Thank you for visiting nature.com. You are using a browser version with limited support for CSS. To obtain the best experience, we recommend you use a more up to date browser (or turn off compatibility mode in Internet Explorer). In the meantime, to ensure continued support, we are displaying the site without styles and JavaScript.
- View all journals
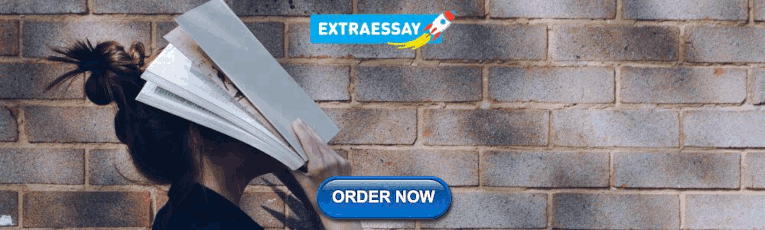
Fuel cells articles from across Nature Portfolio
A fuel cell is a device that generates electric energy through electrochemical reactions between an oxidizing agent and a fuel – a material that stores energy in chemical form. Both species are not stored in the fuel cell but must be supplied from external sources.
Latest Research and Reviews
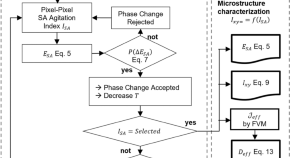
Theoretical analysis of the effect of isotropy on the effective diffusion coefficient in the porous and agglomerated phase of the electrodes of a PEMFC
- Romeli Barbosa
- L. C. Ordoñez
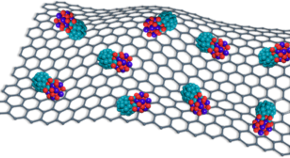
A strongly coupled Ru–CrO x cluster–cluster heterostructure for efficient alkaline hydrogen electrocatalysis
The synthesis of well-defined heterostructure interfaces can be leveraged to design advanced catalysts. Now a catalyst consisting of carbon-supported Janus particles with crystalline Ru and amorphous CrO x sides is shown to achieve high performance for both alkaline hydrogen oxidation and evolution reactions due to the synergy between both sides.
- Bingxing Zhang
- Jianmei Wang
- Wenping Sun
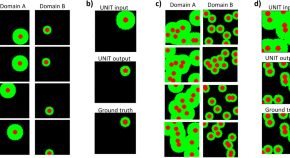
Prediction of electrode microstructure evolutions with physically constrained unsupervised image-to-image translation networks
- Anna Sciazko
- Yosuke Komatsu
- Naoki Shikazono
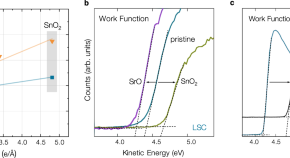
Engineering surface dipoles on mixed conducting oxides with ultra-thin oxide decoration layers
Improving materials for energy devices relates to an optimisation of their surfaces. Here authors show that surface modification with ultrathin oxide layers allows for a tailoring of the surface dipole and the work function of mixed ionic and electronic conducting oxides.
- Matthäus Siebenhofer
- Andreas Nenning
- Markus Kubicek
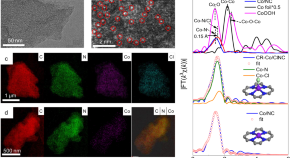
In situ modulating coordination fields of single-atom cobalt catalyst for enhanced oxygen reduction reaction
The electrochemical oxygen reduction reaction plays an important role in new energy technologies such as fuel cells and metal-air batteries. Here the authors present a cobalt catalyst with a symmetry-broken Cl−Co−N 4 moiety capable of dynamically modulating electron occupancy at active sites during practical reaction conditions to optimize oxygen reduction performance.
- Meihuan Liu
- Qinghua Liu
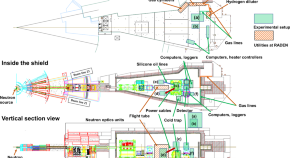
Experimental visualization of water/ice phase distribution at cold start for practical-sized polymer electrolyte fuel cells
Yuki Higuchi and colleagues report a method to directly observe the distribution of water and ice in large polymer electrolyte fuel cells (PEFCs). This information is critical for understanding the cold start performance of these cells. Their findings could guide the design of more effective coldstart protocols for PEFCs.
- Yuki Higuchi
- Wataru Yoshimune
- Yasutaka Nagai
News and Comment
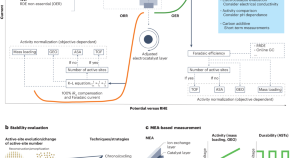
Best practices for oxygen electrocatalysis
Oxygen electrocatalysis is key for energy conversion and storage technologies such as fuel cells and water electrolysers. However, the measurement of the performance of electrocatalysts is not standardized. This Comment addresses emerging pitfalls in performance evaluation and discusses best practices for oxygen electrocatalysis.
- Daniel J. Zheng
- Yang Shao-Horn
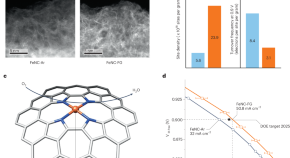
Modifying Fe–N interaction to boost catalytic performance
Iron–nitrogen–carbon (FeNC) catalysts are a viable alternative to platinum, but still lack the necessary performance. Now, pyrolysis under forming gas is found as a path to boosting their site density, activity and durability.
- Ulrike I. Kramm
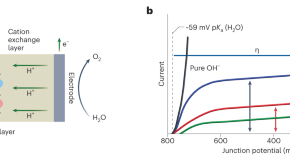
Ions block up the junction
Bipolar membranes are emerging as an enabling component in numerous energy-relevant electrochemical devices, but a greater understanding of how they operate in complex electrolytes is needed. Research now reveals that ionic species in mixed-ion electrolytes can block the heart of the membrane, the bipolar junction, hampering its performance.
- Sebastian Z. Oener
Costing out fuel cells
- James Gallagher
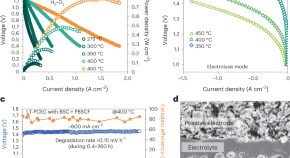
Ceramic electrochemical cells that operate at temperatures below 450 °C
Protonic ceramic electrochemical cells (PCECs) have the potential to operate below 450 °C, but their performance is poor at such temperatures. Through the development of a low-resistance electrolyte and the in situ formation of a composite positive electrode, a PCEC with good fuel cell and electrolysis cell performance at <450 °C is demonstrated.
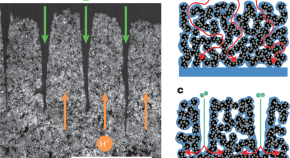
Into the groove
The wide deployment of fuel cells in transportation applications necessitates efficiency, lifetime, and cost improvements. Now, grooved electrodes with controlled design and composition address the limitations of conventional flat electrode structures, yielding improved fuel cell performance.
- Sara Cavaliere
Quick links
- Explore articles by subject
- Guide to authors
- Editorial policies

Hydrogen fuel cell electric vehicles: State of Art and outlooks
Ieee account.
- Change Username/Password
- Update Address
Purchase Details
- Payment Options
- Order History
- View Purchased Documents
Profile Information
- Communications Preferences
- Profession and Education
- Technical Interests
- US & Canada: +1 800 678 4333
- Worldwide: +1 732 981 0060
- Contact & Support
- About IEEE Xplore
- Accessibility
- Terms of Use
- Nondiscrimination Policy
- Privacy & Opting Out of Cookies
A not-for-profit organization, IEEE is the world's largest technical professional organization dedicated to advancing technology for the benefit of humanity. © Copyright 2024 IEEE - All rights reserved. Use of this web site signifies your agreement to the terms and conditions.

An official website of the United States government
The .gov means it’s official. Federal government websites often end in .gov or .mil. Before sharing sensitive information, make sure you’re on a federal government site.
The site is secure. The https:// ensures that you are connecting to the official website and that any information you provide is encrypted and transmitted securely.
- Publications
- Account settings
Preview improvements coming to the PMC website in October 2024. Learn More or Try it out now .
- Advanced Search
- Journal List
- v.11(5); 2021 May

Microbial fuel cells: a comprehensive review for beginners
A. s. vishwanathan.
WATER Laboratory, Department of Biosciences, Sri Sathya Sai Institute of Higher Learning, Prasanthi Nilayam, Puttaparthi, 515134 Andhra Pradesh India
Microbial fuel cells (MFCs) have shown immense potential as a one-stop solution for three major sustainability issues confronting the world today—energy security, global warming and wastewater management. MFCs represent a cross-disciplinary platform for research at the confluence of the natural and engineering sciences. The diversity of variables influencing performance of MFCs has garnered research interest across varied scientific disciplines since the beginning of this century. The increasing number of research publications has made it necessary to keep track of work being carried out by research groups across the globe and consolidate significant findings on a regular basis. Review articles are often the nodal points for beginners who are required to undertake an exploratory survey of literature to identify a suitable research problem. This ‘review of reviews’ is a ready-reckoner that directs readers to relevant reviews and research articles reporting significant developments in MFC research in the last two decades. The article also highlights the areas needing research attention which when addressed could take this technology a few more steps closer to practical implementation.
Introduction
The Earth presently plays host to almost 8 billion human beings (UN DESA Population Division 2019 ) and the number is expected to go up further and level out by the latter half of the Twenty-first century (Gonzalo et al. 2016 ). Sustainability of natural resources has been a cause for concern (Buhaug and Urdal 2013 ) due to ambitious social and economic goals. Dwindling reserves of fossil fuels (Hallenbeck and Ghosh 2009 ) account for over 80% of the world's primary energy consumption (Mohr et al. 2015 ). Greenhouse effect, a natural phenomenon that is chiefly responsible for the habitability of earth, appears to be assuming unmanageable proportions. Unregulated release of carbon dioxide and other greenhouse gases resulting from anthropocentric activities have led to increased absorption of infrared radiation from the sun leading to above-normal surface temperatures on earth (IPCC 2014 ). The need to curb such emissions underlines the search for sustainable, carbon–neutral sources of energy (Arent et al. 2011 ; Villano et al. 2012 ). Reinforcing the need to shift to renewable energy, Rittman ( 2008 ) specifically outlines the potential of microorganisms as a source of energy.
Urbanization is on the rise in developing nations (Buhaug and Urdal 2013 ) and the resultant increase in average income has ameliorated food preferences, putting pressure on water resources (de Fraiture and Wichelns 2010 ). The increased demand for water has impacted water availability (Haddeland et al. 2014 ) and has promoted reuse of wastewater for applications such as irrigation (Toze 2006 ) and landscaping. However, in many developing countries, advances in sanitation infrastructure and wastewater treatment have been outpaced by population growth (Qadir et al. 2010 ). As a result, many of them are on the lookout for reliable and low-cost means for treatment of domestic, agricultural and industrial wastewater to make it reusable (Massoud et al. 2009 ). An informative and well-illustrated review article by Larsen et al. ( 2016 ) discusses the need to adopt innovative strategies for arriving at resource-efficient solutions for issues related to urban water management.
Past and present of MFCs
Electrical effects resulting from microbial disintegration of organic compounds were first described by Potter ( 1911 ) over a hundred years ago. In the subsequent decades leading to the next century, there were only a few isolated reports of attempts to extend this fascinating discovery towards practical applications. Schröder ( 2011 ) traces the century-long history of microbial electrochemical systems from the time they were first reported, highlights significant milestones, succinctly outlines the reasons for the initial dearth of interest in taking this technology further, and finally describes the relevance and future scope of this discipline following its resurgence at the turn of the century.
Microbial Fuel Cells (MFCs) have been aptly described by Du et al. ( 2007 ) as “ bioreactors that convert the energy in the chemical bonds of organic compounds into electrical energy through catalytic activity of microorganisms under anaerobic conditions ”. Figure Figure1 1 is a graphical representation of a generic two-chambered MFC comprising an anode and a cathode chamber separated by a selectively permeable membrane. The microbes’ need for a compatible electron acceptor to deposit electrons is readily fulfilled by the anode of an MFC in the absence of a more suitable acceptor (Stams et al. 2006 ). These electrons collected by the anode are channelised across an external load (resistor) to harness usable energy. The final step of the electron transport occurs at the cathode in the presence of a terminal electron acceptor. Thus, a ‘quasi-engineered’ electron transport chain that mimics the bacterial respiratory chain forms the core of an MFC. Basic concepts relating to MFCs are presented in a lucidly written lecture text by Schröder ( 2018 ). The technical foundations and principles which form the basis of this technology are presented in comprehensive review articles by Logan et al. ( 2006 ) and Santoro et al. ( 2017 ). These microbe-catalysed electrochemical devices are viewed as a potential solution for wastewater management and as a source of sustainable and clean energy. To make this solution practically viable, research on microbial electrochemical technologies has primarily focused on four aspects, viz. minimizing electrochemical losses, improving performance efficiency, lowering working costs and scaling up systems for practical applications (Fig. (Fig.2 2 ).

Schematic of a generic two-chambered MFC

Four major focus areas of MFC research
A query submitted for the term ‘microbial fuel cells’ on the Web of Science™ platform of Clarivate Analytics (Fig. (Fig.3) 3 ) showed a gradual increase in the number of research articles on MFCs that were published in the years 2004–2020 in scientific, peer-reviewed journals. It must be noted that this figure serves to only emphasize the growth trend and that the output of a similar query in different search engines would obviously return varying numbers based on the websites and databases that are indexed by the respective algorithms.

Year-wise trend of publications based on a search on the Web of Science™ portal for the keyword 'microbial fuel cell' (2004–2020)
Among the different types of articles that are published in scientific journals, review articles represent a starting point for budding researchers and a vade mecum for established scientists. In general, reviews primarily serve to fulfil the following tasks:
- i. classifying the ever-growing information in a subject into relevant categories,
- ii. providing references to research papers that describe significant advancements, and
- iii. highlighting lacunae to be addressed by researchers.
This article has been compiled with the primary objective of aiding beginners to sift through the abundantly available scientific literature on MFCs by directing them to focused reviews and relevant breakthrough research articles highlighting significant advances in the field. The content has been divided into independent sub-sections pertaining to configuration, microbes, materials, performance characterization, scale-up and applications for the sake of convenience. The choice of references cited in this article is based entirely on their content and is not influenced by any intentional bias whatsoever.
MFC design and modeling
A wide variety of MFC configurations have been designed for specific applications and with the objective of improving performance by minimizing systemic losses. Some of the significant examples include air–cathode single-chamber MFCs (Liu and Logan 2004 ), flat-plate MFCs (Min and Logan 2004 ), upflow MFCs (He et al. 2005 ), tubular MFCs (Rabaey et al. 2005 ), membrane-electrode assembly MFCs (Pham et al. 2005 ), stacked MFCs (Aelterman et al. 2006 ), separator-electrode assembly MFCs (Ahn and Logan 2012 ). However, the most commonly reported are the two-chambered, 'H-shaped' MFCs which, despite their low current output, have been the most convenient for optimizing performance of new components and characterising operating conditions (Logan et al. 2006 ). Figure Figure4 4 (adapted) presents some of the different experimental designs that have been used in MFC studies and reported in literature.

