitigation through vertical evacuation buildings: a case study from the March 11th 2011 Tohoku earthquake. In: Forensic engineering: informing the future with lessons from the past - proceedings of the 5th international conference on forensic engineering. Thomas Telford Services Ltd, pp 137–147
González M (2013) “Evacuación vertical” en Chile: una alternativa posible para evitar víctimas fatales en caso de tsunami. In: 01-07-2013. http://ciperchile.cl/2013/07/01/“evacuacion-vertical”-en-chile-una-alternativa-posible-para-evitar-victimas-fatales-en-caso-de-tsunami/ . Accessed 12 Mar 2018
Goto Y, Affan M, Agussabti, et al (2012) Tsunami evacuation simulation for disaster education and city planning. J Disaster Res 7:92–101
Haiqal M, Sari LH, Evalina Z, Hasibuan P (2019) A review of vertical evacuation on tsunami mitigation case. IOP Conf Ser Mater Sci Eng 523:1–8. https://doi.org/10.1088/1757-899X/523/1/012061
Hall S, Pettersson J, Meservy W et al (2017) Awareness of tsunami natural warning signs and intended evacuation behaviors in Java, Indonesia. Nat Hazards 89:473–496. https://doi.org/10.1007/S11069-017-2975-3/TABLES/1
Hassan W, Kakoty P, Ortega M, Simbson B (2018) Efficiency assessment of tsunami evacuation routes in Viña del mar, Chile. In: Eleventh U.S. national conference on earthquake engineering. Los Angeles, CA
Heintz JA, Rojahn C, Coulbourne WL, et al (2019) Guidelines for Design of Structures for Vertical Evacuation from Tsunamis (Third edition). FEMA P-646. Redwood City, California
INE (2018) Censo 2017
Izquierdo T, Fritis E, Abad M (2018) Analysis and validation of the PTVA tsunami building vulnerability model using the 2015 Chile post-tsunami damage data in Coquimbo and la Serena cities. Nat Hazards Earth Syst Sci 18:1703–1716. https://doi.org/10.5194/NHESS-18-1703-2018
Kim K, Kaviari F, Pant P, Yamashita E (2022) An agent-based model of short-notice tsunami evacuation in Waikiki. Hawaii Transp Res Part D Transp Environ 105:103239. https://doi.org/10.1016/J.TRD.2022.103239
Kubisch S, Guth J, Keller S et al (2020) The contribution of tsunami evacuation analysis to evacuation planning in Chile: applying a multi-perspective research design. Int J Disaster Risk Reduct 45:101462. https://doi.org/10.1016/j.ijdrr.2019.101462
León J, Castro S, Mokrani C, Gubler A (2020) Tsunami evacuation analysis in the urban built environment: a multi-scale perspective through two modeling approaches in Viña del Mar, Chile. Coast Eng J. https://doi.org/10.1080/21664250.2020.1738073
León J, Catalán PA, Gubler A (2021) Assessment of top-down design of tsunami evacuation strategies based on drill and modelled data. Front Earth Sci 9:1. https://doi.org/10.3389/FEART.2021.744193
León J, Mokrani C, Catalán P et al (2019a) The role of built environment’s physical urban form in supporting rapid tsunami evacuations: using computer-based models and real-world data as examination tools. Front Built Environ. https://doi.org/10.3389/fbuil.2018.00089
León J, Vicuña M, Gubler A (2019b) Increasing tsunami risk through intensive urban densification in metropolitan areas: a longitudinal analysis in Viña del Mar. Chile Int J Disaster Risk Reduct. https://doi.org/10.1016/j.ijdrr.2019.101312
León J, Zamora N, Castro S et al (2019c) Evacuación vertical como medida de mitigación del riesgo de tsunamis en Chile
Lindell MK, Prater CS, Gregg CE et al (2015) Households’ immediate responses to the 2009 American Samoa earthquake and tsunami. Int J Disaster Risk Reduct 12:328–340. https://doi.org/10.1016/J.IJDRR.2015.03.003
Mas E, Adriano B, Koshimura S (2013) An Integrated simulation of tsunami hazard and human evacuation in La Punta, Peru. J Disaster Res 8:285–295
McCaughey JW, Mundir I, Daly P et al (2017) Trust and distrust of tsunami vertical evacuation buildings: Extending protection motivation theory to examine choices under social influence. Int J Disaster Risk Reduct 24:462–473
MINVU (2013) Diseño estructural para edificaciones en áreas de riesgo de inundación por tsunami o seiche - NTM 007. Santiago
Mostafizi A, Wang H, Cox D et al (2017) Agent-based tsunami evacuation modeling of unplanned network disruptions for evidence-driven resource allocation and retrofitting strategies. Nat Hazards 88:1347–1372. https://doi.org/10.1007/s11069-017-2927-y
Mostafizi A, Wang H, Cox D, Dong S (2019) An agent-based vertical evacuation model for a near-field tsunami: Choice behavior, logical shelter locations, and life safety. Int J Disaster Risk Reduct 34:467–479
Mück M (2008) Tsunami Evacuation Modelling. Development and application of a spatial information system supporting tsunami evacuation planning in South-West Bali. Universität Regensburg
Obando J, Ugalde D, López-García D (2015) HOUSING – Illapel Earthquake. Oakland, CA
ONEMI (2014) Recomendaciones para la preparación y respuesta ante tsunamis. ONEMI, Santiago, Chile
Padilla Almeida O, Matheus A, Cruz M et al (2016) Enhanced vertical evacuation application with geomatic tools for tsunamis in Salinas. Ecuador J Tsunami Soc Int 35:106
Park S, van de Lindt JW, Gupta R, Cox D (2012) Method to determine the locations of tsunami vertical evacuation shelters. Nat Hazards 63:891–908. https://doi.org/10.1007/s11069-012-0196-3
Poulos A, Tocornal F, de la Llera JC, Mitrani-Reiser J (2018) Validation of an agent-based building evacuation model with a school drill. Transp Res Part C Emerg Technol 97:82–95. https://doi.org/10.1016/j.trc.2018.10.010
Raskin J, Wang Y (2017) Fifty-year resilience strategies for coastal communities at risk for tsunamis. Nat Hazards Rev 18:B4016003. https://doi.org/10.1061/(ASCE)NH.1527-6996.0000220
Raskin J, Wang Y, Boyer M et al (2011) An evacuation building project for Cascadia earthquakes and tsunamis. Obras y Proy 9:11–22
SECTRA (2016) Encuesta de Origen-Destino de Viajes Gran Valparaíso. Ministerio de Obras Públicas, Transportes y Telecomunicaciones, Santiago, Chile
Shibayama T, Esteban M, Nistor I et al (2013) Classification of tsunami and evacuation areas. Nat Hazards 67:365–386. https://doi.org/10.1007/s11069-013-0567-4
SHOA (2012) Proyecto CITSU. http://www.shoa.cl/index.htm
Shuto N (2005) Tsunamis: their coastal effects and defense works. In: Tingsanchali T (ed) Scientific forum on the tsunami, its impact and recovery. Asian Institute of Technology, Bangkok, Thailand, pp 1–12
Sugimoto T, Murakami H, Kozuki Y, Nishikawa K (2003) A human damage prediction method for tsunami disasters incorporating evacuation activities. Nat Hazards 585–600
Sun Y, Sun J (2019) Perception, preparedness, and response to tsunami risks in an aging society: Evidence from Japan. Saf Sci 118:466–474. https://doi.org/10.1016/j.ssci.2019.05.060
Suppasri A, Shuto N, Imamura F et al (2012) Lessons learned from the 2011 Great East Japan tsunami: performance of tsunami countermeasures, coastal buildings, and tsunami evacuation in Japan. Pure Appl Geophys 170:993–1018
Suppasri A, Shuto N, Imamura F et al (2013) Lessons learned from the 2011 Great East Japan Tsunami: performance of tsunami countermeasures, coastal buildings, and tsunami evacuation in Japan. Pure Appl Geophys 170:993–1018. https://doi.org/10.1007/s00024-012-0511-7
Velotti L, Trainor JE, Engel K et al (2013) Beyond vertical evacuation: research considerations for a comprehensive “vertical protection strategy.” Int J Mass Emerg Disasters 31:60–77
Voulgaris G, Aleksejeva J (2017) Spatiotemporal identification of potential tsunami vertical evacuation sites: a case study of Shizuoka City. Japan Plos Curr. https://doi.org/10.1371/currents.dis.e66442ce2b19de55532457d967d9645d