Different designs used in MFC studies: a salt bridge MFC; b , c upflow MFCs; d flat-plate MFC; e h-shaped MFC; f , g single-chamber MFCs; h stacked MFC (The figure has been reprinted (adapted) with permission from Logan BE, Hamelers B, Rozendal R, et al. (2006) Microbial fuel cells: Methodology and technology. Environmental Science & Technology 40:5181–5192. https://doi.org/10.1021/es0605016 .
Copyright © 2006 American Chemical Society.)
Discussing essential aspects to be considered while designing MFCs for various practical applications, an article by Logan et al. ( 2015 ) highlights the importance of electrode configuration and source of organic substrate in determining performance. Modeling studies, which facilitate detailed analyses of factors affecting the performance of MFCs (Jadhav et al. 2020a ), include mathematical modeling (Deb et al. 2020 ), computer simulations (Xia et al. 2018 ), neural network modeling (Ma et al. 2019 ) and electrochemical modeling (Kadivarian and Karamzadeh 2020 ). Given the diversity of dependent variables that can determine the performance of MFCs (Oliveira et al. 2013 ; Zhang et al. 2019a ), analysing their influence to arrive at a valid conclusion depends to a considerable extent on the number of replicates of an experiment because repeatability is not necessarily assured (Larrosa et al. 2009 ).
Electroactive microbes
Microbes play a key role in an MFC by catalysing the release of electrons from energy rich bonds of organic substrates under anoxic conditions. Review articles by Pant et al. ( 2010b ) and Pandey et al. ( 2016 ) describe different pure substrates and types of wastewater that have been used as a carbon source for microbes in MFCs. The electrons released in this process of oxidation travel through versatile microbial electron transport chains (Fredrickson et al. 2008 ; Kracke et al. 2015 ) which comprise serially arranged conductive protein complexes, cytochromes, nanowires and redox proteins (Costa et al. 2018 ) before being donated to the anode of the MFC. Schröder explains the fundamental mechanisms and energy considerations of anodic electron transfer in a classic review ( 2007 ). Electron transfer between microbes and the electrode (Lovley 2012 ; Kumar et al. 2017 ) can be either indirect—mediated by naturally produced or artificially added redox shuttles (Martinez and Alvarez 2018 )—or by direct extracellular electron transfer (Yang et al. 2012 ) (Fig. (Fig.5). 5 ). Glasser et al. ( 2017 ) provide valuable insights into endogenous extracellular electron shuttles while Lovley ( 2017 ) describes the processes associated with direct interspecies electron transfer which enables long-distance transport of electrons in bioelectrochemical systems. Dynamics of electron transfer within microbes (intra), between microbial species (inter), and at the microbe-electrode interface have been detailed in a review article by Zheng et al. ( 2020 ).

Direct (solid lines) and indirect (dotted lines) electron transfer from bacteria to the anode
Mixed consortia of electrogenic and electrotrophic microbes (Logan 2009 ; Logan et al. 2019 ) are known to contribute more effectively to production of current in MFCs as compared to pure cultures of bacteria. This difference could be attributed to synergistic interactions between syntrophic microbial species resulting in effective utilization of available substrates (Kiely et al. 2011 ) by the formation of electrochemically active biofilms (Borole et al. 2011 ; Babauta et al. 2012 ; Reguera 2018 ; Kiran and Patil 2019 ). Growth and performance of electroactive biofilms can be enhanced (Li et al. 2018a ) by selectively controlling growth conditions (Doyle and Marsili 2015 , 2018 ), using synthetic biology (Glaven 2019 ) and adopting engineering approaches (Angelaalincy et al. 2018 ; Chiranjeevi and Patil 2020 ). Communities of microbial consortia have also been profiled and characterized using ‘omics’ technologies (Rittmann et al. 2008 ; Lacerda and Reardon 2009 ; Moran et al. 2013 ; Franzosa et al. 2015 ; Kouzuma et al. 2018 ), flow-cytometric approaches (Koch et al. 2014 ), computational tools (Haft and Tovchigrechko 2012 ; Segata et al. 2013 ) and statistical analysis (Buttigieg and Ramette 2014 ) to obtain insights from a structural and functional perspective (Zhi et al. 2014 ).
Electrodes and separators
Efficient electrode materials in MFCs must essentially be biocompatible, electrically conductive, non-corrosive and electrochemically stable. Wei et al. ( 2011 ), in their detailed review article, analyse the advantages and disadvantages of different materials used as electrodes in MFCs and discuss the prospects of electrode development. Assessing the performance of electrodes and separators (Hamelers et al. 2010 ) and use of low-cost materials such as ceramics (Winfield et al. 2016 ), ligno-cellulosic material (Mehta et al. 2020 ) and biochar (Chakraborty et al. 2020a ) without significantly compromising on efficiency is important for design of efficient MFCs. Breheny et al. ( 2019 ) discuss critical aspects for improvement of bioelectrodes in MFCs and Pasternak et al. ( 2020 ) present a new dimension for enhancing performance of microbial electrochemical systems using surfactants.
Anodes serve as the substratum for biofilm formation and also function as current collectors in MFCs. Among different materials that have been reported, carbon is most preferred for anodes because of its versatility, non-reactivity, high electrical conductivity and biocompatibility (Logan 2008 ). While carbon cloth and carbon felt provide more room for colonization of microbes by virtue of being more porous compared to graphite sheets or carbon paper, the innovative introduction of graphite brush anodes (Logan et al. 2007 ) enabled the incorporation of larger surface area of electrodes for a given volume of the reactor. The high conductivity and surface area provided by nanomaterials resulted in their use in the anode chamber of MFCs (Liu et al. 2020 ). Gnana kumar et al. ( 2013 ) describe the features of anode materials used in MFCs and different processing techniques that can improve efficiency of bacterial adhesion, electron capture and transfer. A comparative account of conventional and modified anodes (Cai et al. 2020 ) opens up a new window for understanding the characteristics of anode materials and paves the way for development of next generation MFC anodes.
Cathodes provide a common interface for the culmination of the microbial electron transfer process in an MFC resulting in the confluence of electrons, protons and the terminal electron acceptor. On account of their complex role, cathodes have been considered as a critical point to determine the efficiency of MFCs (Rabaey and Keller 2008 ). Based on the type of electron acceptor used (He et al. 2015 ), cathodes can be classified as chemical or biological. Oxygen is often preferred as a terminal electron acceptor due to its ubiquity and propensity to get reduced to water. However, poor kinetics of the oxygen reduction reaction led to the use of expensive, precious-metal catalysts such as platinum at the cathode. Studies that focused on reduction of operating costs (Zhang et al. 2009 ) eventually led the way to development of more economical, alternate cathode materials based on carbon (Peera et al. 2020 ) and nanocomposites (Dessie et al. 2020 ) devoid of precious metals for improving efficiency of the oxygen reduction reaction (Yuan et al. 2016 ). Erable et al. ( 2012 ) describe the application of microbes to catalyse the rate-limiting oxygen reduction reaction at the cathode. Biocathodes (He and Angenent 2006 ), comprising electrotrophic microbes that can directly accept electrons from the electrode (Lovley 2011 ), can overcome many of the shortcomings encountered using chemical cathodes and are now being actively pursued as a topic of research interest (Song et al. 2019 ).
A separator in an MFC is a physical barrier that allows charges to pass through but serves as a hurdle to prevent direct electrical contact between the anode and cathode. In the early years, proton exchange membranes such as Nafion® were used in MFCs to selectively allow only protons to the cathode chamber of an MFC (Rahimnejad et al. 2014 ). Eliminating the use of a proton-specific, separating membrane in MFCs (Jang et al. 2004 ) was a significant breakthrough for reducing operation costs, but it brought along the twin drawbacks of oxygen diffusion into the anoxic anode chamber and short circuiting of electrons between the anode and cathode, both of which when unregulated have a detrimental impact on performance efficiency. In subsequent years, expensive membranes were substituted with alternatives like Zirfon® (Pant et al. 2010a ; Pasupuleti et al. 2016 ) and low-cost materials having more general transport properties such as ion exchange membranes (Leong et al. 2013 ), ceramic filtration membranes (Yang et al. 2016a ), polymeric membrane separators (Bakonyi et al. 2018 ), sand/activated carbon separators (Gao et al. 2018 ), silk fibroin membranes (Pasternak et al. 2019 ) and polystyrene (Mathuriya and Pant 2019 ).
Performance characterization
Electrochemical techniques and tools are used to analyze the effect of modifications made to MFCs with the objective of minimizing electrochemical losses and enhancing performance efficiency. Rimboud et al. ( 2014 ) present a detailed perspective on the factors to be considered while designing anodes for microbial electrochemical systems. Electroactivity of biofilms has been characterized using techniques such as cyclic voltammetry (Gimkiewicz and Harnisch 2013 ), electrochemical impedance spectroscopy (ter Heijne et al. 2015 ), confocal resonance Raman microscopy (Virdis et al. 2016 ), interdigitated electrode array (Yates et al. 2018 ) and other methods. Technical aspects such as internal resistance (Zhang and Liu 2010 ) and anode potential (Aelterman et al. 2008 ; Wagner et al. 2010 ; Zhu et al. 2013 ) have to be understood and commonly encountered issues such as power overshoot (Watson and Logan 2011 ; Winfield et al. 2011 ) and voltage reversal (Kim et al. 2020 ) must be analysed to minimise losses and enhance performance of MFCs. Tutorial articles provide the necessary support to beginners to understand fundamental concepts in electronic circuitry (Sánchez et al. 2020 ), choice of electrode configurations and operating conditions for electroanalysis (Zhao et al. 2009 ) and nuances of techniques such as cyclic voltammetry (Harnisch and Freguia 2012 ; Elgrishi et al. 2018 ) and electrochemical impedance spectroscopy (He and Mansfeld 2009 ). Other useful reviews outline performance indicators (Sharma et al. 2014 ) and terms used to describe performance of microbial electrochemical systems (Wang and He 2020 ). Challenges encountered due to the diverse configurations of MFCs and different techniques available for characterizing activity of electroactive microbes can be addressed by having a standardized framework (Harnisch and Rabaey 2012 ) and fundamental guidelines to plan experiments, analyse observations and report results in a more meaningful manner (Logan 2012 ).
Schröder ( 2011 ) reported that the performance of MFCs improved by close to three orders of magnitude—from few μA/cm 2 to over 1 mA/cm 2 —during the first decade of this century. Microscale (Wang et al. 2011 ; Choi 2015 ) and microfluidic (Yang et al. 2016b ; Parkhey and Sahu 2020 ) MFCs have shown enhanced performance in terms of power production. Although μL and mL scale laboratory experiments provide cues and clues regarding different mechanisms involved in the functioning of MFCs, systemic understanding obtained from such studies must be transferred and translated (Janicek et al. 2014 ; Butti et al. 2016 ) to enable setting up of pilot-scale systems (Logan 2010 ). Knowledge of the different components and processes involved is critical to make upscaling of MFCs practically feasible (Logan et al. 2015 ). Significant progress has been achieved over the past decade in developing scaled-up MFC systems for practical applications (Gajda et al. 2018 ; Abdallah et al. 2019 ; Jadhav et al. 2020b ).
Applications
The primary application of MFCs is wastewater treatment with concomitant production of electricity (Pant et al. 2012 ). Lefebvre et al. ( 2011 ) describe energetics of MFCs with the objective of developing a self-sustaining domestic wastewater treatment process (Oh et al. 2010 ). Harnessing the potential of MFCs as a power source (Wang et al. 2015 ) and for production of valuable products by microbial electrosynthesis (Rabaey and Rozendal 2010 ; Harnisch and Urban 2018 ) requires an in-depth understanding of factors that limit performance (Sleutels et al. 2012 ) along with the principles of energy capture and storage (Sun et al. 2016 ).
Evolution of microbes has favoured the diversification of MFCs into a number of technologies (Schröder and Harnisch 2017 ) with varied applications (Schröder et al. 2015 ), resulting in the more generic term ‘microbial electrochemical cells’ (MXCs) (Fig. (Fig.6). 6 ). Table Table1 1 presents an overview of the multifarious applications of microbial electrochemical technologies and provides references to recently published review articles. Torres ( 2014 ) emphasizes on the need to “ identify, understand and predict ” different phenomena that govern the performance of such systems.

Diversification of microbial electrochemical cells
Applications of microbial electrochemical technologies
Conclusions
The pursuit of alternate sources of energy due to the consequences of an unabated rise in human population has directed attention of researchers towards MFCs which essentially perform a dual-role of wastewater treatment and clean energy production. The steady increase in the number of research articles published on MFCs (Md Khudzari et al. 2018 ) over the last 15 years is an indicator of the steadfastness and commitment of the research community. Moreover, among the several books written or compiled on MFCs, the following three which encapsulate significant advances pertaining to construction, characterization, applications and diversification of this technology deserve a mention: Microbial fuel cells (Wiley-Interscience) (Logan 2008 ), Microbial Electrochemical Technology: Sustainable Platform for Fuels, Chemicals and Remediation (Elsevier) (Venkata Mohan et al. 2018 ) and Microbial Electrochemical Technologies (Routledge/CRC Press) (Tiquia-Arashiro and Pant 2020 ).
However, what goes unnoticed is the increasing number of students opting for MFCs and related technologies for their projects at high school and university levels due to the societal relevance of these topics. Considering the fact that data presented in such project reports often trigger more specialized and resource intensive studies, it might be worthwhile exploring the creation of a platform to document and collate promising results from such projects. Moreover, acknowledging the efforts of these young contributors, in a noteworthy manner, in research publications resulting from these leads would encourage more exploratory studies by students.
Planning for a student project or designing a research experiment might seem to be an elementary process because of the seemingly limitless possibilities that exist to observe the effects of tweaking the diverse physico-chemical and biological variables that directly or indirectly influence MFC performance. However, it would preferable to align the scope of such investigations to the aforementioned four major objectives of MFC research—keeping electrochemical losses under check, boosting electron transfer efficiency, bringing down operational costs and upscaling systems for practical applications—so that it results in a significant contribution to the existing body of knowledge.
MFCs have been prototyped in various shapes and sizes; each new configuration presenting an improvement over the others in some aspect of performance. Alterations to MFC configurations will continue in the quest for models that can be effectively implemented on a large scale. The understanding of variables associated with MFC performance has certainly improved over the years and the inventory of materials that improve the performance of MFCs is also continually expanding. These must go hand-in-hand with efforts to curb costs of scaled-up systems. Agricultural wastes, for instance, are carbon-rich materials that can be carbonized and exploited as low-cost electrode material. However, such substitutions can imply a trade-off with performance efficiency, opening up new avenues for detailed optimization studies using statistical methods such as response surface methodology.
Carrying out mathematical modeling and computer simulations can provide a near-realistic estimate of the optimal configuration, components and operating parameters to be employed under a given set of conditions for specific applications. Designing high-throughput methods for screening performance of components and operating parameters is a challenge that is still relevant and needs attention; especially because of the inter-relationships among the physico-chemical and variables influencing MFCs.
The fact remains, however, that the biological component will always be a complex variable that cannot be precisely modelled; and thus needs more focused attention for unravelling unknown facets of bacterial metabolism and energetics specifically in the context of bioelectrochemical systems. Community dynamics of microbial consortia in electroactive biofilms powering microbial electrochemical systems are still being understood. In silico analyses of genomic and proteomic data in openly available repositories such as the National Center for Biotechnology Information ( www.ncbi.nlm.nih.gov ), Worldwide Protein Data Bank ( www.wwpdb.org ), European Bioinformatics Institute ( www.ebi.ac.uk ) and many others make it possible to gain insights into the mechanistic aspects of bacterial electron transfer systems and processes. Metagenomic approaches for microbial community profiling are gaining relevance as they also account for bacteria which cannot be easily cultured in laboratory conditions. Sophisticated protein modelling and visualization tools available today can uncover hitherto unknown aspects of bacterial respiratory proteins and biofilm-associated proteins ( www.biofilms.biosim.pt ). Protocols employed for control of biofilms, especially in the food and healthcare sectors where they are known to be a nuisance, could provide useful hints to develop methods for promoting their growth in bioelectrochemical systems.
Tutorial articles on a design of experiments approach to effectively plan experiments and on electrochemical techniques for performance characterization will help in hand-holding students and scientists from diverse backgrounds to set-up the working environment. Limited access to equipment for electrochemical characterization, often not affordable for school and colleges not having established routes to obtain funding, can be a major bottleneck for obtaining reliable results. Efforts to bring down costs of basic instrumentation using micro-controllers (Meloni 2016 ; Li et al. 2018b ) would bolster the quality of results of academic projects relating to MFCs.
As evidenced by literature, what began as a fascinating phenomenon over a century ago has evolved into a fertile avenue for researchers from different disciplines to converge and contribute (Fig. (Fig.7). 7 ). The journey of MFCs seems to be akin to the folk-tale of the six blind men who tried to describe an elephant; each one basing his judgement on a part of the animal that he felt with his hands. It was only when all their views were rationally consolidated that they perceived the bigger picture and came to the conclusion that an elephant is actually much more than just fan-like ears, pillar-like legs, spear-like tusks, a tube-like trunk, a rope-like tail and a wall-like body. Multidisciplinary approaches and transdisciplinary efforts have demolished traditional barriers and bridged the gaps which had prevailed in the earlier years on account of adopting a simplex approach towards harnessing energy from wastewater using microbial catalysts.

MFC research can be classified under many subject areas
The plethora of applications conceptualized, demonstrated and envisaged portray microbial electrochemical technologies as a 'magic bullet' for impending sustainability crises. However, global sustainability issues can be successfully addressed by MFCs only if the efforts are collated, structured and directed towards a common objective of practical application of these technologies. Singular efforts in multiple directions would only result in a tug-of-war between research groups of varying skills and capabilities. Rather, a collaborative approach at the regional level could optimally utilize the available pool of expertise for the output of MFCs to reach usable levels in large scale applications at affordable costs. Established groups must take the lead in their respective regions for drawing up a framework and charting a roadmap for other fledgling groups to also contribute in their respective niche areas towards a common objective of societal benefit. The untiring efforts of the International Society for Microbial Electrochemistry and Technology ( www.is-met.org ) in this direction will certainly go a long way in making this possible. As it has been rightly said: “ Coming together is a beginning. Keeping together is progress. Working together is success. ”
Acknowledgements
This work is dedicated to Bhagawan Sri Sathya Sai Baba, the founder chancellor of the Sri Sathya Sai Institute of Higher Learning. Continued support of my research supervisors—Prof. Govind Rao, UMBC and Prof. S. Siva Sankara Sai, SSSIHL—is gratefully acknowledged. Insightful suggestions provided by my students—Sahashransu Satyajeet Mahapatra and Mayur Mukhi—are greatly appreciated.
Declarations
The author declares no conflict of interest in the publication.
- Abdallah M, Feroz S, Alani S, et al. Continuous and scalable applications of microbial fuel cells: a critical review. Rev Environ Sci Biotechnol. 2019; 18 :543–578. doi: 10.1007/s11157-019-09508-x. [ CrossRef ] [ Google Scholar ]
- Aelterman P, Rabaey K, Pham HT, et al. Continuous electricity generation at high voltages and currents using stacked microbial fuel cells. Environ Sci Technol. 2006; 40 :3388–3394. doi: 10.1021/es0525511. [ PubMed ] [ CrossRef ] [ Google Scholar ]
- Aelterman P, Freguia S, Keller J, et al. The anode potential regulates bacterial activity in microbial fuel cells. Appl Microbiol Biotechnol. 2008; 78 :409–418. doi: 10.1007/s00253-007-1327-8. [ PubMed ] [ CrossRef ] [ Google Scholar ]
- Ahn Y, Logan BE. A multi-electrode continuous flow microbial fuel cell with separator electrode assembly design. Appl Microbiol Biotechnol. 2012; 93 :2241–2248. doi: 10.1007/s00253-012-3916-4. [ PubMed ] [ CrossRef ] [ Google Scholar ]
- Angelaalincy MJ, Navanietha Krishnaraj R, Shakambari G, et al. Biofilm engineering approaches for improving the performance of microbial fuel cells and bioelectrochemical systems. Front Energy Res. 2018; 6 :1–12. doi: 10.3389/fenrg.2018.00063. [ CrossRef ] [ Google Scholar ]
- Arent DJ, Wise A, Gelman R. The status and prospects of renewable energy for combating global warming. Energy Econ. 2011; 33 :584–593. doi: 10.1016/j.eneco.2010.11.003. [ CrossRef ] [ Google Scholar ]
- Babauta JT, Renslow R, Lewandowski Z, Beyenal H. Electrochemically active biofilms: facts and fiction. A review. Biofouling. 2012; 28 :789–812. doi: 10.1080/08927014.2012.710324. [ PMC free article ] [ PubMed ] [ CrossRef ] [ Google Scholar ]
- Bakonyi P, Koók L, Kumar G, et al. Architectural engineering of bioelectrochemical systems from the perspective of polymeric membrane separators: a comprehensive update on recent progress and future prospects. J Memb Sci. 2018; 564 :508–522. doi: 10.1016/j.memsci.2018.07.051. [ CrossRef ] [ Google Scholar ]
- Borole AP, Reguera G, Ringeisen B, et al. Electroactive biofilms—current status and future research needs. Energy Environ Sci. 2011; 4 :4813. doi: 10.1039/c1ee02511b. [ CrossRef ] [ Google Scholar ]
- Breheny M, Bowman K, Farahmand N, et al. Biocatalytic electrode improvement strategies in microbial fuel cell systems. J Chem Technol Biotechnol. 2019; 94 :2081–2091. doi: 10.1002/jctb.5916. [ CrossRef ] [ Google Scholar ]
- Buhaug H, Urdal H. An urbanization bomb? Population growth and social disorder in cities. Glob Environ Chang. 2013; 23 :1–10. doi: 10.1016/j.gloenvcha.2012.10.016. [ CrossRef ] [ Google Scholar ]
- Butti SK, Velvizhi G, Sulonen MLK, et al. Microbial electrochemical technologies with the perspective of harnessing bioenergy: maneuvering towards upscaling. Renew Sustain Energy Rev. 2016; 53 :462–476. doi: 10.1016/j.rser.2015.08.058. [ CrossRef ] [ Google Scholar ]
- Buttigieg PL, Ramette A. A guide to statistical analysis in microbial ecology: a community-focused, living review of multivariate data analyses. FEMS Microbiol Ecol. 2014; 90 :543–550. doi: 10.1111/1574-6941.12437. [ PubMed ] [ CrossRef ] [ Google Scholar ]
- Cai T, Meng L, Chen G, et al. Application of advanced anodes in microbial fuel cells for power generation: a review. Chemosphere. 2020 doi: 10.1016/j.chemosphere.2020.125985. [ PubMed ] [ CrossRef ] [ Google Scholar ]
- Caizán-Juanarena L, Borsje C, Sleutels T, et al. Combination of bioelectrochemical systems and electrochemical capacitors: principles, analysis and opportunities. Biotechnol Adv. 2020; 39 :107456. doi: 10.1016/j.biotechadv.2019.107456. [ PMC free article ] [ PubMed ] [ CrossRef ] [ Google Scholar ]
- Cecconet D, Sabba F, Devecseri M, et al. In situ groundwater remediation with bioelectrochemical systems: a critical review and future perspectives. Environ Int. 2020; 137 :105550. doi: 10.1016/j.envint.2020.105550. [ PubMed ] [ CrossRef ] [ Google Scholar ]
- Chakraborty I, Sathe SM, Dubey BK, Ghangrekar MM. Waste-derived biochar: applications and future perspective in microbial fuel cells. Bioresour Technol. 2020; 312 :123587. doi: 10.1016/j.biortech.2020.123587. [ PubMed ] [ CrossRef ] [ Google Scholar ]
- Chakraborty I, Sathe SM, Khuman CN, Ghangrekar MM. Bioelectrochemically powered remediation of xenobiotic compounds and heavy metal toxicity using microbial fuel cell and microbial electrolysis cell. Mater Sci Energy Technol. 2020; 3 :104–115. doi: 10.1016/j.mset.2019.09.011. [ CrossRef ] [ Google Scholar ]
- Chandrasekhar K, Kumar G, Venkata Mohan S, et al. Microbial Electro-Remediation (MER) of hazardous waste in aid of sustainable energy generation and resource recovery. Elsevier BV; 2020. [ Google Scholar ]
- Chiranjeevi P, Patil SA. Strategies for improving the electroactivity and specific metabolic functionality of microorganisms for various microbial electrochemical technologies. Biotechnol Adv. 2020; 39 :107468. doi: 10.1016/j.biotechadv.2019.107468. [ PubMed ] [ CrossRef ] [ Google Scholar ]
- Choi S. Microscale microbial fuel cells: advances and challenges. Biosens Bioelectron. 2015; 69 :8–25. doi: 10.1016/j.bios.2015.02.021. [ PubMed ] [ CrossRef ] [ Google Scholar ]
- Chu N, Liang Q, Jiang Y, Zeng RJ. Microbial electrochemical platform for the production of renewable fuels and chemicals. Biosens Bioelectron. 2020; 150 :111922. doi: 10.1016/j.bios.2019.111922. [ PubMed ] [ CrossRef ] [ Google Scholar ]
- Costa NL, Clarke TA, Philipp LA, et al. Electron transfer process in microbial electrochemical technologies: the role of cell-surface exposed conductive proteins. Bioresour Technol. 2018; 255 :308–317. doi: 10.1016/j.biortech.2018.01.133. [ PubMed ] [ CrossRef ] [ Google Scholar ]
- Das S, Das S, Das I, Ghangrekar MM. Application of bioelectrochemical systems for carbon dioxide sequestration and concomitant valuable recovery: a review. Mater Sci Energy Technol. 2019; 2 :687–696. doi: 10.1016/j.mset.2019.08.003. [ CrossRef ] [ Google Scholar ]
- de Fraiture C, Wichelns D. Satisfying future water demands for agriculture. Agric Water Manag. 2010; 97 :502–511. doi: 10.1016/j.agwat.2009.08.008. [ CrossRef ] [ Google Scholar ]
- Deb D, Patel R, Balas VE. A review of control-oriented bioelectrochemical mathematical models of microbial fuel cells. Processes. 2020; 8 :583. doi: 10.3390/pr8050583. [ CrossRef ] [ Google Scholar ]
- Dessie Y, Tadesse S, Eswaramoorthy R. Review on manganese oxide based biocatalyst in microbial fuel cell: nanocomposite approach. Mater Sci Energy Technol. 2020; 3 :136–149. doi: 10.1016/j.mset.2019.11.001. [ CrossRef ] [ Google Scholar ]
- Doyle LE, Marsili E. Methods for enrichment of novel electrochemically-active microorganisms. Bioresour Technol. 2015; 195 :273–282. doi: 10.1016/j.biortech.2015.07.025. [ PubMed ] [ CrossRef ] [ Google Scholar ]
- Doyle LE, Marsili E. Weak electricigens: a new avenue for bioelectrochemical research. Bioresour Technol. 2018; 258 :354–364. doi: 10.1016/j.biortech.2018.02.073. [ PubMed ] [ CrossRef ] [ Google Scholar ]
- Du Z, Li H, Gu T. A state of the art review on microbial fuel cells: a promising technology for wastewater treatment and bioenergy. Biotechnol Adv. 2007; 25 :464–482. doi: 10.1016/j.biotechadv.2007.05.004. [ PubMed ] [ CrossRef ] [ Google Scholar ]
- Elgrishi N, Rountree KJ, McCarthy BD, et al. A practical beginner’s guide to cyclic voltammetry. J Chem Educ. 2018; 95 :197–206. doi: 10.1021/acs.jchemed.7b00361. [ CrossRef ] [ Google Scholar ]
- Elmaadawy K, Liu B, Hu J, et al. Performance evaluation of microbial fuel cell for landfill leachate treatment: research updates and synergistic effects of hybrid systems. J Environ Sci (China) 2020; 96 :1–20. doi: 10.1016/j.jes.2020.05.005. [ PubMed ] [ CrossRef ] [ Google Scholar ]
- ElMekawy A, Hegab HM, Pant D, Saint CP. Bio-analytical applications of microbial fuel cell–based biosensors for onsite water quality monitoring. J Appl Microbiol. 2018; 124 :302–313. doi: 10.1111/jam.13631. [ PubMed ] [ CrossRef ] [ Google Scholar ]
- Erable B, Féron D, Bergel A. Microbial catalysis of the oxygen reduction reaction for microbial fuel cells: a review. Chemsuschem. 2012; 5 :975–987. doi: 10.1002/cssc.201100836. [ PubMed ] [ CrossRef ] [ Google Scholar ]
- Franzosa EA, Hsu T, Sirota-Madi A, et al. Sequencing and beyond: integrating molecular “omics” for microbial community profiling. Nat Rev Microbiol. 2015; 13 :360–372. doi: 10.1038/nrmicro3451. [ PMC free article ] [ PubMed ] [ CrossRef ] [ Google Scholar ]
- Fredrickson JK, Romine MF, Beliaev AS, et al. Towards environmental systems biology of Shewanella. Nat Rev Microbiol. 2008; 6 :592–603. doi: 10.1038/nrmicro1947. [ PubMed ] [ CrossRef ] [ Google Scholar ]
- Gajda I, Greenman J, Ieropoulos IA. Recent advancements in real-world microbial fuel cell applications. Curr Opin Electrochem. 2018; 11 :78–83. doi: 10.1016/j.coelec.2018.09.006. [ PMC free article ] [ PubMed ] [ CrossRef ] [ Google Scholar ]
- Gao C, Liu L, Yang F. A novel bio-electrochemical system with sand/activated carbon separator, Al anode and bio-anode integrated micro-electrolysis/electro-flocculation cost effectively treated high load wastewater with energy recovery. Bioresour Technol. 2018; 249 :24–34. doi: 10.1016/j.biortech.2017.09.134. [ PubMed ] [ CrossRef ] [ Google Scholar ]
- Gimkiewicz C, Harnisch F. Waste water derived electroactive microbial biofilms: growth, maintenance, and basic characterization. J Vis Exp. 2013 doi: 10.3791/50800. [ PMC free article ] [ PubMed ] [ CrossRef ] [ Google Scholar ]
- Glasser NR, Saunders SH, Newman DK. The colorful world of extracellular electron shuttles. Annu Rev Microbiol. 2017; 71 :731–751. doi: 10.1146/annurev-micro-090816-093913. [ PMC free article ] [ PubMed ] [ CrossRef ] [ Google Scholar ]
- Glaven SM. Bioelectrochemical systems and synthetic biology: more power, more products. Microb Biotechnol. 2019; 12 :819–823. doi: 10.1111/1751-7915.13456. [ PMC free article ] [ PubMed ] [ CrossRef ] [ Google Scholar ]
- Gonzalo JA, Alfonseca M, Muñoz F-F. World population: past, present, & future. Singapore: World Scientific; 2016. [ Google Scholar ]