Wang H, Mostafizi A, Cramer LA et al (2016) An agent-based model of a multimodal near-field tsunami evacuation: Decision-making and life safety. Transp Res Part C Emerg Technol 64:86–100. https://doi.org/10.1016/J.TRC.2015.11.010
Wang Z, Jia G (2020) A novel agent-based model for tsunami evacuation simulation and risk assessment. Nat Hazards 105:2045–2071. https://doi.org/10.1007/S11069-020-04389-8
Wang Z, Jia G (2021) A novel agent-based model for tsunami evacuation simulation and risk assessment. Nat Hazards 105:2045–2071. https://doi.org/10.1007/S11069-020-04389-8/FIGURES/1
Wood N, Jones J, Schelling J, Schmidtlein M (2014) Tsunami vertical-evacuation planning in the US Pacific Northwest as a geospatial, multi-criteria decision problem. Int J Disaster Risk Reduct 9:68–83
Yagi S, Hasemi Y (2010) Requirements and verification methodology for the design performance of Tsunami-Hinan buildings (temporary tsunami refuge building). J Disaster Res 5:591–600
Yeh HH, Robertson I, Preuss J (2005) Development of design guidelines for structures that serve as tsunami vertical evacuation sites. Washington State Department of Natural Resources, Division of Geology and Earth Resources
Yuzal H, Kim K, Pant P, Yamashita E (2017) Tsunami evacuation buildings and evacuation planning in Banda Aceh, Indonesia. J Emerg Manag 15:49–61. https://doi.org/10.5055/jem.2017.0312
Zamora N, Catalán PA, Gubler A, Carvajal M (2021) Microzoning tsunami hazard by combining flow depths and arrival times. Front Earth Sci 8:747. https://doi.org/10.3389/feart.2020.591514
Download references
Acknowledgements
Powered@NLHPC: This research was partially supported by the supercomputing infrastructure of the NLHPC (ECM-02). We also would like to thank Matías Carvajal for providing the fault model of the 1730 earthquake.
JL, PC, and AG were funded by the Research Center for Integrated Disaster Risk Management (CIGIDEN), ANID/ FONDAP/15110017. JL was also funded by the research Grant ANID/FONDECYT/1210184. PC and AG were also funded by ANID, Chile, through its grant FONDEF ID19I10048. PC was also funded by the Centro Científico Tecnológico de Valparaíso, ANID PIA/APOYO AFB180002.
Author information
Authors and affiliations.
Departamento de Arquitectura, Universidad Técnica Federico Santa María, Valparaíso, Chile
Jorge León & Matías Correa
Department of Mathematical Sciences, George Mason University, Fairfax, VA, USA
Alonso Ogueda
Research Center for Integrated Disaster Risk Management (CIGIDEN), Santiago, Chile
Alejandra Gubler & Javiera Castañeda
Departamento de Obras Civiles, Universidad Técnica Federico Santa María, Valparaíso, Chile
Patricio Catalán
Departamento de Informática, Universidad Técnica Federico Santa María, Valparaíso, Chile
Gianni Beninati
You can also search for this author in PubMed Google Scholar
Contributions
This study was conceived and developed by JL and PC. AO carried out the agent-based model and the statistical analysis of its results. AG worked out the tsunami flood model. MC and GB prepared and applied the VR immersive experience, with the help of JC. JL prepared the first version of the manuscript with reviews by PC.
Corresponding author
Correspondence to Jorge León .
Ethics declarations
Conflict of interest.
The authors have no relevant financial or non-financial interests to disclose.
Additional information
Publisher's note.
Springer Nature remains neutral with regard to jurisdictional claims in published maps and institutional affiliations.
Rights and permissions
Springer Nature or its licensor (e.g. a society or other partner) holds exclusive rights to this article under a publishing agreement with the author(s) or other rightsholder(s); author self-archiving of the accepted manuscript version of this article is solely governed by the terms of such publishing agreement and applicable law.
Reprints and permissions
About this article
León, J., Ogueda, A., Gubler, A. et al. Increasing resilience to catastrophic near-field tsunamis: systems for capturing, modelling, and assessing vertical evacuation practices. Nat Hazards (2023). https://doi.org/10.1007/s11069-022-05732-x
Download citation
Received : 08 July 2022
Accepted : 23 November 2022
Published : 10 January 2023
DOI : https://doi.org/10.1007/s11069-022-05732-x
Share this article
Anyone you share the following link with will be able to read this content:
Sorry, a shareable link is not currently available for this article.
Provided by the Springer Nature SharedIt content-sharing initiative
- Vertical evacuation
- Agent-based modelling
- Virtual reality
Advertisement
- Find a journal
- Publish with us
- Track your research
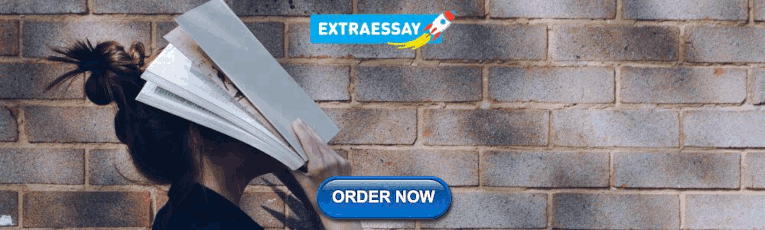
IMAGES
VIDEO
COMMENTS
Tsunami Disasters: Case Studies and Reports. <p>This Collection is part of the 'Tsunami Disaster Channel' containing a number of case studies and reports relevant to tsunami disasters, where we try to find out what we have learnt from the past and how we can best reduce risk in future natural disasters. Current guidance comes from leading ...
The TUNAMI model has been extensively benchmarked and used in several tsunami case studies 24,28,29,30. The accuracy of the model is validated by using the observed waveform at the tide gauge ...
Overview. On Friday 28th September 2018 a magnitude 7.5 earthquake struck Palu, on the Indonesian island of Sulawesi, just before dusk wreaking havoc and destruction across the city and triggering a deadly tsunami on its coast. The 7.5 magnitude earthquake hit only six miles from the country's coast. A map to show the location of Palu.
The topography of the seabed has a vital role to play in deciding the damage, a tsunami can cause. Usually, it's not the first waves of the tsunami that are damaging, but the subsequent second, third and fourth waves do most of the damage (Indonesian Tsunami information centre, 2018).Most of the tsunami occurrences (>80%) are centred along the Pacific Ring of Fire, a highly tectonically active ...
Tsunami research accelerated in response to devastating tsunamis that occurred in 1992 in Nicaragua and Indonesia. In the following three decades, additional tsunamis occurred that challenged the conventional assumptions for tsunami wave generation, propagation, and inundation (Kânoğlu et al., 2020; Okal, 2019).The preponderance of shallow subduction zone faulting and generation of tsunamis ...
The scale and tragedy of the giant tsunamis in 2004, 2010 and 2011 led to a revolution in tsunami monitoring. This Review assesses the advances in tsunami observation, monitoring and hazard ...
This paper summarizes the essential publications that have published tsunami-related documents for researchers. A list of the most active authors, top-cited documents, and most indexed keywords is also available. ... Mizutani N (2019) Machine learning algorithms for real-time tsunami inundation forecasting: a case study in Nankai region. Pure ...
Tsunami, or harbor (tsun-) wave (-ami) in Japanese, are caused by geological processes, such as earthquakes, landslides or volcanic eruptions, which displace large volumes of ocean water.The displaced sea surface propagates outward from the source as a series of ocean waves with extremely long wavelengths and periods. Wind-generated waves cause water motion to depths of 150 m; a tsunami ...
In this study, we presented a tsunami inundation forecasting approach based on a CNN trained with a large quantity of synthetic tsunami scenarios and verified the approach against both synthetic ...
Twenty-three papers are included in this PAGEOPH topical issue "Sixty Years of Modern Tsunami Science, Volume I: Lessons and Progress". The papers are grouped into four categories: historical tsunami events, studies on tsunami source models and case studies, tsunami hydrodynamics, and probabilistic tsunami hazard assessment and forecasting. Papers that reflect the current state of tsunami ...