- Greenman J, Gajda I, Ieropoulos I. Microbial fuel cells (MFC) and microalgae; photo microbial fuel cell (PMFC) as complete recycling machines. Sustain Energy Fuels. 2019; 3 :2546–2560. doi: 10.1039/c9se00354a. [ CrossRef ] [ Google Scholar ]
- Gurjar R, Behera M. Treatment of organic fraction of municipal solid waste in bioelectrochemical systems: a review. J Hazardous Toxic Radioact Waste. 2020; 24 :04020018. doi: 10.1061/(asce)hz.2153-5515.0000505. [ CrossRef ] [ Google Scholar ]
- Haddeland I, Heinke J, Biemans H, et al. Global water resources affected by human interventions and climate change. Proc Natl Acad Sci U S A. 2014; 111 :3251–3256. doi: 10.1073/pnas.1222475110. [ PMC free article ] [ PubMed ] [ CrossRef ] [ Google Scholar ]
- Haft DH, Tovchigrechko A. High-speed microbial community profiling. Nat Methods. 2012; 9 :793–794. doi: 10.1038/nmeth.2080. [ PubMed ] [ CrossRef ] [ Google Scholar ]
- Hallenbeck PC, Ghosh D. Advances in fermentative biohydrogen production: the way forward? Trends Biotechnol. 2009; 27 :287–297. doi: 10.1016/j.tibtech.2009.02.004. [ PubMed ] [ CrossRef ] [ Google Scholar ]
- Hamelers HVM, Ter Heijne A, Sleutels THJ, a, , et al. New applications and performance of bioelectrochemical systems. Appl Microbiol Biotechnol. 2010; 85 :1673–1685. doi: 10.1007/s00253-009-2357-1. [ PubMed ] [ CrossRef ] [ Google Scholar ]
- Harnisch F, Freguia S. A basic tutorial on cyclic voltammetry for the investigation of electroactive microbial biofilms. Chem An Asian J. 2012; 7 :466–475. doi: 10.1002/asia.201100740. [ PubMed ] [ CrossRef ] [ Google Scholar ]
- Harnisch F, Rabaey K. The diversity of techniques to study electrochemically active biofilms highlights the need for standardization. Chemsuschem. 2012; 5 :1027–1038. doi: 10.1002/cssc.201100817. [ PubMed ] [ CrossRef ] [ Google Scholar ]
- Harnisch F, Urban C. Electrobiorefineries: unlocking the synergy of electrochemical and microbial conversions. Angew Chemie Int Ed. 2018; 57 :10016–10023. doi: 10.1002/anie.201711727. [ PubMed ] [ CrossRef ] [ Google Scholar ]
- He Z, Angenent LT. Application of bacterial biocathodes in microbial fuel cells. Electroanalysis. 2006; 18 :2009–2015. doi: 10.1002/elan.200603628. [ CrossRef ] [ Google Scholar ]
- He Z, Mansfeld F. Exploring the use of electrochemical impedance spectroscopy (EIS) in microbial fuel cell studies. Energy Environ Sci. 2009; 2 :215. doi: 10.1039/b814914c. [ CrossRef ] [ Google Scholar ]
- He Z, Minteer SD, Angenent LT. Electricity generation from artificial wastewater using an upflow microbial fuel cell. Environ Sci Technol. 2005; 39 :5262–5267. doi: 10.1021/es0502876. [ PubMed ] [ CrossRef ] [ Google Scholar ]
- He C-S, Mu Z-X, Yang H-Y, et al. Electron acceptors for energy generation in microbial fuel cells fed with wastewaters: a mini-review. Chemosphere. 2015; 140 :12–17. doi: 10.1016/j.chemosphere.2015.03.059. [ PubMed ] [ CrossRef ] [ Google Scholar ]
- Hoareau M, Erable B, Bergel A. Microbial electrochemical snorkels (MESs): a budding technology for multiple applications. A mini review. Electrochem commun. 2019; 104 :106473. doi: 10.1016/j.elecom.2019.05.022. [ CrossRef ] [ Google Scholar ]
- IPCC (2014) Climate Change 2014: Synthesis Report. Contribution of Working Groups I, II and III to the Fifth Assessment Report of the Intergovernmental Panel on Climate Change. Geneva, Switzerland
- Jadhav DA, Carmona-Martínez AA, Chendake AD, et al. Modeling and optimization strategies towards performance enhancement of microbial fuel cells. Bioresour Technol. 2020 doi: 10.1016/j.biortech.2020.124256. [ PubMed ] [ CrossRef ] [ Google Scholar ]
- Jadhav DA, Das I, Ghangrekar MM, Pant D. Moving towards practical applications of microbial fuel cells for sanitation and resource recovery. J Water Process Eng. 2020; 38 :101566. doi: 10.1016/j.jwpe.2020.101566. [ CrossRef ] [ Google Scholar ]
- Jang JK, Pham TH, Chang IS, et al. Construction and operation of a novel mediator- and membrane-less microbial fuel cell. Process Biochem. 2004; 39 :1007–1012. doi: 10.1016/S0032-9592(03)00203-6. [ CrossRef ] [ Google Scholar ]
- Janicek A, Fan Y, Liu H. Design of microbial fuel cells for practical application: a review and analysis of scale-up studies. Biofuels. 2014; 5 :79–92. doi: 10.4155/bfs.13.69. [ CrossRef ] [ Google Scholar ]
- Jiang Y, Yang X, Liang P, et al. Microbial fuel cell sensors for water quality early warning systems: fundamentals, signal resolution, optimization and future challenges. Renew Sustain Energy Rev. 2018; 81 :292–305. doi: 10.1016/j.rser.2017.06.099. [ CrossRef ] [ Google Scholar ]
- Kadivarian M, Karamzadeh M. Electrochemical modeling of microbial fuel cells performance at different operating and structural conditions. Bioprocess Biosyst Eng. 2020; 43 :393–401. doi: 10.1007/s00449-019-02235-1. [ PubMed ] [ CrossRef ] [ Google Scholar ]
- Kiely PD, Regan JM, Logan BE. The electric picnic: synergistic requirements for exoelectrogenic microbial communities. Curr Opin Biotechnol. 2011; 22 :378–385. doi: 10.1016/j.copbio.2011.03.003. [ PubMed ] [ CrossRef ] [ Google Scholar ]
- Kim B, Mohan SV, Fapyane D, Chang IS. Controlling voltage reversal in microbial fuel cells. Trends Biotechnol. 2020; 38 :667–678. doi: 10.1016/j.tibtech.2019.12.007. [ PubMed ] [ CrossRef ] [ Google Scholar ]
- Kiran R, Patil SA. Microbial electroactive biofilms. American Chemical Society; 2019. [ Google Scholar ]
- Koch C, Harnisch F, Schröder U, Müller S. Cytometric fingerprints: evaluation of new tools for analyzing microbial community dynamics. Front Microbiol. 2014; 5 :273. doi: 10.3389/fmicb.2014.00273. [ PMC free article ] [ PubMed ] [ CrossRef ] [ Google Scholar ]
- Kouzuma A, Ishii S, Watanabe K. Metagenomic insights into the ecology and physiology of microbes in bioelectrochemical systems. Bioresour Technol. 2018; 255 :302–307. doi: 10.1016/j.biortech.2018.01.125. [ PubMed ] [ CrossRef ] [ Google Scholar ]
- Kracke F, Vassilev I, Krömer JO. Microbial electron transport and energy conservation—the foundation for optimizing bioelectrochemical systems. Front Microbiol. 2015; 6 :1–18. doi: 10.3389/fmicb.2015.00575. [ PMC free article ] [ PubMed ] [ CrossRef ] [ Google Scholar ]
- Kumar GG, Sarathi VGS, Nahm KS. Recent advances and challenges in the anode architecture and their modifications for the applications of microbial fuel cells. Biosens Bioelectron. 2013; 43 :461–475. doi: 10.1016/j.bios.2012.12.048. [ PubMed ] [ CrossRef ] [ Google Scholar ]
- Kumar A, Hsu LHH, Kavanagh P, et al. The ins and outs of microorganism-electrode electron transfer reactions. Nat Rev Chem. 2017; 1 :1–13. doi: 10.1108/10662240210438434. [ CrossRef ] [ Google Scholar ]
- Lacerda CMR, Reardon KF. Environmental proteomics: applications of proteome profiling in environmental microbiology and biotechnology. Brief Funct Genomic Proteomic. 2009; 8 :75–87. doi: 10.1093/bfgp/elp005. [ PubMed ] [ CrossRef ] [ Google Scholar ]
- Larrosa A, Lozano LJ, Katuri KP, et al. On the repeatability and reproducibility of experimental two-chambered microbial fuel cells. Fuel. 2009; 88 :1852–1857. doi: 10.1016/j.fuel.2009.04.026. [ CrossRef ] [ Google Scholar ]
- Larsen TA, Hoffmann S, Lüthi C, et al. Emerging solutions to the water challenges of an urbanizing world. Science. 2016; 352 :928–933. doi: 10.1017/CBO9781107415324.004. [ PubMed ] [ CrossRef ] [ Google Scholar ]
- Lefebvre O, Uzabiaga A, Chang IS, et al. Microbial fuel cells for energy self-sufficient domestic wastewater treatment-a review and discussion from energetic consideration. Appl Microbiol Biotechnol. 2011; 89 :259–270. doi: 10.1007/s00253-010-2881-z. [ PubMed ] [ CrossRef ] [ Google Scholar ]
- Leong JX, Daud WRW, Ghasemi M, et al. Ion exchange membranes as separators in microbial fuel cells for bioenergy conversion: a comprehensive review. Renew Sustain Energy Rev. 2013; 28 :575–587. doi: 10.1016/j.rser.2013.08.052. [ CrossRef ] [ Google Scholar ]
- Li M, Zhou M, Tian X, et al. Microbial fuel cell (MFC) power performance improvement through enhanced microbial electrogenicity. Biotechnol Adv. 2018; 36 :1316–1327. doi: 10.1016/j.biotechadv.2018.04.010. [ PubMed ] [ CrossRef ] [ Google Scholar ]
- Li YC, Melenbrink EL, Cordonier GJ, et al. An easily fabricated low-cost potentiostat coupled with user-friendly software for introducing students to electrochemical reactions and electroanalytical techniques. J Chem Educ. 2018; 95 :1658–1661. doi: 10.1021/acs.jchemed.8b00340. [ CrossRef ] [ Google Scholar ]
- Li T, Li R, Zhou Q. The application and progress of bioelectrochemical systems (BESs) in soil remediation: a review. Green Energy Environ. 2020 doi: 10.1016/j.gee.2020.06.026. [ CrossRef ] [ Google Scholar ]
- Liu H, Logan BE. Electricity generation using an air-cathode single chamber microbial fuel cell in the presence and absence of a proton exchange membrane. Environ Sci Technol. 2004; 38 :4040–4046. doi: 10.1021/es0499344. [ PubMed ] [ CrossRef ] [ Google Scholar ]
- Liu Y, Zhang X, Zhang Q, Li C. Microbial fuel cells: nanomaterials based on anode and their application. Energy Technol. 2020 doi: 10.1002/ente.202000206. [ CrossRef ] [ Google Scholar ]
- Logan BE. Microbial fuel cells. New Jersey: John Wiley & Sons Inc; 2008. [ Google Scholar ]
- Logan BE. Exoelectrogenic bacteria that power microbial fuel cells. Nat Rev Microbiol. 2009; 7 :375–381. doi: 10.1038/nrmicro2113. [ PubMed ] [ CrossRef ] [ Google Scholar ]
- Logan BE. Scaling up microbial fuel cells and other bioelectrochemical systems. Appl Microbiol Biotechnol. 2010; 85 :1665–1671. doi: 10.1007/s00253-009-2378-9. [ PubMed ] [ CrossRef ] [ Google Scholar ]
- Logan BE. Essential data and techniques for conducting microbial fuel cell and other types of bioelectrochemical system experiments. Chemsuschem. 2012; 5 :988–994. doi: 10.1002/cssc.201100604. [ PubMed ] [ CrossRef ] [ Google Scholar ]
- Logan BE, Hamelers B, Rozendal R, et al. Microbial fuel cells: methodology and technology. Environ Sci Technol. 2006; 40 :5181–5192. doi: 10.1021/es0605016. [ PubMed ] [ CrossRef ] [ Google Scholar ]
- Logan BE, Cheng S, Watson V, Estadt G. Graphite fiber brush anodes for increased power production in air-cathode microbial fuel cells. Environ Sci Technol. 2007; 41 :3341–3346. doi: 10.1021/es062644y. [ PubMed ] [ CrossRef ] [ Google Scholar ]
- Logan BE, Wallack MJ, Kim K, et al. Assessment of microbial fuel cell configurations and power densities. Environ Sci Technol Lett. 2015; 2 :206–214. doi: 10.1021/acs.estlett.5b00180. [ CrossRef ] [ Google Scholar ]
- Logan BE, Rossi R, Ragab A, Saikaly PE. Electroactive microorganisms in bioelectrochemical systems. Nat Rev Microbiol. 2019; 17 :307–319. doi: 10.1038/s41579-019-0173-x. [ PubMed ] [ CrossRef ] [ Google Scholar ]
- Lovley DR. Powering microbes with electricity: direct electron transfer from electrodes to microbes. Environ Microbiol Rep. 2011; 3 :27–35. doi: 10.1111/j.1758-2229.2010.00211.x. [ PubMed ] [ CrossRef ] [ Google Scholar ]
- Lovley DR. Electromicrobiology. Annu Rev Microbiol. 2012; 66 :391–409. doi: 10.1146/annurev-micro-092611-150104. [ PubMed ] [ CrossRef ] [ Google Scholar ]
- Lovley DR. Happy together: microbial communities that hook up to swap electrons. ISME J. 2017; 11 :327–336. doi: 10.1038/ismej.2016.136. [ PMC free article ] [ PubMed ] [ CrossRef ] [ Google Scholar ]
- Ma F, Yin Y, Pang S, et al. A data-driven based framework of model optimization and neural network modeling for microbial fuel cells. IEEE Access. 2019; 7 :162036–162049. doi: 10.1109/ACCESS.2019.2951943. [ CrossRef ] [ Google Scholar ]
- Martinez CM, Alvarez LH. Application of redox mediators in bioelectrochemical systems. Biotechnol Adv. 2018; 36 :1412–1423. doi: 10.1016/j.biotechadv.2018.05.005. [ PubMed ] [ CrossRef ] [ Google Scholar ]
- Massoud MA, Tarhini A, Nasr JA. Decentralized approaches to wastewater treatment and management: applicability in developing countries. J Environ Manage. 2009; 90 :652–659. doi: 10.1016/j.jenvman.2008.07.001. [ PubMed ] [ CrossRef ] [ Google Scholar ]
- Mathuriya AS, Pant D. Assessment of expanded polystyrene as a separator in microbial fuel cell. Environ Technol (United Kingdom) 2019; 40 :2052–2061. doi: 10.1080/09593330.2018.1435740. [ PubMed ] [ CrossRef ] [ Google Scholar ]
- Md Khudzari J, Kurian J, Tartakovsky B, Raghavan GSV. Bibliometric analysis of global research trends on microbial fuel cells using Scopus database. Biochem Eng J. 2018; 136 :51–60. doi: 10.1016/j.bej.2018.05.002. [ CrossRef ] [ Google Scholar ]
- Mehta S, Jha S, Liang H. Lignocellulose materials for supercapacitor and battery electrodes: a review. Renew Sustain Energy Rev. 2020; 134 :110345. doi: 10.1016/j.rser.2020.110345. [ CrossRef ] [ Google Scholar ]
- Mekuto L, Olowolafe AVA, Pandit S, et al. Microalgae as a biocathode and feedstock in anode chamber for a self-sustainable microbial fuel cell technology: a review. S Afr J Chem Eng. 2020; 31 :7–16. doi: 10.1016/j.sajce.2019.10.002. [ CrossRef ] [ Google Scholar ]
- Meloni GN. Building a microcontroller based potentiostat: a inexpensive and versatile platform for teaching electrochemistry and instrumentation. J Chem Educ. 2016; 93 :1320–1322. doi: 10.1021/acs.jchemed.5b00961. [ CrossRef ] [ Google Scholar ]
- Min B, Logan BE. Continuous electricity generation from domestic wastewater and organic substrates in a flat plate microbial fuel cell. Environ Sci Technol. 2004; 38 :5809–5814. doi: 10.1021/es0491026. [ PubMed ] [ CrossRef ] [ Google Scholar ]
- Mohr SH, Wang J, Ellem G, et al. Projection of world fossil fuels by country. Fuel. 2015; 141 :120–135. doi: 10.1016/j.fuel.2014.10.030. [ CrossRef ] [ Google Scholar ]
- Moran MA, Satinsky B, Gifford SM, et al. Sizing up metatranscriptomics. ISME J. 2013; 7 :237–243. doi: 10.1038/ismej.2012.94. [ PMC free article ] [ PubMed ] [ CrossRef ] [ Google Scholar ]
- Oh ST, Kim JR, Premier GC, et al. Sustainable wastewater treatment: how might microbial fuel cells contribute. Biotechnol Adv. 2010; 28 :871–881. doi: 10.1016/j.biotechadv.2010.07.008. [ PubMed ] [ CrossRef ] [ Google Scholar ]
- Oliveira VB, Simões M, Melo LF, Pinto AMFR. Overview on the developments of microbial fuel cells. Biochem Eng J. 2013; 73 :53–64. doi: 10.1016/j.bej.2013.01.012. [ CrossRef ] [ Google Scholar ]
- Pandey P, Shinde VN, Deopurkar RL, et al. Recent advances in the use of different substrates in microbial fuel cells toward wastewater treatment and simultaneous energy recovery. Appl Energy. 2016; 168 :706–723. doi: 10.1016/j.apenergy.2016.01.056. [ CrossRef ] [ Google Scholar ]
- Pant D, Van Bogaert G, De Smet M, et al. Use of novel permeable membrane and air cathodes in acetate microbial fuel cells. Electrochim Acta. 2010; 55 :7710–7716. doi: 10.1016/j.electacta.2009.11.086. [ CrossRef ] [ Google Scholar ]
- Pant D, Van Bogaert G, Diels L, Vanbroekhoven K. A review of the substrates used in microbial fuel cells (MFCs) for sustainable energy production. Bioresour Technol. 2010; 101 :1533–1543. doi: 10.1016/j.biortech.2009.10.017. [ PubMed ] [ CrossRef ] [ Google Scholar ]
- Pant D, Singh A, Van Bogaert G, et al. Bioelectrochemical systems (BES) for sustainable energy production and product recovery from organic wastes and industrial wastewaters. RSC Adv. 2012; 2 :1248–1263. doi: 10.1039/c1ra00839k. [ CrossRef ] [ Google Scholar ]
- Parkhey P, Sahu R. Microfluidic microbial fuel cells: recent advancements and future prospects. Int J Hydrogen Energy. 2020 doi: 10.1016/j.ijhydene.2020.07.019. [ CrossRef ] [ Google Scholar ]
- Pasternak G, Yang Y, Santos BB, et al. Regenerated silk fibroin membranes as separators for transparent microbial fuel cells. Bioelectrochemistry. 2019; 126 :146–155. doi: 10.1016/j.bioelechem.2018.12.004. [ PubMed ] [ CrossRef ] [ Google Scholar ]
- Pasternak G, Askitosari TD, Rosenbaum MA. Biosurfactants and synthetic surfactants in bioelectrochemical systems: a mini-review. Front Microbiol. 2020; 11 :1–9. doi: 10.3389/fmicb.2020.00358. [ PMC free article ] [ PubMed ] [ CrossRef ] [ Google Scholar ]
- Pasupuleti SB, Srikanth S, Dominguez-Benetton X, et al. Dual gas diffusion cathode design for microbial fuel cell (MFC): optimizing the suitable mode of operation in terms of bioelectrochemical and bioelectro-kinetic evaluation. J Chem Technol Biotechnol. 2016; 91 :624–639. doi: 10.1002/jctb.4613. [ CrossRef ] [ Google Scholar ]
- Peera SG, Maiyalagan T, Liu C, et al. A review on carbon and non-precious metal based cathode catalysts in microbial fuel cells. Int J Hydrogen Energy. 2020 doi: 10.1016/j.ijhydene.2020.07.252. [ CrossRef ] [ Google Scholar ]
- Pham TH, Jang JK, Moon HS, et al. Improved performance of microbial fuel cell using membrane-electrode assembly. J Microbiol Biotechnol. 2005; 15 :438–441. [ Google Scholar ]
- Potter MC. Electrical effects accompanying the decomposition of organic compounds. Proc R Soc B Biol Sci. 1911; 84 :260–276. doi: 10.1098/rspb.1911.0073. [ CrossRef ] [ Google Scholar ]
- Qadir M, Wichelns D, Raschid-Sally L, et al. The challenges of wastewater irrigation in developing countries. Agric Water Manag. 2010; 97 :561–568. doi: 10.1016/j.agwat.2008.11.004. [ CrossRef ] [ Google Scholar ]
- Rabaey K, Keller J. Microbial fuel cell cathodes: from bottleneck to prime opportunity? Water Sci Technol. 2008; 57 :655–659. doi: 10.2166/wst.2008.103. [ PubMed ] [ CrossRef ] [ Google Scholar ]
- Rabaey K, Rozendal RA. Microbial electrosynthesis—revisiting the electrical route for microbial production. Nat Rev Microbiol. 2010; 8 :706–716. doi: 10.1038/nrmicro2422. [ PubMed ] [ CrossRef ] [ Google Scholar ]
- Rabaey K, Clauwaert P, Aelterman P, Verstraete W. Tubular microbial fuel cells for efficient electricity generation. Environ Sci Technol. 2005; 39 :8077–8082. doi: 10.1021/es050986i. [ PubMed ] [ CrossRef ] [ Google Scholar ]
- Rahimnejad M, Bakeri G, Ghasemi M, Zirepour A. A review on the role of proton exchange membrane on the performance of microbial fuel cell. Polym Adv Technol. 2014; 25 :1426–1432. doi: 10.1002/pat.3383. [ CrossRef ] [ Google Scholar ]
- Reguera G. Microbial nanowires and electroactive biofilms. FEMS Microbiol Ecol. 2018; 94 :1–13. doi: 10.1093/femsec/fiy086. [ PubMed ] [ CrossRef ] [ Google Scholar ]
- Rimboud M, Pocaznoi D, Erable B, Bergel A. Electroanalysis of microbial anodes for bioelectrochemical systems: Basics, progress and perspectives. Phys Chem Chem Phys. 2014; 16 :16349–16366. doi: 10.1039/c4cp01698j. [ PubMed ] [ CrossRef ] [ Google Scholar ]
- Rittmann BE. Opportunities for renewable bioenergy using microorganisms. Biotechnol Bioeng. 2008; 100 :203–212. doi: 10.1002/bit.21875. [ PubMed ] [ CrossRef ] [ Google Scholar ]
- Rittmann BE, Krajmalnik-Brown R, Halden RU. Pre-genomic, genomic and post-genomic study of microbial communities involved in bioenergy. Nat Rev Microbiol. 2008; 6 :604–612. doi: 10.1038/nrmicro1939. [ PubMed ] [ CrossRef ] [ Google Scholar ]
- Roy S, Schievano A, Pant D. Electro-stimulated microbial factory for value added product synthesis. Bioresour Technol. 2015; 213 :129–139. doi: 10.1016/j.biortech.2016.03.052. [ PubMed ] [ CrossRef ] [ Google Scholar ]
- Sánchez C, Dessì P, Duffy M, Lens PNL. Microbial electrochemical technologies: electronic circuitry and characterization tools. Biosens Bioelectron. 2020; 150 :111884. doi: 10.1016/j.bios.2019.111884. [ PubMed ] [ CrossRef ] [ Google Scholar ]
- Santoro C, Arbizzani C, Erable B, Ieropoulos I. Microbial fuel cells: From fundamentals to applications. a review. J Power Sources. 2017; 356 :225–244. doi: 10.1016/j.jpowsour.2017.03.109. [ PMC free article ] [ PubMed ] [ CrossRef ] [ Google Scholar ]
- Santoro C, Garcia MJS, Walter XA, et al. Urine in bioelectrochemical systems: an overall review. ChemElectroChem. 2020; 7 :1312–1331. doi: 10.1002/celc.201901995. [ PMC free article ] [ PubMed ] [ CrossRef ] [ Google Scholar ]
- Schröder U. Anodic electron transfer mechanisms in microbial fuel cells and their energy efficiency. Phys Chem Chem Phys. 2007; 9 :2619–2629. doi: 10.1039/b703627m. [ PubMed ] [ CrossRef ] [ Google Scholar ]
- Schröder U. Discover the possibilities: microbial bioelectrochemical systems and the revival of a 100-year–old discovery. J Solid State Electrochem. 2011; 15 :1481–1486. doi: 10.1007/s10008-011-1395-7. [ CrossRef ] [ Google Scholar ]
- Schröder U. A basic introduction into microbial fuel cells and microbial electrocatalysis. ChemTexts. 2018; 4 :19. doi: 10.1007/s40828-018-0072-1. [ CrossRef ] [ Google Scholar ]
- Schröder U, Harnisch F. Life electric—nature as a blueprint for the development of microbial electrochemical technologies. Joule. 2017; 1 :244–252. doi: 10.1016/j.joule.2017.07.010. [ CrossRef ] [ Google Scholar ]
- Schröder U, Harnisch F, Angenent LT. Microbial electrochemistry and technology: terminology and classification. Energy Environ Sci. 2015; 8 :513–519. doi: 10.1039/C4EE03359K. [ CrossRef ] [ Google Scholar ]
- Segata N, Boernigen D, Tickle TL, et al. Computational meta’omics for microbial community studies. Mol Syst Biol. 2013; 9 :666. doi: 10.1038/msb.2013.22. [ PMC free article ] [ PubMed ] [ CrossRef ] [ Google Scholar ]
- Sevda S, Sreekishnan TR, Pous N, et al. Bioelectroremediation of perchlorate and nitrate contaminated water: a review. Bioresour Technol. 2018; 255 :331–339. doi: 10.1016/j.biortech.2018.02.005. [ PubMed ] [ CrossRef ] [ Google Scholar ]
- Sevda S, Garlapati VK, Naha S, et al. Biosensing capabilities of bioelectrochemical systems towards sustainable water streams: technological implications and future prospects. J Biosci Bioeng. 2020; 129 :647–656. doi: 10.1016/j.jbiosc.2020.01.003. [ PubMed ] [ CrossRef ] [ Google Scholar ]
- Sharma M, Bajracharya S, Gildemyn S, et al. A critical revisit of the key parameters used to describe microbial electrochemical systems. Electrochim Acta. 2014; 140 :191–208. doi: 10.1016/j.electacta.2014.02.111. [ CrossRef ] [ Google Scholar ]
- Sleutels THJA, Ter Heijne A, Buisman CJN, Hamelers HVM. Bioelectrochemical systems: an outlook for practical applications. Chemsuschem. 2012; 5 :1012–1019. doi: 10.1002/cssc.201100732. [ PubMed ] [ CrossRef ] [ Google Scholar ]
- Soavi F, Santoro C. Supercapacitive operational mode in microbial fuel cell. Curr Opin Electrochem. 2020; 22 :1–8. doi: 10.1016/j.coelec.2020.03.009. [ CrossRef ] [ Google Scholar ]
- Song H-L, Zhu Y, Li J. Electron transfer mechanisms, characteristics and applications of biological cathode microbial fuel cells—a mini review. Arab J Chem. 2019; 12 :2236–2243. doi: 10.1016/j.arabjc.2015.01.008. [ CrossRef ] [ Google Scholar ]
- Stams AJM, de Bok FAM, Plugge CM, et al. Exocellular electron transfer in anaerobic microbial communities. Environ Microbiol. 2006; 8 :371–382. doi: 10.1111/j.1462-2920.2006.00989.x. [ PubMed ] [ CrossRef ] [ Google Scholar ]
- Sun JZ, Kingori GP, Si RW, et al. Microbial fuel cell-based biosensors for environmental monitoring: a review. Water Sci Technol. 2015; 71 :801–809. doi: 10.2166/wst.2015.035. [ PubMed ] [ CrossRef ] [ Google Scholar ]
- Sun M, Zhai L-F, Li W-W, Yu H-Q. Harvest and utilization of chemical energy in wastes by microbial fuel cells. Chem Soc Rev. 2016; 45 :2847–2870. doi: 10.1039/C5CS00903K. [ PubMed ] [ CrossRef ] [ Google Scholar ]
- ter Heijne A, Schaetzle O, Gimenez S, et al. Analysis of bio-anode performance through electrochemical impedance spectroscopy. Bioelectrochemistry. 2015; 106 :64–72. doi: 10.1016/j.bioelechem.2015.04.002. [ PubMed ] [ CrossRef ] [ Google Scholar ]
- Tiquia-Arashiro SM, Pant D. Microbial electrochemical technologies. CRC Press; 2020. [ Google Scholar ]
- Torres CI. On the importance of identifying, characterizing, and predicting fundamental phenomena towards microbial electrochemistry applications. Curr Opin Biotechnol. 2014; 27 :107–114. doi: 10.1016/j.copbio.2013.12.008. [ PubMed ] [ CrossRef ] [ Google Scholar ]
- Toze S. Reuse of effluent water—benefits and risks. Agric Water Manag. 2006; 80 :147–159. doi: 10.1016/j.agwat.2005.07.010. [ CrossRef ] [ Google Scholar ]