Field study of the effects of the December 2004 and March 2005 earthquakes and tsunamis - April 2005. December 26, 2004, Sumatra-Andaman Islands. Tsunami generation from the 2004 M=9.1 Sumatra-Andaman earthquake. Initial findings on tsunami sand deposits, damage, and inundation in Sumatra - January 2005.
111 Chapter j 4 Tsunami Case Studies It is estimated that on e-third of Hilo was destroyed by thre e large waves. Approximat ely 500 buildin gs were destroyed, 1,000 bui ldings were se verely
The tsunamis we hear about most often are caused by undersea earthquakes, and the waves they generate can travel at speeds of up to 250 miles per hour and reach tens of meters high when they make landfall and break. They can cause massive flooding and rapid widespread devastation in coastal areas, as happened in Southeast Asia in 2004 and in ...
The tsunami and its aftermath were responsible for immense destruction and loss on the rim of the Indian Ocean. On December 26, 2004, at 7:59 am local time, an undersea earthquake with a magnitude of 9.1 struck off the coast of the Indonesian island of Sumatra. Over the next seven hours, a tsunami —a series of immense ocean waves—triggered ...
In this paper, we review three post-tsunami disaster case studies: the Indian Ocean tsunami (IOT) on 26 December 2004, the Java tsunami on 17 July 2006 and the South Pacific tsunami on 29 September 2009. The 2004 IOT and 2006 Java tsunami surveys involved delayed-response post-disaster research using video interviewing. ... Related Research ...
3. Case Studies and Observations Case studies and the observations therein are an important part of tsunami research that highlight the hazard for specific areas, including important recent and historic events. The largest recorded tsunami along the Caribbean coast of Central America occurred on 22 April, 1991 when an earthquake with magnitude M
The word 'tsunami' brings immediately to mind the havoc that can be wrought by these uniquely powerful waves. The tsunamis we hear about most often are caused by undersea earthquakes, and the ...
As a case study, we examine two M w 7.8 ... Three-dimensional numerical modeling of tsunami-related internal gravity waves in the Hawaiian atmosphere. Earth Planets Space 63, 847-851 (2011).
The characteristics of the tsunami that struck the study area were mega hazards, with recorded tsunami heights of 20 m and 13.7 m on the hill slopes of Gampong Lambaro Neujid (Lavigne et al. 2009). The recorded wave heights were influenced by the proximity of the tsunami source to the Aceh coast, categorizing it as a near-field tsunami.
Location: The earthquake struck 250 miles off the northeastern coast of Japan's Honshu Island at 2:46 pm (local time) on March 11, 2011. Japan 2011 Earthquake map. Magnitude: It measured 9.1 on the Moment Magnitude scale, making it one of the most powerful earthquakes ever recorded. Japan is a highly developed country with advanced ...
Tsunami detection and investigation of its early warning is the very important issue nowadays, which supports our existing system more precise. • This paper proposes a case study of the mathematical models of the ocean wave imaging schemes and the Tsunami detection system model for the Japan's region where Tsunamis hits on March 11, 2011. •
With the advancement of the global economy, the coastal region has become heavily developed and densely populated and suffers significant damage potential considering various natural disasters, including tsunamis, as indicated by several catastrophic tsunami disasters in the 21st century. This study reviews the up-to-date tsunami research from two different viewpoints: tsunamis caused by ...
The study concluded that negative information related to (dis)trusted institutions intensifies the levels of (dis)trust among the public. Cvetkovich et al.'s (2002) study, however, used a continuum scale ranging from "−5: very great distrust" to "+5: very much trust" to measure trust and distrust, rather than using two different ...
Contact Data CONTACT: ResearchAndMarkets.com Laura Wood,Senior Press Manager [email protected] For E.S.T Office Hours Call 1-917-300-0470 For U.S./ CAN Toll Free Call 1-800-526-8630 For ...
Tsunami vertical evacuation (TVE) buildings have the potential to save many human lives in countries exposed to near-field tsunamis. Up to now, TVE research has examined three main topics separately: shelter siting, building benchmarks, and decision-making by evacuees. This study aims to integrate these topics to develop more comprehensive TVE planning frameworks. To this, we examined a ...