- UN DESA Population Division (2019) World Population Prospects 2019: Ten Key Findings
- Venkata Mohan S, Pandey A, Varjani S. Microbial electrochemical technology: sustainable platform for fuels, chemicals and remediation. Elsevier; 2018. [ Google Scholar ]
- Villano M, Aulenta F, Majone M. Perspectives of biofuels production from renewable resources with bioelectrochemical systems. Asia-Pacific J Chem Eng. 2012; 7 :S263–S274. doi: 10.1002/apj.1643. [ CrossRef ] [ Google Scholar ]
- Virdis B, Millo D, Donose BC, et al. Analysis of electron transfer dynamics in mixed community electroactive microbial biofilms. RSC Adv. 2016; 6 :3650–3660. doi: 10.1039/c5ra15676a. [ CrossRef ] [ Google Scholar ]
- Wagner RC, Call DF, Logan BE. Optimal set anode potentials vary in bioelectrochemical systems. Environ Sci Technol. 2010; 44 :6036–6041. doi: 10.1021/es101013e. [ PubMed ] [ CrossRef ] [ Google Scholar ]
- Wang Z, He Z. Demystifying terms for understanding bioelectrochemical systems towards sustainable wastewater treatment. Curr Opin Electrochem. 2020; 19 :14–19. doi: 10.1016/j.coelec.2019.09.001. [ CrossRef ] [ Google Scholar ]
- Wang H-Y, Bernarda A, Huang C-Y, et al. Micro-sized microbial fuel cell: a mini-review. Bioresour Technol. 2011; 102 :235–243. doi: 10.1016/j.biortech.2010.07.007. [ PubMed ] [ CrossRef ] [ Google Scholar ]
- Wang H, Park J, Ren ZJ. Practical energy harvesting for microbial fuel cells: a review. Environ Sci Technol. 2015; 49 :3267–3277. doi: 10.1021/es5047765. [ PubMed ] [ CrossRef ] [ Google Scholar ]
- Wang W, Zhang Y, Li M, et al. Operation mechanism of constructed wetland-microbial fuel cells for wastewater treatment and electricity generation: a review. Bioresour Technol. 2020; 314 :123808. doi: 10.1016/j.biortech.2020.123808. [ PubMed ] [ CrossRef ] [ Google Scholar ]
- Wang X, Aulenta F, Puig S, et al. Microbial electrochemistry for bioremediation. Environ Sci Ecotechnol. 2020; 1 :100013. doi: 10.1016/j.ese.2020.100013. [ PMC free article ] [ PubMed ] [ CrossRef ] [ Google Scholar ]
- Watson VJ, Logan BE. Analysis of polarization methods for elimination of power overshoot in microbial fuel cells. Electrochem commun. 2011; 13 :54–56. doi: 10.1016/j.elecom.2010.11.011. [ CrossRef ] [ Google Scholar ]
- Wei J, Liang P, Huang X. Recent progress in electrodes for microbial fuel cells. Bioresour Technol. 2011; 102 :9335–9344. doi: 10.1016/j.biortech.2011.07.019. [ PubMed ] [ CrossRef ] [ Google Scholar ]
- Winfield J, Ieropoulos I, Greenman J, Dennis J. The overshoot phenomenon as a function of internal resistance in microbial fuel cells. Bioelectrochemistry. 2011; 81 :22–27. doi: 10.1016/j.bioelechem.2011.01.001. [ PubMed ] [ CrossRef ] [ Google Scholar ]
- Winfield J, Gajda I, Greenman J, Ieropoulos I. A review into the use of ceramics in microbial fuel cells. Bioresour Technol. 2016; 215 :296–303. doi: 10.1016/j.biortech.2016.03.135. [ PubMed ] [ CrossRef ] [ Google Scholar ]
- Xia C, Zhang D, Pedrycz W, et al. Models for microbial fuel cells: a critical review. J Power Sources. 2018; 373 :119–131. doi: 10.1016/j.jpowsour.2017.11.001. [ CrossRef ] [ Google Scholar ]
- Yang Y, Xu M, Guo J, Sun G. Bacterial extracellular electron transfer in bioelectrochemical systems. Process Biochem. 2012; 47 :1707–1714. doi: 10.1016/j.procbio.2012.07.032. [ CrossRef ] [ Google Scholar ]
- Yang E, Chae KJ, Kim IS. Assessment of different ceramic filtration membranes as a separator in microbial fuel cells. Desalin Water Treat. 2016; 57 :28077–28085. doi: 10.1080/19443994.2016.1183523. [ CrossRef ] [ Google Scholar ]
- Yang Y, Ye D, Li J, et al. Microfluidic microbial fuel cells: From membrane to membrane free. J Power Sources. 2016; 324 :113–125. doi: 10.1016/j.jpowsour.2016.05.078. [ CrossRef ] [ Google Scholar ]
- Yang E, Chae KJ, Choi MJ, et al. Critical review of bioelectrochemical systems integrated with membrane-based technologies for desalination, energy self-sufficiency, and high-efficiency water and wastewater treatment. Desalination. 2019; 452 :40–67. doi: 10.1016/j.desal.2018.11.007. [ CrossRef ] [ Google Scholar ]
- Yates M, Strycharz-Glaven S, Golden J, et al. Characterizing electron transport through living biofilms. J Vis Exp. 2018; 2018 :1–8. doi: 10.3791/54671. [ PMC free article ] [ PubMed ] [ CrossRef ] [ Google Scholar ]
- Yuan H, Hou Y, Abu-Reesh IM, et al. Oxygen reduction reaction catalysts used in microbial fuel cells for energy-efficient wastewater treatment: a review. Mater Horizons. 2016; 3 :382–401. doi: 10.1039/c6mh00093b. [ CrossRef ] [ Google Scholar ]
- Zhang PY, Liu ZL. Experimental study of the microbial fuel cell internal resistance. J Power Sources. 2010; 195 :8013–8018. doi: 10.1016/j.jpowsour.2010.06.062. [ CrossRef ] [ Google Scholar ]
- Zhang F, Cheng S, Pant D, et al. Power generation using an activated carbon and metal mesh cathode in a microbial fuel cell. Electrochem commun. 2009; 11 :2177–2179. doi: 10.1016/j.elecom.2009.09.024. [ CrossRef ] [ Google Scholar ]
- Zhang X, Li X, Zhao X, Li Y. Factors affecting the efficiency of a bioelectrochemical system: a review. RSC Adv. 2019; 9 :19748–19761. doi: 10.1039/c9ra03605a. [ PMC free article ] [ PubMed ] [ CrossRef ] [ Google Scholar ]
- Zhang Y, Liu M, Zhou M, et al. Microbial fuel cell hybrid systems for wastewater treatment and bioenergy production: Synergistic effects, mechanisms and challenges. Renew Sustain Energy Rev. 2019; 103 :13–29. doi: 10.1016/j.rser.2018.12.027. [ CrossRef ] [ Google Scholar ]
- Zhao F, Slade RCT, Varcoe JR. Techniques for the study and development of microbial fuel cells: an electrochemical perspective. Chem Soc Rev. 2009; 38 :1926–1939. doi: 10.1039/b819866g. [ PubMed ] [ CrossRef ] [ Google Scholar ]
- Zheng T, Li J, Ji Y, et al. Progress and prospects of bioelectrochemical systems: electron transfer and its applications in the microbial metabolism. Front Bioeng Biotechnol. 2020; 8 :1–10. doi: 10.3389/fbioe.2020.00010. [ PMC free article ] [ PubMed ] [ CrossRef ] [ Google Scholar ]
- Zhi W, Ge Z, He Z, Zhang H. Methods for understanding microbial community structures and functions in microbial fuel cells: a review. Bioresour Technol. 2014; 171 :461–468. doi: 10.1016/j.biortech.2014.08.096. [ PubMed ] [ CrossRef ] [ Google Scholar ]
- Zhu X, Tokash JC, Hong Y, Logan BE. Controlling the occurrence of power overshoot by adapting microbial fuel cells to high anode potentials. Bioelectrochemistry. 2013; 90 :30–35. doi: 10.1016/j.bioelechem.2012.10.004. [ PubMed ] [ CrossRef ] [ Google Scholar ]
- Zou S, He Z. Efficiently “pumping out” value-added resources from wastewater by bioelectrochemical systems: a review from energy perspectives. Water Res. 2018; 131 :62–73. doi: 10.1016/j.watres.2017.12.026. [ PubMed ] [ CrossRef ] [ Google Scholar ]
Vehicle Technologies and Hydrogen and Fuel Cell Technologies Research and Development Programs Benefits Assessment Report for 2020
- Center for Integrated Mobility Sciences
- Strategic Energy Analysis Center
Research output : NREL › Technical Report
NREL Publication Number
- NREL/TP-5400-79617
- benefits analysis
- choice modeling
- electric vehicles
- electrification
- hybrid vehicles
- plug-in hybrid vehicles
- vehicle technologies
Access to Document
- 10.2172/1818458
- https://www.nrel.gov/docs/fy21osti/79617.pdf
Fingerprint
- Research and Development Earth and Planetary Sciences 100%
- Report Earth and Planetary Sciences 100%
- Assessment Earth and Planetary Sciences 100%
- Program Earth and Planetary Sciences 100%
- Vehicle Earth and Planetary Sciences 100%
- Technology Earth and Planetary Sciences 100%
- Hydrogen Earth and Planetary Sciences 100%
- Fuel Cell Earth and Planetary Sciences 100%
T1 - Vehicle Technologies and Hydrogen and Fuel Cell Technologies Research and Development Programs Benefits Assessment Report for 2020
AU - Brooker, Aaron
AU - Birky, Alicia
AU - Reznicek, Evan
AU - Gonder, Jeff
AU - Hunter, Chad
AU - Lustbader, Jason
AU - Zhang, Chen
AU - Sittler, Lauren
AU - Yip, Arthur
AU - Yang, Fan
AU - Lee, Dong-Yeon
N2 - The U.S. Department of Energy’s Vehicle Technologies and Hydrogen and Fuel Cell Technologies Offices (VTO and HFTO) support research and development of efficient and sustainable transportation technologies that will improve energy efficiency, minimize emissions, and enable America to use less petroleum. VTO and HFTO regularly revisit and update relevant research and development goals and areas of emphasis in response to the latest technological advancements and in alignment with current national priorities. As such, analyses of expected benefits resulting from VTO and HFTO investments and anticipated goal achievements are updated periodically and will be again for 2021 in the context of the latest national-level transportation decarbonization goals. The analysis in the present report is based on technical progress goals established in VTO and HFTO in the years immediately prior to and including 2020, and it summarizes the estimated energy and emissions benefits corresponding to achievement of those goals. The goals span research activities on batteries, electric drive technologies (EDT), combustion, lightweight materials, fuel cells, and hydrogen storage. The evaluation includes detailed analyses into the benefits of technology improvements on the U.S. light-duty (LD) vehicle fleet and separately on the U.S. medium- and heavy-duty (MDHD) vehicle fleet. This report summarizes the outcomes from each of these analyses both independently and in combination.
AB - The U.S. Department of Energy’s Vehicle Technologies and Hydrogen and Fuel Cell Technologies Offices (VTO and HFTO) support research and development of efficient and sustainable transportation technologies that will improve energy efficiency, minimize emissions, and enable America to use less petroleum. VTO and HFTO regularly revisit and update relevant research and development goals and areas of emphasis in response to the latest technological advancements and in alignment with current national priorities. As such, analyses of expected benefits resulting from VTO and HFTO investments and anticipated goal achievements are updated periodically and will be again for 2021 in the context of the latest national-level transportation decarbonization goals. The analysis in the present report is based on technical progress goals established in VTO and HFTO in the years immediately prior to and including 2020, and it summarizes the estimated energy and emissions benefits corresponding to achievement of those goals. The goals span research activities on batteries, electric drive technologies (EDT), combustion, lightweight materials, fuel cells, and hydrogen storage. The evaluation includes detailed analyses into the benefits of technology improvements on the U.S. light-duty (LD) vehicle fleet and separately on the U.S. medium- and heavy-duty (MDHD) vehicle fleet. This report summarizes the outcomes from each of these analyses both independently and in combination.
KW - benefits analysis
KW - choice modeling
KW - electric vehicles
KW - electrification
KW - fuel cells
KW - hybrid vehicles
KW - hydrogen
KW - investment
KW - plug-in hybrid vehicles
KW - research
KW - vehicle technologies
U2 - 10.2172/1818458
DO - 10.2172/1818458
M3 - Technical Report
Notification: View the latest site access restrictions, updates, and resources related to the coronavirus (COVID-19) »
NREL's research accelerates development, integration, and scale up of hydrogen and fuel cell technologies to enable widespread deployment across multiple energy sectors.
Our work helps industry overcome technical challenges and supports the U.S. Department of Energy's (DOE's) Hydrogen and Fuel Cell Technologies Office and H2@Scale vision for clean hydrogen across multiple applications and economic sectors. We also bridge technologies with other research areas across the lab and through multiple DOE and national lab research initiatives, consortia, and collaborations .
R&D Areas

Hydrogen Production and Delivery
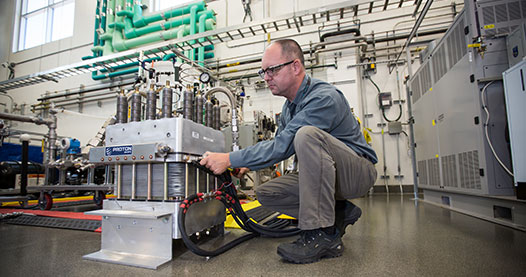
Hydrogen Storage

Manufacturing

Market Transformation

Safety, Codes, and Standards

Systems Analysis

Technology Validation

Related Research Areas
Advanced Manufacturing
Chemistry and Nanoscience
Energy Analysis
Energy Storage
Energy Systems Integration Facility
Transportation and Mobility

Featured Research
Aries: advanced research on integrated energy systems.
Preface for Special Section on Fuel Cell Technology
- Editorial Preface
- Published: 08 May 2021
- Volume 4 , pages 117–118, ( 2021 )
Cite this article
- Cunman Zhang 1
1779 Accesses
2 Citations
Explore all metrics
Avoid common mistakes on your manuscript.
Faced with the resource shortage and environmental problems, the development and utilization of new energy are deemed critical in many countries around the world in order to adapt to the change of energy supply and demand pattern as well as ensure national energy security. As a system that converts chemical energy of fuel oxidation directly into electric energy, fuel cell has shown strong competitiveness in the field of transportation, and is regarded as a new generation of vehicle power source and is favoured by the world, because of its advantages such as high efficiency, environment-friendly, long driving range, high reliability, no vibration and noise, efficiency changing with the output power, strong overload capacity and so on.
Fuel cell technology development has gradually become a research hotspot in the global energy field. A large number of investigations have been carried out on the preparation technology and basic theory of key materials, components and system such as high activity and anti-aggregation electrocatalyst, high specific surface area and anti-oxidation support, high conductivity and self-humidifying electrolyte, high permeability and high stability carbon paper, anti-corrosion and high conductivity bipolar plates, high performance membrane electrode assembly and fuel cell power system. However, fuel cells are still facing a series of challenges, such as high cost, insufficient material performance, electrode design and thermal management technology to be improved, requiring the joint efforts of researchers worldwide to contribute to fuel cells into the practical application as soon as possible.
In this special section, we have gathered 5 papers with significant contributions in the field of fuel cell, which reflects the latest advances in developing and understanding the design and optimization of key materials and components of fuel cells. The papers, as listed below, cover the topics of carbon support evaluation, Pt-free cathode electrocatalyst, cathode catalyst layer design, local behaviours characterization of fuel cell and heat exchanger numerical analysis.
The paper No. 1 “Modifying carbon supports of catalyst for the oxygen reduction reaction in vehicular PEMFCs” provides an efficient method to evaluate PEMFC carbon supports for vehicles. It is helpful to select a suitable carbon support to achieve uniform dispersion of Pt nanoparticles, resist corrosion and reinforce metal-support interactions, which is beneficial to improve the overall fuel cell performance.
The paper No. 2 “Proton exchange membrane (PEM) fuel cells with platinum group metal (PGM)-free cathode” reviews the research progress of PGM-free fuel cell cathode catalysts in recent years, summarizes the main challenges and puts forward the promising strategies to address the challenges. This work is helpful for readers to have a quick and comprehensive understanding of PGM-free cathode catalysts.
The paper No. 3 “Cathode design for proton exchange membrane fuel cells in automotive applications” reviews the effects of cathode design on performance of PEMFCs, presents the measurement to achieve higher power density for automotive application and provides a good reference for readers in this field.
The paper No. 4 “Experimental investigation on local behaviors of PEMFC with segmented cell” investigates on local behaviors of PEMFC with segmented cell, puts forward novel research methods to directly test the internal local RH, current and temperature to analyse co-flow and counter-flow effects of air/H 2 and draws a conclusion with great application value.
The paper No. 5 “Air-side fin geometry of tube-strip heat exchanger for fuel cell vehicles” constructs the typical unit cell of tube-strip heat exchanger to obtain the cell-level thermal performance of heat exchanger for fuel cell vehicles numerically. It provides valuable theoretical support for the design of heat management of fuel cell vehicle systems.
The publication of this special issue would not be achieved without the meticulous work and joint efforts of all the authors, reviewers and editors. We would like to thank all authors, reviewers and the staff from the editorial office of Automotive Innovation for their hard work and support. Heartly hope this special issue can inspire scientific ideas and promote researches in the fields of fuel cell.
Guest Editors
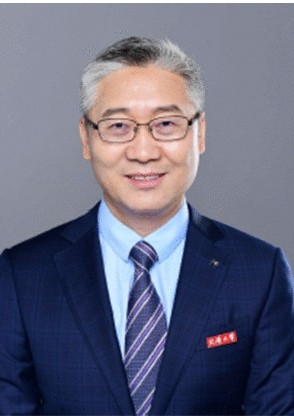
Prof. Cunman Zhang , Professor in the School of Automotive Studies, the Deputy Director of Clean Energy Automotive Engineering Center and the Director of Institute of Fuel Cell Hybrid Power, Tongji University. His current research interests is hydrogen energy and fuel cell technology, including electrolytic hydrogen production technology from renewable energy, high pressure hydrogen storage technology and safety on vehicle, key technology of hydrogen refuelling station and hydrogen safety evaluation system, core technology of high performance and high power fuel cell stack and so on. In recent years, he has published more than 80 high-level academic papers and has been granted over 40 invention patents.
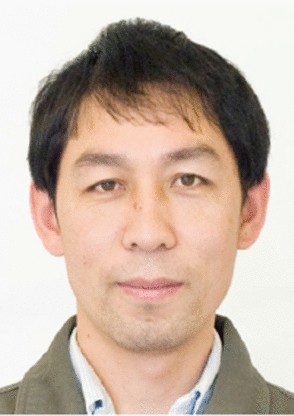
Prof. Shangfeng Du , Associate Professor in Chemical Engineering, leading Low Temperature Fuel Cell and Electrolyser research group at the University of Birmingham. He is a recipient of Marie Curie Incoming International Fellowship and Science City Fellowship. His group is focused on electrochemical engineering research of catalyst electrodes that bridges the gap between the novel catalyst nanostructures and high power performance devices. His research activities cover electrode design, 1D nanostructure development, surface and interface characterization for fuel cells, electrolysers and zinc-air batteries. Research is also carried on the characterization of adhesion and aggregation of nanoparticles for health and energy applications.
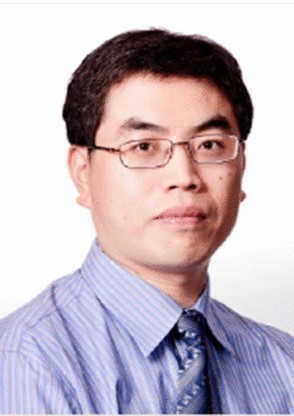
Prof. Shuhui Sun , Full Professor at the Institut National de la Recherche Scientifique (INRS), center for Energy, Materials, and Telecommunications (Montreal, Canada), where he directs the Laboratory of Sustainable Nanotechnology. He is a member of the Royal Society of Canada’s College of New Scholars, and is among the world’s top 2% scientists. His current research interests focus on multi-functional Nanomaterials for Energy Conversion and Storage, including H 2 fuel cells (low-Pt and Pt-free catalysts), Lithium batteries, Zn-air batteries, Na-ion/Zn-ion batteries, CO 2 reduction, water splitting for hydrogen generation, as well as wastewater treatment. He has published over 220 articles in peer-reviewed journals, and his publications have been cited over 12,000 times with an H index of 56. He has edited 3 books, published 14 book chapters and holds 2 US patents.
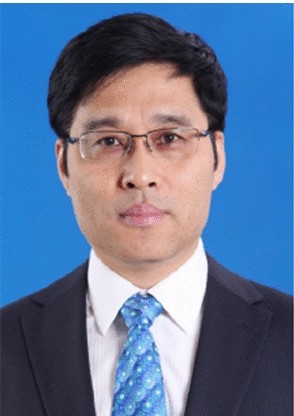
Prof. Hao Tang , Distinguished Professor, Director of Hydrogen and Fuel Cell Research Institute at University of Electronic Science and Technology of China. Dr. Tang has over 26 years of industrial and academic experience in the fundamental research, engineering design and product development in the field of hydrogen and fuel cell. Under his leadership, his R&D team has developed a series of commercial products including the key components of fuel cell, hydrogen power systems, and testing equipment. Dr. Tang has co-authored more than 50 research papers published in referenced journals and holds over 70 national and international patents.
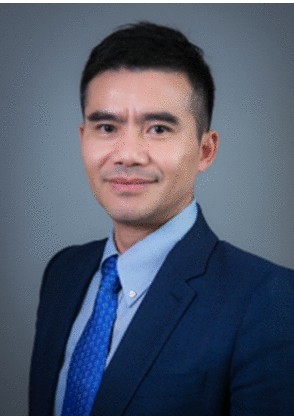
Prof. Junliang Zhang , Zhiyuan Chair Professor, Director of Institute of Fuel Cells, Executive Dean of Zhiyuan College at Shanghai Jiao Tong University (SJTU). From 2007 to 2011, Dr. Zhang worked at General Motors Global Research and Development, Electrochemical Energy Research Laboratory in New York, as a Research Scientist and later a Senior Scientist and Team Leader, responsible for the development of automotive fuel cells. In 2011, he joined the Institute of Fuel Cells at SJTU. Dr. Junliang Zhang’s key expertise is in electrochemistry, electrocatalysis and fuel cells. He has published over 120 peer-reviewed journal articles on electrochemical energy conversion and storage, which have been cited for more than 10,000 times so far. He authored two book, two book chapter and co-invented 50 plus patents.
Author information
Authors and affiliations.
Clean Energy Automotive Engineering Center and School of Automotive Studies, Tongji University, 4800 Caoan Road, Shanghai, 201804, China
Cunman Zhang
You can also search for this author in PubMed Google Scholar
Corresponding author
Correspondence to Cunman Zhang .
Rights and permissions
Reprints and permissions
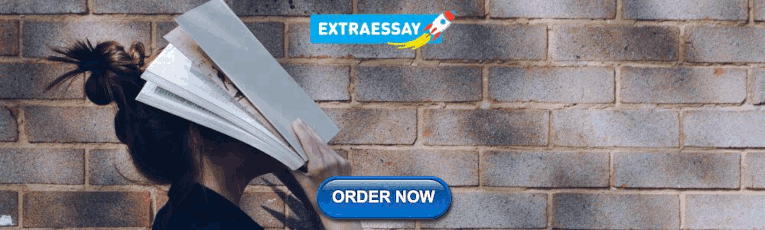
About this article
Zhang, C. Preface for Special Section on Fuel Cell Technology. Automot. Innov. 4 , 117–118 (2021). https://doi.org/10.1007/s42154-021-00150-4
Download citation
Accepted : 07 April 2021
Published : 08 May 2021
Issue Date : May 2021
DOI : https://doi.org/10.1007/s42154-021-00150-4
Share this article
Anyone you share the following link with will be able to read this content:
Sorry, a shareable link is not currently available for this article.
Provided by the Springer Nature SharedIt content-sharing initiative
- Find a journal
- Publish with us
- Track your research
NTRS - NASA Technical Reports Server
Available downloads, related records.
share this!
March 29, 2024
This article has been reviewed according to Science X's editorial process and policies . Editors have highlighted the following attributes while ensuring the content's credibility:
fact-checked
peer-reviewed publication
A high-efficiency photocatalyst for converting carbon dioxide into environmentally friendly energy using sunlight
by DGIST (Daegu Gyeongbuk Institute of Science and Technology)
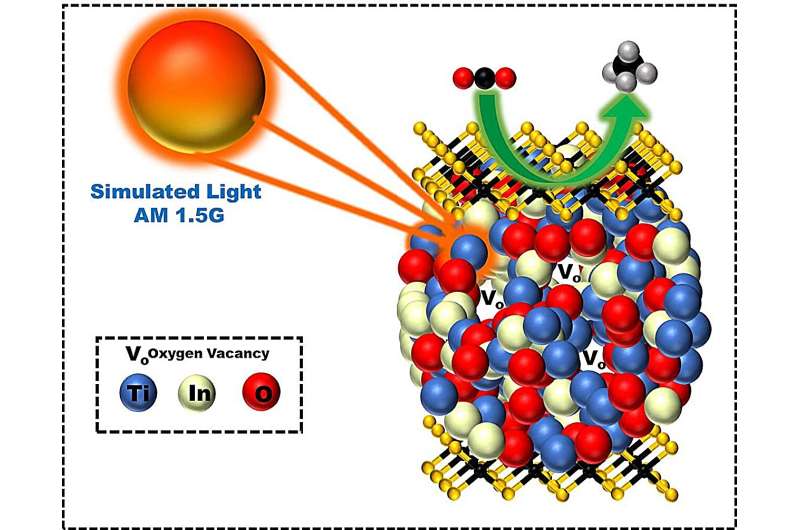
DGIST Professor In Su-il's research team has developed a high-efficiency photocatalyst that utilizes sunlight to convert carbon dioxide (CO 2 ), the primary cause of global warming, into methane (CH 4 ) fuel. The research team expects that this environmentally friendly technology can be applied to Carbon Capture and Utilization (CCU) technology.
According to a US university research team, the current concentration of carbon dioxide in the atmosphere has reached its highest level in 14 million years, at 420 ppm. The World Meteorological Organization (WMO) predicts that 2024 will be a hotter year than last year due to the influence of El Niño.
The World Economic Forum (WEF) has identified climate change as the greatest global risk among 34 crises faced by the world in fields including economics, society, technology, and geopolitics, which could lead to international conflicts as a result of the depletion of resources and polarization. Therefore, reducing the concentration of carbon dioxide in the atmosphere is inevitable to overcome crises induced by climate change.
In this regard, research on photocatalysts, capable of reducing carbon dioxide emissions while simultaneously converting it into useful fuel, has been actively pursued. Photocatalyst research has garnered attention as a promising Carbon Capture and Utilization (CCU) technology for the future, as they rely solely on sunlight without the need for additional energy input, such as electricity, making their systems inherently simple.
However, most photocatalysts developed so far are composed of a crystal structure with regularly arranged atoms. Researchers have, therefore, faced constraints, such as the conditions for the composition to adhere to the arrangement of constituent elements, in designing various active spots within the catalyst while maintaining the structure.
Against this backdrop, Professor In Su-il's research team at DGIST has developed a high-efficiency photocatalyst that includes various active spots and improves electron transfer performance.
The research team fabricated an "amorphous structure of In 2 TiO 5 photocatalyst" containing "Ti 3+ active spots that can adsorb and activate carbon dioxide" and "In 3+ active spots that can decompose water to supply protons," and incorporated it into molybdenum diselenide (MoSe 2 ) nanolayers to improve electron transfer performance.
Through structural analysis , the research team confirmed that the newly developed photocatalyst converts methane 51 times more than the commercially available TiO 2 photocatalysts.
Professor In Su-il of DGIST said, "This research holds significance as it has developed a high-efficiency photocatalyst technology featuring dual active spots. We will conduct follow-up research on improving energy loss and stability of amorphous photocatalysts for future commercialization of the technology."
The research is published in the Chemical Engineering Journal .
Journal information: Chemical Engineering Journal
Provided by DGIST (Daegu Gyeongbuk Institute of Science and Technology)
Explore further
Feedback to editors

A cosmic 'speed camera' just revealed the staggering speed of neutron star jets in a world first
8 hours ago

Saturday Citations: 100-year-old milk, hot qubits and another banger from the Event Horizon Telescope project
11 hours ago

Curiosity rover searches for new clues about Mars' ancient water
14 hours ago

Study says since 1979 climate change has made heat waves last longer, spike hotter, hurt more people

Scientist taps into lobsters' unusual habits to conquer the more than 120-year quest to farm them
Mar 29, 2024

Blind people can hear and feel April's total solar eclipse with new technology

Mapping the best route for a spacecraft traveling beyond the sun's sphere of influence

Researchers outline new approach in search for dark matter through future DUNE research project

Researchers reveal evolutionary path of important proteins

Study identifies protein responsible for gas vesicle clustering in bacteria
Relevant physicsforums posts, zirconium versus zirconium carbide for use with galinstan, electrolysis: dark blue oxide from steel.
Mar 28, 2024
Identification of HOMO/LUMO in radicals
Mar 27, 2024
Quantum hybridized orbitals
New insight into the chemistry of solvents.
Mar 23, 2024
Is G10 material dangerous?
More from Chemistry
Related Stories

Team develops eco-friendly high-efficiency photocatalysts that convert atmospheric carbon dioxide into fuel
Sep 20, 2023

Highly efficient photocatalyst that converts carbon dioxide to methane
Dec 4, 2017

New photocatalyst speeds up the conversion of carbon dioxide into chemical resources
May 29, 2017

One-two-punch catalysts trapping carbon dioxide for cleaner fuels
Jun 7, 2019

A catalyst for converting carbon dioxide, the main cause of global warming, into ethylene using vitamin C

Converting carbon dioxide into methane or ethane selectively
Aug 14, 2018
Recommended for you

Researchers develop a thermoelectric material with optimal cost, efficiency and flexibility

New hydrogel can stretch to 15 times its original size

Storing electrons from hydrogen for clean chemical reactions

Team discovers fundamentally new way to detect radiation involving cheap ceramics

Chemists develop method to confirm mRNA vaccine stability

Study unlocks the power of visible light for sustainable chemistry
Let us know if there is a problem with our content.
Use this form if you have come across a typo, inaccuracy or would like to send an edit request for the content on this page. For general inquiries, please use our contact form . For general feedback, use the public comments section below (please adhere to guidelines ).
Please select the most appropriate category to facilitate processing of your request
Thank you for taking time to provide your feedback to the editors.
Your feedback is important to us. However, we do not guarantee individual replies due to the high volume of messages.
E-mail the story
Your email address is used only to let the recipient know who sent the email. Neither your address nor the recipient's address will be used for any other purpose. The information you enter will appear in your e-mail message and is not retained by Phys.org in any form.
Newsletter sign up
Get weekly and/or daily updates delivered to your inbox. You can unsubscribe at any time and we'll never share your details to third parties.
More information Privacy policy
Donate and enjoy an ad-free experience
We keep our content available to everyone. Consider supporting Science X's mission by getting a premium account.
E-mail newsletter
Suggestions or feedback?
MIT News | Massachusetts Institute of Technology
- Machine learning
- Social justice
- Black holes
- Classes and programs
Departments
- Aeronautics and Astronautics
- Brain and Cognitive Sciences
- Architecture
- Political Science
- Mechanical Engineering
Centers, Labs, & Programs
- Abdul Latif Jameel Poverty Action Lab (J-PAL)
- Picower Institute for Learning and Memory
- Lincoln Laboratory
- School of Architecture + Planning
- School of Engineering
- School of Humanities, Arts, and Social Sciences
- Sloan School of Management
- School of Science
- MIT Schwarzman College of Computing
Second round of seed grants awarded to MIT scholars studying the impact and applications of generative AI
Press contact :.
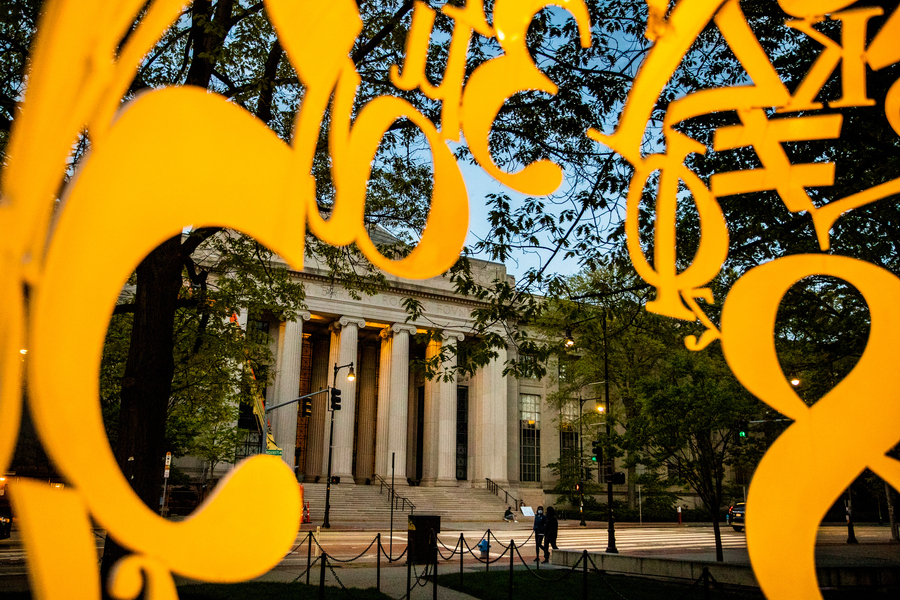
Previous image Next image
Last summer, MIT President Sally Kornbluth and Provost Cynthia Barnhart issued a call for papers to “articulate effective roadmaps, policy recommendations, and calls for action across the broad domain of generative AI.” The response to the call far exceeded expectations with 75 proposals submitted. Of those, 27 proposals were selected for seed funding .
In light of this enthusiastic response, Kornbluth and Barnhart announced a second call for proposals this fall.
“The groundswell of interest and the caliber of the ideas overall made clear that a second round was in order,” they said in their email to MIT’s research community this fall. This second call for proposals resulted in 53 submissions.
Following the second call, the faculty committee from the first round considered the proposals and selected 16 proposals to receive exploratory funding. Co-authored by interdisciplinary teams of faculty and researchers affiliated with all five of the Institute’s schools and the MIT Schwarzman College of Computing, the proposals offer insights and perspectives on the potential impact and applications of generative AI across a broad range of topics and disciplines.
Each selected research group will receive between $50,000 and $70,000 to create 10-page impact papers. Those papers will be shared widely via a publication venue managed and hosted by the MIT Press under the auspices of the MIT Open Publishing Services program.
As with the first round of papers, Thomas Tull, a member of the MIT School of Engineering Dean’s Advisory Council and a former innovation scholar at the School of Engineering, contributed funding to support the effort.
The selected papers are:
- “A Road-map for End-to-end Privacy and Verifiability in Generative AI,” led by Alex Pentland, Srini Devadas, Lalana Kagal, and Vinod Vaikuntanathan;
- “A Virtuous Cycle: Generative AI and Discovery in the Physical Sciences,” led by Philip Harris and Phiala Shanahan;
- “Artificial Cambrian Intelligence: Generating New Forms of Visual Intelligence,” led by Ramesh Raskar and Tomaso A. Poggio;
- “Artificial Fictions and the Value of AI-Generated Art,” led by Justin Khoo;
- “GenAI for Improving Human-to-human Interactions with a Focus on Negotiations,” led by Lawrence Susskind and Samuel Dinnar;
- “Generative AI as a New Applications Platform and Ecosystem,” led by Michael Cusumano;
- “Generative AI for Cities: A Civic Engagement Playbook,” led by Sarah Williams, Sara Beery, and Eden Medina;
- “Generative AI for Textile Engineering: Advanced Materials from Heritage Lace Craft,” led by Svetlana V. Boriskina;
- “Generative AI Impact for Biomedical Innovation and Drug Discovery,” led by Manolis Kellis, Brad Pentelute, and Marinka Zitnik;
- “Impact of Generative AI on the Creative Economy,” led by Ashia Wilson and Dylan Hadfield-Menell;
- “Redefining Virtuosity: The Role of Generative AI in Live Music Performances,” led by Joseph A. Paradiso and Eran Egozy;
- “Reflection-based Learning with Generative AI,” led by Stefanie Mueller;
- “Robust and Reliable Systems for Generative AI,” led by Shafi Goldwasser, Yael Kalai, and Vinod Vaikuntanathan;
- “Supporting the Aging Population with Generative AI,” led by Pattie Maes;
- “The Science of Language in the Era of Generative AI,” led by Danny Fox, Yoon Kim, and Roger Levy; and
- “Visual Artists, Technological Shock, and Generative AI,” led by Caroline Jones and Huma Gupta.
Share this news article on:
Related links.
- President Sally Kornbluth
- Office of the Provost
Related Topics
- Artificial intelligence
- Technology and society
- Technology and policy
- Computer science and technology
- Administration
- School of Architecture and Planning
- Electrical Engineering & Computer Science (eecs)
- School of Humanities Arts and Social Sciences
Related Articles
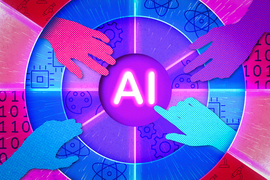
MIT scholars awarded seed grants to probe the social implications of generative AI
Previous item Next item
More MIT News
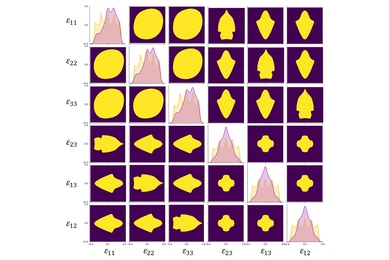
A first-ever complete map for elastic strain engineering
Read full story →
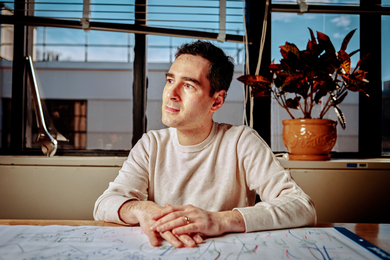
“Life is short, so aim high”
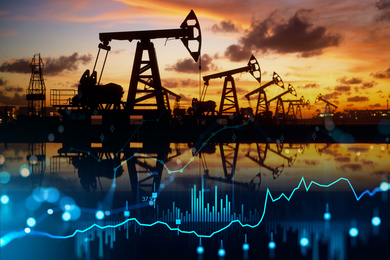
Shining a light on oil fields to make them more sustainable
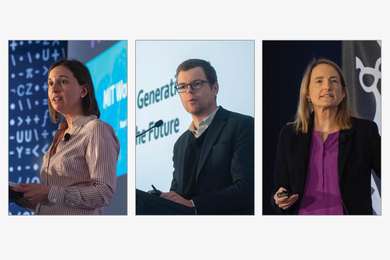
MIT launches Working Group on Generative AI and the Work of the Future
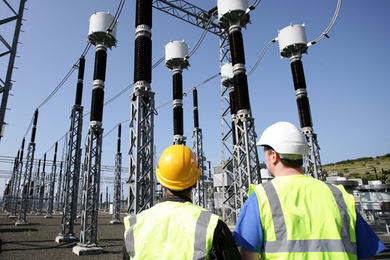
Atmospheric observations in China show rise in emissions of a potent greenhouse gas
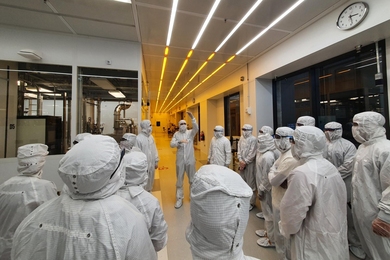
VIAVI Solutions joins MIT.nano Consortium
- More news on MIT News homepage →
Massachusetts Institute of Technology 77 Massachusetts Avenue, Cambridge, MA, USA
- Map (opens in new window)
- Events (opens in new window)
- People (opens in new window)
- Careers (opens in new window)
- Accessibility
- Social Media Hub
- MIT on Facebook
- MIT on YouTube
- MIT on Instagram
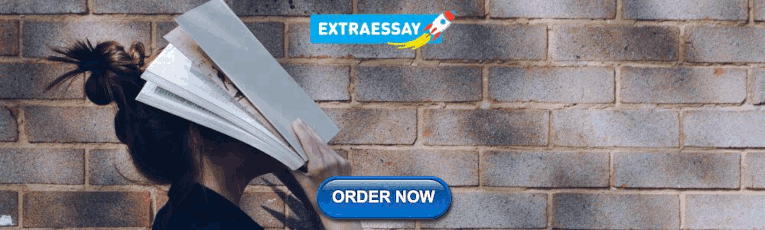
IMAGES
COMMENTS
The fuel cell (FC) technology became in focus within the hydrogen energy landscape as a cost-effective pathway to utilize hydrogen for power generation. Therefore, FC technologies' research and development (R&D) expanded into many pathways such as cost reduction, efficiency improvement, fixed and mobile applications, lifetime, safety and ...
Fuel Cells: Technologies and Applications The Open Fuel Cells Journal, 2013, Volume 6 13. The ove rall cell efficiency is between 37% and 42%. however, if the heated water/steam generat ed by the ...
Global demand for primary energy rises by 1.3% each year to 2040, with an increasing demand for energy services as a consequence of the global economic growth, the increase in the population, and advances in technology. In this sense, fossil fuels (oil, natural gas, and coal) have been widely used for energy production and are projected to remain the dominant energy source until at least 2050 ...
A fuel cell is a device that generates electric energy through electrochemical reactions between an oxidizing agent and a fuel - a material that stores energy in chemical form. ... Research now ...
In recent years, proton exchange membrane (PEM) fuel cells have regained worldwide attention from academia, industries, investors, and governments. The prospect of PEM fuel cells has turned into reality, with fuel cell vehicles successfully launched in the market. However, today's fuel cells remain less competitive than combustion engines and batteries, primarily due to their high cost and ...
Fuel cell electric vehicles: technology. Hydrogen-powered fuel cell vehicles, also called fuel cell electric vehicles, are electric vehicles that depend on an electrochemical system to convert hydrogen to electricity (Sorlei et al. 2021). FCEVs are equipped with a hydrogen storage tank compressed at 700 bars instead of storage batteries for the ...
Future research will dwell on hybrid systems that could harness the advantages of both the hydrogen fuel cell technology and one or combined conventional renewable energy sources. A vehicle with 61a solar rooftop, 61a hydrogen storage-based battery, and 61a stack of fuel cells to power electricity-driven motors is 61a classic example of 61a ...
The purpose of this research was to derive promising technologies for the transport of hydrogen fuel cells, thereby supporting the development of research and development policy and presenting directions for investment. We also provide researchers with information about technology that will lead the technology field in the future. Hydrogen energy, as the core of carbon neutral and green energy ...
MIT - Centre for Advanced Materials Research and Technology, Department of Electronics and Telecommunication Engineering, Maharashtra Institute of Technology, Aurangabad, 431010 India. Search for more papers by this author
Overall, the H 2 (and H 2-dense fuel) IC engines will provide a reliable, durable and cost-efficient solution based on a well-known existing technology, contributing to a fast transition towards carbon-free mobility. The low total cost of ownership, especially in the field of heavy-duty applications, as well as its negligible dependence on low ...
Working from a different perspective, for Mojaver and colleagues, the energy efficiency of an SOFC-O 2 -based system, is higher, which is 60.20% compared to SOFC-H + with 54.06%. The sum of the unit cost of the product (SUPC) of SOFC-O 2− is lower (48.24 $/GJ compared to 48.75 $/GJ) rather than SOFC-H +.
Hydrogen is emerging as a new energy vector outside of its traditional role and gaining more recognition internationally as a viable fuel route. This review paper offers a crisp analysis of the ...
In this study, a recent development in hydrogen fuel cell engines is reviewed to scrutinize the feasibility of using hydrogen as a major fuel in transportation systems. A fuel cell is an ...
Abstract: Hydrogen Proton Exchange Membrane (PEM) fuel cell is considered today as a promising technology for low-carbon transport applications. The successful deployment of fuel cell technology in automotive sector is a key element to reach out the European Union's CO 2 emission goal by 2050. After years of developments by research institutions and enterprises, the automotive fuel cell ...
A query submitted for the term 'microbial fuel cells' on the Web of Science™ platform of Clarivate Analytics (Fig. (Fig.3) 3) showed a gradual increase in the number of research articles on MFCs that were published in the years 2004-2020 in scientific, peer-reviewed journals.It must be noted that this figure serves to only emphasize the growth trend and that the output of a similar ...
N2 - The U.S. Department of Energy's Vehicle Technologies and Hydrogen and Fuel Cell Technologies Offices (VTO and HFTO) support research and development of efficient and sustainable transportation technologies that will improve energy efficiency, minimize emissions, and enable America to use less petroleum.
NREL's research accelerates development, integration, and scale up of hydrogen and fuel cell technologies to enable widespread deployment across multiple energy sectors. Our work helps industry overcome technical challenges and supports the U.S. Department of Energy's (DOE's) Hydrogen and Fuel Cell Technologies Office and H2@Scale vision for ...
In an aim to scale up the microbial fuel cell (MFC), this research is a first attempt to investigate the impact of two lattice structures flow straighteners: gyroid and X lattice to compare with the conventional honeycomb flow straightener, fabricated via additive manufacturing. The study focused on assessing their effect on the degradation of domestic wastewater and power generation in a dual ...
Fuel cell technology development has gradually become a research hotspot in the global energy field. A large number of investigations have been carried out on the preparation technology and basic theory of key materials, components and system such as high activity and anti-aggregation electrocatalyst, high specific surface area and anti-oxidation support, high conductivity and self-humidifying ...
The development of hybrid-electric propulsion technology for transport aircraft presents opportunities for new designs that can reduce fuel consumption and greenhouse gas emissions from commercial aviation and improve on safety and reliability when compared to modern aircraft. The SUbsonic Single Aft eNgine (SUSAN) Electrofan is a conceptual design for a transport aircraft with a series ...
DGIST Professor In Su-il's research team has developed a high-efficiency photocatalyst that utilizes sunlight to convert carbon dioxide (CO2), the primary cause of global warming, into methane ...
In recent years, significant progress in the development of renewable energy sources has increased, presenting viable alternatives to fossilbased energy. These include bioenergy (biofuels ...
Call for Papers Smart Grid Electricity Parameter Sensing Measurement and Self-supply Technology. Submission deadline: Monday, 30 September 2024 Expected Publication Month: March 2025 . With the continuous development of power Internet of Things and wireless sensor networks, intelligent sensing devices will be widely used in the power system.
Massachusetts Institute of Technology (MIT) - Department of Political Science, Massachusetts Institute of Technology. Date Written: March 27, 2024. ... MIT Political Science Department Research Paper Series. Subscribe to this free journal for more curated articles on this topic FOLLOWERS. 1,682. PAPERS. 295. This Journal is curated by: ...
Those papers will be shared widely via a publication venue managed and hosted by the MIT Press under the auspices of the MIT Open Publishing Services program. As with the first round of papers, Thomas Tull, a member of the MIT School of Engineering Dean's Advisory Council and a former innovation scholar at the School of Engineering ...
China continues to set the pace for cutting-edge 5G technology standards Groupe Speciale Mobile Association report At the same time, the number of 5G base stations was nearly 3.38 million - a ...